ABSTRACT
Major advances in pathogen identification, treatment, vaccine development, and avian immunology have enabled the enormous expansion in global poultry production over the last 50 years. Looking forward, climate change, reduced feed, reduced water access, new avian pathogens and restrictions on the use of antimicrobials threaten to hamper further gains in poultry productivity and health. The development of novel in vitro cell culture systems, coupled with new genetic tools to investigate gene function, will aid in developing novel interventions for existing and newly emerging poultry pathogens. Our growing capacity to cryopreserve and generate genome-edited chicken lines will also be useful for developing improved chicken breeds for poultry farmers and conserving chicken genetic resources.
Introduction
Of the three largest animal protein sources, poultry, beef, and pork, poultry is the most affordable, has the shortest production cycle, and has the least environmental impact. Ninety percent of the world's poultry meat production comes from chicken which provides both meat and eggs for consumers of all socioeconomic strata (FAO, Citation2021). In 2020, global poultry production reached over 70 billion chickens, producing 1.6 trillion eggs and 133.3 million tonnes of poultry meat (FAO, Citation2022). To ensure flocks are protected against disease, proper rearing of healthy chickens requires a stringent prescription of vaccinations, high flock biosecurity, and the precise application of anti-bacterials or anti-protozoics. However, in commercial farming systems, increasing flock density and increased global climatic impacts are expected to create future stresses for poultry production leading to increased incidences of infections (Mottet & Tempio, Citation2017). Additionally, in many parts of the world, poultry are raised in open production systems or in small village farms where they have a higher exposure to pathogens, reduced biosecurity, reduced access to pharmaceuticals, and a lower number of vaccinations due to reduced access to vaccines (Cristalli & Capua, Citation2007).
Advances in genetic modification technologies and next-generation sequencing serve as new tools to identify genes activated in both hosts and pathogens during infection, and to modify both genomes to study the role of candidate host genes during infection (Long et al., Citation2019). The results can then be utilized for vaccine development and to identify beneficial alleles for selectively breeding in the chicken population.
Many “transgenic” technologies, such as viral vectors, transposons, and site-directed nucleases, have been developed over the past 30 years to generate genetically modified chicken lines. By lines, we mean chicken containing an introduced DNA construct that will be heritably transferred from parent to offspring.
The first transgenic chicken was produced in 1987 by inserting foreign retroviral DNA, utilizing the avian leukosis virus (ALV) vector, into the yolk sac of the developing embryo, but with very low efficiency (Salter et al., Citation1986). The development of replication-defective lentiviruses almost 20 years later helped improve germline transmission and served as a more stable system for carrying transgenic cargos to generate transgenic chicken (McGrew et al., Citation2004). There are still, however, many drawbacks to using viral vectors, including the formation of replication-deficient viral particles and the cargo size of the transgene is restricted.
The delivery of transgenes to both embryos and cultured PGCs was then improved with the use of transposons. Transposons are DNA sequences capable of “jumping” from one location, such as a plasmid, to another, such as the genome of a cell, in the presence of the transposase enzyme (Ivics et al., Citation2009). Unlike viral vectors, transposons, such as piggyBac and Tol2, have been demonstrated to efficiently integrate into the genomes of chicken embryos and cultured PGCs without silencing, as has been reported with viral vectors (Sato et al., Citation2007; Macdonald et al., Citation2012). Like viral vectors, however, they cannot be directed to specific locations in the genome (Glover et al., Citation2013).
The next major development in the generation of transgenic chickens came with the establishment of culture conditions for primordial germ cells (PGCs) (van de Lavoir et al., Citation2006; Whyte et al., Citation2015). PGCs are the embryonic precursors of sperm and egg. They arise from the epiblast and eventually migrate through the blood vessels to reach the gonads (Eyal-Giladi & Kochav, Citation1976). PGCs can be isolated and cultured during their migration through the blood or from the gonads (Hu et al., Citation2022). Once in culture, PGCs can be genetically modified and then reintroduced into host embryos whose offspring would contain the desired modification, such as gene knockouts. The culture of PGCs allowed transposons to be used to introduce transgenic constructs into the chicken (Macdonald et al., Citation2012; Park & Han, Citation2012) (A). More importantly, cultured PGCs generated the first knockout chickens using standard genetic modification technologies first developed for mouse embryonic stem (ES) cells (Schusser et al., Citation2013).
Figure 1. Creating genetically modified chickens. A) Transposon plasmids or genome editing tools are directly injected into the laid egg or the circulatory system of day 2.5 embryos. The embryos are hatched, raised to sexual maturity, and bred. Breeding these F0 chickens will produce F1 offspring containing the transposon or genetic modification. B) PGCs are cultivated from blood samples from day 2.5 chicken embryos and subsequently transformed with a transposon vector or genome editing tools. These transfected cultures are sorted and grown clonally to produce transgenic PGC cultures containing the desired modification. The genetically modified PGCs are then injected into day 2.5 host embryos and then hatched to create mature F0 chickens with some modified germ cells. Crossing these chickens with wildtype chickens produces several F1 offspring that will be derived from the donor germ cells.
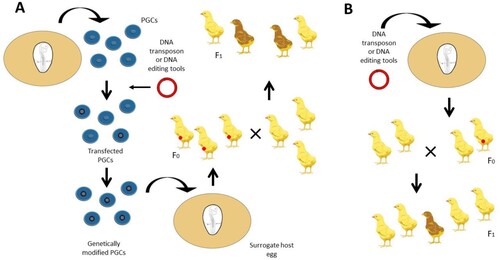
More efficient site-specific genome modifications were made possible with the development of site-directed nucleases such as zinc finger nucleases (ZFNs), transcription activator-like effector nucleases (TALENs), and CRISPR/Cas9 which have been revolutionary to the field. Unlike previous tools, site-directed nucleases can be programmed to initiate double-strand breaks (DSB) at precise locations in the genome (Kim et al., Citation1996; Li et al., Citation2011; Cong et al., Citation2013). Once a DSB has been created, the DNA is repaired by either the nonhomologous end joining (NHEJ) or by the homology-directed repair (HDR) pathways (Chojnacka-Puchta & Sawicka, Citation2020).
The NHEJ pathway is the primary pathway used for repairing DSBs and can occur at any point during the cell cycle. In this pathway, proteins involved in the NHEJ process generate regions of small microhomology between two otherwise incompatible DNA ends to facilitate end joining. This allows NHEJ to work with a wide range of DNA-end configurations but typically results in the formation of insertion/deletion (INDEL) mutations in the repaired DNA junction (Chang et al., Citation2017). This process can be exploited to generate null mutation alleles to investigate gene function. Meanwhile, the HDR pathway is active during the S and G2 phases of the cell cycle and requires a repair template strand, with homologous regions surrounding the loose ends, to accurately repair the DSB (Rothkamm et al., Citation2003). The HDR pathway can thus be utilized to introduce desired sequence changes in the target genome.
Among the site-directed nucleases, the CRISPR-Cas editing system has become the most widely adopted, as both ZFNs and TALENs are expensive to synthesize and difficult to construct compared to the CRISPR/Cas9 system. The CRISPR-Cas editing system, developed from a bacterial antiviral pathway, utilizes short RNA sequences to guide and bind a Cas endonuclease protein to the complementary regions of the target loci, where it induces DSBs (Cong et al., Citation2013). However, it is important to note that the Cas9 protein may cleave nonspecifically or at unintended locations at sites in the genome, known as off-targets, resulting in unwanted mutations. However, the number of off targets may be reduced by using off-target prediction tools or by using Cas9 variants such as the high fidelity Cas9 variant. Targeting of PGCs using TALENs and CRISPR/Cas9 has facilitated the production of knockout chickens (Woodcock et al., Citation2017).
Excitingly, it was recently demonstrated that transposons and CRISPR/Cas9 vectors could be delivered directly to the early avian embryo, either under the epiblast or into the embryonic vascular system, eliminating the need for the in vitro culture of PGCs (Tyack et al., Citation2013; Lee et al., Citation2019; Challagulla et al., Citation2020; Barzilai-Tutsch et al., Citation2022) (B). With these new genetic and cellular tools, it may soon be possible to investigate gene function in any species of bird.
Cells for in vitro modelling of poultry-pathogen interactions
Studying host–pathogen interactions has revealed fundamental information on how chickens respond to viruses, parasites, and bacterial infections. This has been accomplished by understanding the molecular mechanisms employed by pathogens to proliferate and survive within hosts as well as those involved in the hosts’ defence (Cossart et al., Citation1996; Welch, Citation2015). While in vivo animal infection studies are highly informative, cell lines, primary cells, and pluripotent stem cells as in vitro models have been crucial to study host–pathogen interactions in isolation () (Zuo et al., Citation2016; Zhang et al., Citation2019). Working with cell lines has several advantages, such as their affordability, ease of use, and ability to be continually passaged as they are immortalized (Pellegrino & Gutierrez, Citation2021). Initially, cell lines were established from cells which underwent spontaneous immortalization. However, methods were later developed to immortalize cells deliberately. Cell lines can be immortalized by introducing oncogenes, which encode oncoproteins, into a cell utilizing viral vectors. These oncogenes bypass or inactivate tumour suppressants p53, Rb, or p16 which are all critical regulators of the cell cycle (Irfan Maqsood et al., Citation2013). Normally, once DNA damage is detected in a cell, the transcription factor p53 is activated, causing the cell to enter cell cycle arrest till the DNA damage can be corrected. In cases where the damage is severe, p53 causes cell cycle arrest and then subsequently induces apoptosis (Shay et al., Citation1991; Chen, Citation2016). Meanwhile, expression of Rb and p16 are critical to prevent DNA replication in cells containing damaged DNA resulting in cell senescence (Takahashi et al., Citation2007). Alterations to these critical cell cycle regulators by oncoproteins thus allows cells to continue dividing without control (Pereira-Smith & Smith, Citation1988). Activation or insertion of the c-Myc gene can also result in cell immortality as was the case in the avian DT-40 B cell line (Hayward et al., Citation1981; De Filippis et al., Citation2007). Cell lines can also be immortalized by ectopic expression of telomerase or telomerase reverse transcriptase (TERT) as was achieved in the ICP1 and ICP2 chicken preadipocyte lines (Wang et al., Citation2017). Telomerase and TERT both act to stabilize and elongate telomeres, highly repetitive nucleotide sequences located at the ends of chromosomes. In a normal somatic cell, each time a cell replicates its DNA, the telomeres gradually shorten, eventually exposing the chromosome ends, ultimately resulting in cell senescence. However, the ectopic expression of telomerase and TERT leads to telomere elongation, increasing the cell's chromosomal stability and allowing the cell to bypass cell senescence to become immortalized (Morales et al., Citation1999).
Figure 2. In vitro cell systems to study avian pathogens. Cell lines, primary cells, and pluripotent stem cells are all useful for studying host-pathogen interaction in avian species. Each system has its advantages and disadvantages.
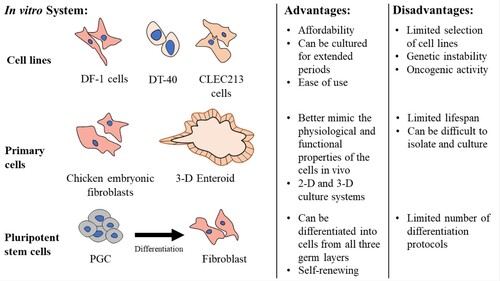
In poultry, the lung epithelial CLEC213 cell line, B cell line DT-40, and the DF-1 fibroblast cell line have been especially useful for modelling diseases in vitro as they are amenable to transfection and infection with several poultry pathogens (Winding & Berchtold, Citation2001; Esnault et al., Citation2011; Koslová et al., Citation2018). DT-40 cells were critically used to study B cell biology and led to the discovery that activation-induced cytidine deaminase is required to initiate immunoglobulin gene diversification via gene conversion (Buerstedde et al., Citation1990; Kim et al., Citation1990). In 2019, Cheng et al. (Citation2019) utilized the CRISPR/Cas9 system in DF-1 cells to knock out chicken TANK-binding kinase 1 (TBK1) to investigate its role in the production of chicken stimulator of interferon gene (chSTING) mediated interferon-beta (IFNβ) response in chickens. Their work led to the finding that chTBK1 is essential for regulating chSTING-mediated IFN regulation (Cheng et al., Citation2019).
Cell lines and CRISPR/Cas9 have also facilitated the production of disease-resistant cells and chicken. Koslová et al. (Citation2018) utilized CRISPR/Cas9 in DF-1 cells to generate frame-shifting INDEL mutations into the tva, tvc, and chNHE1 genes to confer the cells with resistance to ALV subgroups A, C, and J infection. This work, and subsequent work in chicken embryonic fibroblasts, played a critical role in the development of ALV-J resistant chickens (Hellmich et al., Citation2020; Koslová et al., Citation2020). Cell lines, however, do have their limitations. In avian species, most cell types do not have established cell lines. This is because the methods utilized to immortalize cell lines do not reliably produce immortalized cell lines, and a cell line must be made for each tissue of interest (Soice & Johnston, Citation2021). Furthermore, using viral vectors to immortalize cells with oncogenes tends to increase genetic instability and oncogenic activity resulting in unregulated growth and metabolism (Frattini et al., Citation2015; Liu et al., Citation2019). In contrast to cell lines, primary cells are directly derived from tissues and tend to preserve better the functional and morphological properties of the tissues from which they originated (Kartsogiannis & Ng, Citation2004). Traditionally, primary cells are grown using a 2-dimensional (2-D) culture system. However, in recent years, there has been a growing emphasis on developing more sophisticated, physiologically appropriate in vitro systems that more closely represent their in vivo environment. This has given rise to the development of organoids, 3-dimensional (3-D), self-organizing, multicellular aggregates that mimic the cellular interactions and structural characteristics of the tissues from which they originate (Kim et al., Citation2020).
In vitro disease modelling of intestinal pathogens has traditionally been done using 2-D intestinal epithelial primary cultures (Rath et al., Citation2018). However, recent work by Nash et al. (Citation2021) led to the development of inside-out suspension chicken enteroids, organoids used to model the intestine. Remarkably, their system consists of several cell types, including interepithelial leukocytes and lamina propria leukocytes, in addition to fibroblasts and intestinal epithelial cells found in 2-D cultures. These 3-D enteroids develop with the apical brush border in direct contact with the medium allowing enteroids to be easily infected with pathogens such as Eimeria tenella, influenza A virus, and Salmonella typhimurium without using microinjections (Nash et al., Citation2021). Thus, this system is useful for investigating multicellular interactions and cellular responses to infection. However, isolating primary cells for 2-D and 3-D cultures can be tedious, and many cell types do not grow well when cultured. Furthermore, most primary cells have a limited lifespan and must be continuously harvested (Pellegrino & Gutierrez, Citation2021). Pluripotent stem cell types (PSCs), such as embryonic stem cells, embryonic germ cells, i.e. PGCs, and induced pluripotent stem cells, can also serve as an alternative source of somatic cells to primary cells and cell lines (Young et al., Citation2016; Zhang et al., Citation2017). PSCs are cells capable of self-renewing and differentiating into all three primary germ layers of an early embryo. They cannot, however, form the extraembryonic tissues (Romito & Cobellis, Citation2015). In mammalian species, various protocols have been established to differentiate PSCs towards many defined cell lineages, including immune cells and enteroids, and have proven to be an effective tool for studying development, disease modelling, and the genetic bases of various illnesses (Spence et al., Citation2011; Chal et al., Citation2015; Young et al., Citation2016; Shi et al., Citation2019). To date, several pluripotent stem cell types have been established from avian embryos, including embryonic stem cells, induced pluripotent stem cells and embryonic germ cells (Pain et al., Citation1996; van de Lavoir et al., Citation2006; Lu et al., Citation2012). However, few studies have conducted functional analyses on the somatic cells differentiated from these PSCs. Long et al. (Citation2019) conducted one of the first such studies. Using CRISPR/Cas9, they knocked out acidic nuclear phosphoprotein 32 family member A (ANP32A), a gene critical for avian influenza replication, in chicken PGCs. The ANP32A knock-out PGCs were then subsequently differentiated into fibroblast-like cells, a cell type permissive to avian influenza virus, and shown to support neither avian nor mammalian influenza virus polymerase activity (Long et al., Citation2019). While avian stem cells appear to be a promising tool, avian differentiation protocols for many cell types have yet to be established, and the field is still in its infancy.
As new gene editing tools are developed, all in vitro models will continue to play a critical role for investigating host–pathogen interactions. One especially exciting development will be the adaptation of genome-wide CRISPR-Cas9 knockout screens to avian in vitro models to identify multiple pathways involved during an infection (Shalem et al., Citation2014).
Genome editing to investigate immune gene function
A major development in the generation of transgenic chickens to study host–pathogen interactions came with the establishment of culture conditions for PGCs (van de Lavoir et al., Citation2006; Whyte et al., Citation2015) which are the embryonic precursors of sperm and egg. As introduced above, PGCs arise from the epiblast and migrate through the blood vessels to reach the gonads (Eyal-Giladi & Kochav, Citation1976). PGCs can be isolated and cultured during their migratory period or from the gonads of stage 20–24 Hamburger and Hamilton embryos (Hu et al., Citation2022). Once in culture, PGCs can be modified and then reintroduced into host embryos whose offspring could be used to produce genetically modified offspring within one to two generations (Schusser et al., Citation2013). While originally these modifications were introduced in vivo using viral vectors in developing embryos, improvements in PGC culture medium has facilitated the genetic manipulation of PGCs in vitro to generate transgenic chickens () (Whyte et al., Citation2015; Lee et al., Citation2016). This, in turn, allows better in vitro assessment of genome editing and pathogen resistance prior to in vivo testing. However, this is still more commonly carried out using embryonic fibroblast lines rather than PGCs (Koslová et al., Citation2020; Li et al., Citation2020; Challagulla, Jenkins et al., Citation2021; Challagulla, Schat et al., Citation2021). Genome editing technologies have been adapted for a wide range of different functions, such as gene editing using Cas9 being used to produce knockouts of immunity genes to investigate gene function in lymphocytes (Seki & Rutz, Citation2018; Akidil et al., Citation2021). For example, the knockout of immunoglobulin subunits in chicken PGCs via homologous recombination was used to assess the role of these subunits in B-cell development and subsequently as the basis for a knockout chicken model to study the effects of B-cell depletion on the immune response to Marek's disease virus (MDV) (Schusser et al., Citation2013, Citation2016; Bertzbach et al., Citation2018). Similarly, Lee et al. (Citation2022) produced an immunodeficient chicken model lacking B and T cells via CRISPR-mediated knockdown of the RAG1 gene (Lee et al., Citation2022). Other gene knockouts have also been used to produce pathogen resistant and susceptible animals, with applications for studying host–pathogen interactions and improving food security in livestock species such as poultry.
While Cas9 remains widely used as the first CRISPR-Cas system adapted for eukaryotic targeting, a variety of other CRISPR effector proteins with a diverse range of features have been identified over the years (Cong et al., Citation2013; Makarova et al., Citation2015; Abudayyeh et al., Citation2016). These include the Cas12 effectors with both DNA and RNA targeting activity or the exclusively RNA targeting Cas13 effector proteins (Zetsche et al., Citation2015; Abudayyeh et al., Citation2017). A similarly wide variety of systems have been utilized for delivery and expression of the CRISPR system in avian models, including transposon vectors, plasmid vectors or modified viral vectors such as adenovirus or MDV (Abu-Bonsrah et al., Citation2016; Lee et al., Citation2019; Liu et al., Citation2020). To generate CRISPR-based homozygous edited in vivo knockout models, these systems are most commonly used to create transgenic PGC cultures, which are then reinserted into host embryos as described above and crossbred to produce homozygous transgenic offspring ().
Genome editing to investigate pathogen resistance in chicken
To date, one of the most common methods of successfully studying pathogen resistance in chicken cells has been to knockout or knockdown expression of host viral cofactors or receptors (Kim & Zhou, Citation2015; Koslová et al., Citation2018; Cheng et al., Citation2019; Long et al., Citation2019). This technique has produced several promising examples of viral inhibition, such as the inhibition of avian influenza virus (AIV) and ALV replication mentioned above (Koslová et al., Citation2018; Long et al., Citation2019; Hellmich et al., Citation2020). In both cases, specific exons or amino acid residues, which previous studies had associated with resistance or susceptibility to viral infection, were edited to inhibit viral infection. The resulting edited cell lines in both cases showed no significant change in normal phenotype aside from the observed viral resistance. Similarly, some studies have inhibited viral replication via overexpression of host proteins known to be downregulated during infection. For example, Duan et al. (Citation2020) significantly reduced replication of then Newcastle disease virus (NDV) in DF-1 cultures via plasmid-based overexpression of the transcription factor bromodomain-containing protein 2 (BRD2) (Duan et al., Citation2020). The PGC transfection utilized by Long et al. (Citation2019) is a common strategy for generating pathogen-resistant chicken lines, given that it allows stable introduction of germline modifications to produce fully transgenic offspring within one to two generations.
In addition to direct CRISPR-based genome editing, new tools for epigenetic regulation have recently been developed using catalytically dead CRISPR effectors, such as the CRISPRoff system of a dCas9 effector fused to domains from the epigenetic silencing factors Dnmt3A, Dnmt3L and KRAB (Nuñez et al., Citation2021). Transient expression of the CRISPRoff protein was shown to induce at least 40% epigenetic silencing of GFP-tagged transgenes for over 50 days in mammalian cells, consistent with previous epigenome engineering studies utilizing similar dCas9 fusions (O’Geen et al., Citation2019; Nuñez et al., Citation2021). Similarly, reactivation of epigenetically silenced genes was performed in the same study via the CRISPRon system, composed of dCas9 fused to a sgRNA containing MS2 binding sites for transcriptional activator proteins (Nuñez et al., Citation2021). Given that little to no off-target activity was observed and that similar dCas9-based epigenetic editing tools have been designed and tested in chicken models, this offers the possibility of a highly specific, reversible system to identify and introduce pathogen resistant epigenetic variants into avian models (Williams et al., Citation2018).
Using shRNAs, Cas9 and Cas13 to directly target pathogens
In addition to host immune factor knockdown, a number of papers have assessed options for inhibiting viral replication via direct targeting and interference of the viral genome (Zhang et al., Citation2019). Some have aimed to target the genome with short hairpin RNA (shRNA) sequences to produce RNA interference, such as targeting the NP gene of the NDV genome (Yue et al., Citation2008). Similarly, direct shRNA targeting of DNA binding proteins was moderately successful at inhibiting replication of MDV in chicken embryonic fibroblasts (CEFs), with up to a 10-fold reduction in viral titre recorded compared to non-specific sequences (Lambeth et al., Citation2009). However, while relatively effective, other studies have recorded an increase in morbidity and lethality associated with long-term transgenic shRNA expression in mammalian models, possibly due to the inhibition of endogenous miRNA pathways (Grimm et al., Citation2006; Dai et al., Citation2014). As such, more recent research has attempted a variety of different approaches to reduce the toxicity of shRNA-based targeting, such as using less potent or more tissue-specific promoters to limit shRNA expression and reduce toxicity (An et al., Citation2006; Giering et al., Citation2008). Notably, Challagulla et al. (Citation2022) developed promoter-free expression of shRNAs using parallel processing adjacent to an intronic miRNA site, producing significantly reduced expression of exogenous target sites, including in the AIV PB1 subunit, without observable toxicity or cell death in chicken PGCs. Though the knockdowns observed were relatively small, with an average reduction of around 20–25% per construct, this suggests that miRNA-linked shRNA expression could be further developed for RNAi-based viral targeting applications in vivo, such as by linking shRNA expression to expression of innate immune response genes both in vitro and in vivo (Challagulla et al., Citation2022).
As a more specific alternative to RNAi-based knockdown, several studies have developed CRISPR/Cas-based systems for direct viral genome targeting in avian cells (Li et al., Citation2020; Liu et al., Citation2020; Challagulla, Jenkins et al., Citation2021). To ensure greater antiviral efficacy, these systems commonly target multiple loci within genes key to viral replication, as these genes are more functionally conserved and thus less likely to develop escape mutations which could inhibit effective viral targeting. To date, a number of studies have successfully inhibited viral replication in avian models via CRISPR targeting – for example, replication of MDV in transgenic chicken embryonic cells was significantly reduced by gRNA targeting of the ICP4 viral polypeptide gene (Challagulla, Jenkins et al., Citation2021). Some systems have even demonstrated significant impacts on viral replication in vivo. For example, one study recorded significantly reduced viral shedding from reticuloendotheliosis virus (REV)-infected chickens using a Cas9-based system directly targeting the REV genome (Li et al., Citation2020).
Similar transgenic systems are also being developed and tested to target RNA viruses, utilizing the Cas13 family of RNA-specific CRISPR effectors. Cas13 has shown some efficacy in viral targeting, with Cas13a-based targeting of influenza A virus (IAV) genes producing a 2- to-4-fold reduction in viral replication in embryonic fibroblasts (Challagulla, Schat et al., Citation2021). As such, these systems are a viable alternative to host factor targeting for generating resistant chickens, particularly to mediate possible side-effects from altering endogenous gene expression. However, other studies have noted the variation in Cas13 off-target effects across other model organisms (Ai et al., Citation2022), indicating that these systems would likely require further optimization before they can be applied to generate commercial disease-resistant lines.
Development of sterile host embryos
Once injected into a host chick embryo, PGCs colonize the gonads of the host embryo alongside the endogenous PGCs. This reduces the likelihood that the offspring produced from subsequent mating will be descended from the donor PGCs (Nakamura et al., Citation2010; Trefil et al., Citation2017). Therefore, it is beneficial to reduce or irradicate endogenous PGCs. Several chemical and physical methods have been utilized for this purpose, including UV radiation, γ irradiation and an injection of the cross-linking reagent, busulfan. While all these methods reduce the number of endogenous PGCs, they do not reliably eliminate all germ cells, and these agents are very toxic to the host embryo (Minematsu et al., Citation2004; Park et al., Citation2010). Recently, however, Ballantyne et al. (Citation2021) developed a surrogate host chicken line that allows for conditional ablation of both the male and female germline. Utilizing CRISPR/Cas9-mediated homology-directed repair, the chicken contains an inducible caspase-9 protein targeted to the DAZL gene locus. The DAZL gene is expressed exclusively in the germ cell lineage. In the presence of a chemical compound, the caspase 9 protein is activated, inducing apoptosis and selectively killing the host's endogenous germ cells. The introduced donor PGCs can then efficiently colonize the host's gonads (Ballantyne et al., Citation2021).
Direct mating of sire and dam surrogates (SDS) can therefore be used to create pure-breed homozygous edited offspring (). The development of this system significantly reduces the generation time of genome-edited birds and significantly increases the number of homozygous genome-edited offspring. In addition, SDS mating can be utilized for the conservation of chicken breeds by bypassing the challenges of cryopreserving avian sperm and eggs. Instead, avian species can be brought back from cryopreserved PGCs or embryonic gonads (Ballantyne et al., Citation2021) ().
Figure 3. Generating homozygous genome-edited or rare breed offspring using sterile surrogate hosts. Embryonic gonads, cultured PGCs, or gene-edited PGCs can be cryopreserved before injection into sterile surrogate host embryos. The surrogate hosts are hatched and raised to sexual maturity. When mated, the offspring are entirely derived from the donor genetic material.
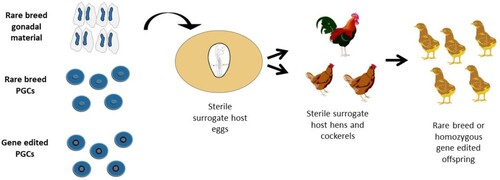
Discussion
Over the last two decades, advances in genetic engineering tools, especially the CRISPR/Cas9 system, have facilitated the generation of gene-edited in vitro and in vivo models to study host–pathogen interactions in chicken. Together with improvements made in generating transgenic chicken models, namely via adenovirus delivery of CRISPR/Cas9 vectors in vitro PGC transfection, these systems should provide a diverse array of models for studying host–pathogen interactions in chickens and poultry species (Tyack et al., Citation2013; Lee et al., Citation2019).
In addition to targeting the genomes of both hosts and pathogens in such studies, the discovery of CRISPR/Cas effectors, such as Cas12 or Cas13, now opens the possibility of modulating viral and host transcriptomes to study host–pathogen interactions. Such effectors may also be effective in engineering CRISPR-based pathogen resistance in chickens, through both host factor knockdown and direct targeting of viral genomes (Zhang et al., Citation2019).
Continuing developments in PGC transfection and reintroduction into host embryos also offer a new, more viable option for biobanking and preservation of chicken genetics. Though still being assessed for efficacy, this method could have direct applications for future host–pathogen interactions, particularly the effect that existing genetic variation and environmental adaptations have on pathogen resistance, and could therefore be highly useful in maintaining and improving agricultural biosecurity in the poultry industry.
Acknowledgements
Euan Mitchell is funded by a BBSRC EastBio fellowship. Guillermo Tellez is funded by the University of Edinburgh Principal’s Career Development PhD Scholarship and PhD funding from Cobb-Vantress, Inc.
Disclosure statement
No potential conflict of interest was reported by the authors.
Additional information
Funding
References
- Abu-Bonsrah, K.D., Zhang, D. & Newgreen, D.F. (2016). CRISPR/Cas9 targets chicken embryonic somatic cells in vitro and in vivo and generates phenotypic abnormalities. Scientific Reports, 6, 34524.
- Abudayyeh, O.O., Gootenberg, J.S., Essletzbichler, P., Han, S., Joung, J., Belanto, J.J., Verdine, V., Cox, D.B.T., Kellner, M.J., Regev, A., Lander, E.S., Voytas, D.F., Ting, A.Y. & Zhang, F. (2017). RNA targeting with CRISPR–Cas13. Nature, 550, 280–284.
- Abudayyeh, O.O., Gootenberg, J.S., Konermann, S., Joung, J., Slaymaker, I.M., Cox, D.B.T., Shmakov, S., Makarova, K.S., Semenova, E., Minakhin, L., Severinov, K., Regev, A., Lander, E.S., Koonin, E.V. & Zhang, F. (2016). C2c2 is a single-component programmable RNA-guided RNA-targeting CRISPR effector. Science, 353, aaf5573.
- Ai, Y., Liang, D. & Wilusz, J.E. (2022). CRISPR/Cas13 effectors have differing extents of off-target effects that limit their utility in eukaryotic cells. Nucleic Acids Research, 50, e65.
- Akidil, E., Albanese, M., Buschle, A., Ruhle, A., Pich, D., Keppler, O.T. & Hammerschmidt, W. (2021). Highly efficient CRISPR-Cas9-mediated gene knockout in primary human B cells for functional genetic studies of Epstein-Barr virus infection. PLoS Pathogens, 17, e1009117.
- An, D.S., Qin, F.X.-F., Auyeung, V.C., Mao, S.H., Kung, S.K.P., Baltimore, D. & Chen, I.S.Y. (2006). Optimization and functional effects of stable short hairpin RNA expression in primary human lymphocytes via lentiviral vectors. Molecular Therapy, 14, 494–504.
- Ballantyne, M., Woodcock, M., Doddamani, D., Hu, T., Taylor, L., Hawken, R.J. & McGrew, M.J. (2021). Direct allele introgression into pure chicken breeds using sire dam Surrogate (SDS) mating. Nature Communications, 12, 659.
- Barzilai-Tutsch, H., Morin, V., Toulouse, G., Chernyavskiy, O., Firth, S., Marcelle, C. & Serralbo, O. (2022). Transgenic quails reveal dynamic TCF/β-catenin signaling during avian embryonic development. ELife, 11, e72098.
- Bertzbach, L.D., Laparidou, M., Härtle, S., Etches, R.J., Kaspers, B., Schusser, B. & Kaufer, B.B. (2018). Unraveling the role of B cells in the pathogenesis of an oncogenic avian herpesvirus. Proceedings of the National Academy of Sciences, 115, 11603–11607.
- Buerstedde, J.M., Reynaud, C.A., Humphries, E.H., Olson, W., Ewert, D.L. & Weill, J.C. (1990). Light chain gene conversion continues at high rate in an ALV-induced cell line. The EMBO Journal, 9, 921–927.
- Chal, J., Oginuma, M., Al Tanoury, Z., Gobert, B., Sumara, O., Hick, A., Bousson, F., Zidouni, Y., Mursch, C., Moncuquet, P. & Tassy, O. (2015). Differentiation of pluripotent stem cells to muscle fiber to model Duchenne muscular dystrophy. Nature Biotechnology, 33, 962–969.
- Challagulla, A., Jenkins, K.A., O’Neil, T.E., Morris, K.R., Wise, T.G., Tizard, M.L., Bean, A.G.D., Schat, K.A. & Doran, T.J. (2020). Germline engineering of the chicken genome using CRISPR/Cas9 by in vivo transfection of PGCs. Animal Biotechnology, 0, 1–10.
- Challagulla, A., Jenkins, K.A., O’Neil, T.E., Shi, S., Morris, K.R., Wise, T.G., Paradkar, P.N., Tizard, M.L., Doran, T.J. & Schat, K.A. (2021). In vivo inhibition of Marek’s disease virus in transgenic chickens expressing Cas9 and gRNA against ICP4. Microorganisms, 9, 164.
- Challagulla, A., Schat, K.A. & Doran, T.J. (2021). In vitro inhibition of influenza virus using CRISPR/Cas13a in chicken cells. Methods and Protocols, 4, 40.
- Challagulla, A., Tizard, M.L., Doran, T.J., Cahill, D.M. & Jenkins, K.A. (2022). Harnessing intronic microRNA structures to improve tolerance and expression of shRNAs in animal cells. Methods and Protocols, 5, 18.
- Chang, H.H.Y., Pannunzio, N.R., Adachi, N. & Lieber, M.R. (2017). Non-homologous DNA end joining and alternative pathways to double-strand break repair. Nature Reviews Molecular Cell Biology, 18, 495–506.
- Chen, J. (2016). The cell-cycle arrest and apoptotic functions of p53 in tumor initiation and progression. Cold Spring Harbor Perspectives in Medicine, 6, a026104.
- Cheng, Y., Lun, M., Liu, Y., Wang, H., Yan, Y. & Sun, J. (2019). CRISPR/Cas9-mediated chicken TBK1 gene knockout and its essential role in STING-mediated IFN-β induction in chicken cells. Frontiers in Immunology, 9.
- Chojnacka-Puchta, L. & Sawicka, D. (2020). CRISPR/Cas9 gene editing in a chicken model: current approaches and applications. Journal of Applied Genetics, 61, 221–229.
- Cong, L., Ran, F.A., Cox, D., Lin, S., Barretto, R., Habib, N., Hsu, P.D., Wu, X., Jiang, W., Marraffini, L.A. & Zhang, F. (2013). Multiplex genome engineering using CRISPR/Cas systems. Science, 339, 819–823.
- Cossart, P., Boquet, P., Normark, S. & Rappuoli, R. (1996). Cellular microbiology emerging. Science, 271, 315–316.
- Cristalli, A. & Capua, I. (2007). Practical problems in controlling H5N1 high pathogenicity avian influenza at village level in Vietnam and introduction of biosecurity measures. Avian Diseases, 51, 461–462.
- Dai, R., Rossello, R., Chen, C., Kessler, J., Davison, I., Hochgeschwender, U. & Jarvis, E.D. (2014). Maintenance and neuronal differentiation of chicken induced pluripotent stem-like cells. Stem Cells International, 2014, e182737.
- De Filippis, L., Lamorte, G., Snyder, E.Y., Malgaroli, A. & Vescovi, A.L. (2007). A novel, immortal, and multipotent human neural stem cell line generating functional neurons and oligodendrocytes. Stem Cells, 25, 2312–2321.
- Duan, Z., Han, Y., Zhou, L., Yuan, C., Wang, Y., Zhao, C., Tang, H. & Chen, J. (2020). Chicken bromodomain-containing protein 2 interacts with the Newcastle disease virus matrix protein and promotes viral replication. Veterinary Research, 51, 120.
- Esnault, E., Bonsergent, C., Larcher, T., Bed’hom, B., Vautherot, J.-F., Delaleu, B., Guigand, L., Soubieux, D., Marc, D. & Quéré, P. (2011). A novel chicken lung epithelial cell line: characterization and response to low pathogenicity avian influenza virus. Virus Research, 159, 32–42.
- Eyal-Giladi, H. & Kochav, S. (1976). From cleavage to primitive streak formation: a complementary normal table and a new look at the first stages of the development of the chick: I. general morphology. Developmental Biology, 49, 321–337.
- FAO. (2021). Meat market review: emerging trends and outlook, December 2021. Rome.
- FAO. (2022, August 22). FAOSTAT Statistical Database.
- Frattini, A., Fabbri, M., Valli, R., De Paoli, E., Montalbano, G., Gribaldo, L., Pasquali, F. & Maserati, E. (2015). High variability of genomic instability and gene expression profiling in different HeLa clones. Scientific Reports, 5, 15377.
- Giering, J.C., Grimm, D., Storm, T.A. & Kay, M.A. (2008). Expression of shRNA from a tissue-specific pol II promoter is an effective and safe RNAi therapeutic. Molecular Therapy, 16, 1630–1636.
- Glover, J.D., Taylor, L., Sherman, A., Zeiger-Poli, C., Sang, H.M. & McGrew, M.J. (2013). A novel piggyBac transposon inducible expression system identifies a role for Akt signalling in primordial germ cell migration. PLoS One, 8, e77222.
- Grimm, D., Streetz, K.L., Jopling, C.L., Storm, T.A., Pandey, K., Davis, C.R., Marion, P., Salazar, F. & Kay, M.A. (2006). Fatality in mice due to oversaturation of cellular microRNA/short hairpin RNA pathways. Nature, 441, 537–541.
- Hayward, W.S., Neel, B.G. & Astrin, S.M. (1981). Activation of a cellular onc gene by promoter insertion in ALV-induced lymphoid leukosis. Nature, 290, 475–480.
- Hellmich, R., Sid, H., Lengyel, K., Flisikowski, K., Schlickenrieder, A., Bartsch, D., Thoma, T., Bertzbach, L.D., Kaufer, B.B., Nair, V., Preisinger, R. & Schusser, B. (2020). Acquiring resistance against a retroviral infection via CRISPR/Cas9 targeted genome editing in a commercial chicken line. Frontiers in Genome Editing, 2.
- Hu, T., Taylor, L., Sherman, A., Keambou Tiambo, C., Kemp, S.J., Whitelaw, B., Hawken, R.J., Djikeng, A. & McGrew, M.J. (2022). A low-tech, cost-effective and efficient method for safeguarding genetic diversity by direct cryopreservation of poultry embryonic reproductive cells. ELife, 11, e74036.
- Irfan Maqsood, M., Matin, M.M., Bahrami, A.R. & Ghasroldasht, M.M. (2013). Immortality of cell lines: challenges and advantages of establishment. Cell Biology International, 37, 1038–1045.
- Ivics, Z., Li, M.A., Mátés, L., Boeke, J.D., Nagy, A., Bradley, A. & Izsvák, Z. (2009). Transposon-mediated genome manipulation in vertebrates. Nature Methods, 6, 415–422.
- Kartsogiannis, V. & Ng, K.W. (2004). Cell lines and primary cell cultures in the study of bone cell biology. Molecular and Cellular Endocrinology, 228, 79–102.
- Kim, Y.G., Cha, J. & Chandrasegaran, S. (1996). Hybrid restriction enzymes: Zinc finger fusions to Fok I cleavage domain. Proceedings of the National Academy of Sciences of the United States of America, 93, 1156–1160.
- Kim, J., Koo, B.-K. & Knoblich, J.A. (2020). Human organoids: model systems for human biology and medicine. Nature Reviews Molecular Cell Biology, 21, 571–584.
- Kim, S., Humphries, E.H., Tjoelker, L., Carlson, L. & Thompson, C.B. (1990). Ongoing diversification of the rearranged immunoglobulin light-chain gene in a bursal lymphoma cell line. Molecular and Cellular Biology, 10, 3224–3231.
- Kim, T.H. & Zhou, H. (2015). Functional analysis of chicken IRF7 in response to dsRNA analog poly(I:C) by integrating overexpression and knockdown. PLoS One, 10, e0133450.
- Koslová, A., Kučerová, D., Reinišová, M., Geryk, J., Trefil, P. & Hejnar, J. (2018). Genetic resistance to avian leukosis viruses induced by CRISPR/Cas9 editing of specific receptor genes in chicken cells. Viruses, 10, 605.
- Koslová, A., Trefil, P., Mucksová, J., Reinišová, M., Plachý, J., Kalina, J., Kučerová, D., Geryk, J., Krchlíková, V., Lejčková, B. & Hejnar, J. (2020). Precise CRISPR/Cas9 editing of the NHE1 gene renders chickens resistant to the J subgroup of avian leukosis virus. Proceedings of the National Academy of Sciences, 117, 2108–2112.
- Lambeth, L.S., Zhao, Y., Smith, L.P., Kgosana, L. & Nair, V. (2009). Targeting Marek’s disease virus by RNA interference delivered from a herpesvirus vaccine. Vaccine, 27, 298–306.
- Lee, K.Y., Choi, H.J., Park, K.J., Woo, S.J., Kim, Y.M. & Han, J.Y. (2022). Development and characterization of a CRISPR/Cas9-mediated RAG1 knockout chicken model lacking mature B and T cells. Frontiers in Immunology, 13.
- Lee, H.C., Lim, S. & Han, J.Y. (2016). Wnt/β-catenin signaling pathway activation is required for proliferation of chicken primordial germ cells in vitro. Scientific Reports, 6, 34510.
- Lee, J., Ma, J. & Lee, K. (2019). Direct delivery of adenoviral CRISPR/Cas9 vector into the blastoderm for generation of targeted gene knockout in quail. Proceedings of the National Academy of Sciences, 116, 13288–13292.
- Li, T., Huang, S., Jiang, W.Z., Wright, D., Spalding, M.H., Weeks, D.P. & Yang, B. (2011). TAL nucleases (TALNs): hybrid proteins composed of TAL effectors and FokI DNA-cleavage domain. Nucleic Acids Research, 39, 359–372.
- Li, K., Liu, Y., Xu, Z., Zhang, Y., Yao, Y., Nair, V., Liu, C., Zhang, Y., Gao, Y., Qi, X., Cui, H., Gao, L. & Wang, X. (2020). Prevention of avian retrovirus infection in chickens using CRISPR-Cas9 delivered by Marek’s disease virus. Molecular Therapy - Nucleic Acids, 21, 343–353.
- Liu, Y., Mi, Y., Mueller, T., Kreibich, S., Williams, E.G., Van Drogen, A., Borel, C., Frank, M., Germain, P.L., Bludau, I. & Mehnert, M. (2019). Multi-omic measurements of heterogeneity in HeLa cells across laboratories. Nature Biotechnology, 37, 314–322.
- Liu, Y., Xu, Z., Zhang, Y., Yu, M., Wang, S., Gao, Y., Liu, C., Zhang, Y., Gao, L., Qi, X., Cui, H., Pan, Q., Li, K. & Wang, X. (2020). Marek’s disease virus as a CRISPR/Cas9 delivery system to defend against avian leukosis virus infection in chickens. Veterinary Microbiology, 242, 108589.
- Long, J.S., Idoko-Akoh, A., Mistry, B., Goldhill, D., Staller, E., Schreyer, J., Ross, C., Goodbourn, S., Shelton, H., Skinner, M.A., Sang, H., McGrew, M.J. & Barclay, W. (2019). Species specific differences in use of ANP32 proteins by influenza a virus. ELife, 8, e45066.
- Lu, Y., West, F.D., Jordan, B.J., Mumaw, J.L., Jordan, E.T., Gallegos-Cardenas, A., Beckstead, R.B. & Stice, S.L. (2012). Avian-induced pluripotent stem cells derived using human reprogramming factors. Stem Cells and Development, 21, 394–403.
- Macdonald, J., Taylor, L., Sherman, A., Kawakami, K., Takahashi, Y., Sang, H.M. & McGrew, M.J. (2012). Efficient genetic modification and germ-line transmission of primordial germ cells using piggyBac and Tol2 transposons. Proceedings of the National Academy of Sciences, 109, E1466–E1472.
- Makarova, K.S., Wolf, Y.I., Alkhnbashi, O.S., Costa, F., Shah, S.A., Saunders, S.J., Barrangou, R., Brouns, S.J., Charpentier, E., Haft, D.H. & Horvath, P. (2015). An updated evolutionary classification of CRISPR–Cas systems. Nature Reviews Microbiology, 13, 722–736.
- McGrew, M.J., Sherman, A., Ellard, F.M., Lillico, S.G., Gilhooley, H.J., Kingsman, A.J., Mitrophanous, K.A. & Sang, H. (2004). Efficient production of germline transgenic chickens using lentiviral vectors. EMBO Reports, 5, 728–733.
- Minematsu, T., Kanai, Y. & Tajima, A. (2004). Effects of ultraviolet irradiation on the migratory ability of primordial germ cells (PGCs) in the domestic chicken. The Journal of Poultry Science, 41, 110–119.
- Morales, C.P., Holt, S.E., Ouellette, M., Kaur, K.J., Yan, Y., Wilson, K.S., White, M.A., Wright, W.E. & Shay, J.W. (1999). Absence of cancer–associated changes in human fibroblasts immortalized with telomerase. Nature Genetics, 21, 115–118.
- Mottet, A. & Tempio, G. (2017). Global poultry production: current state and future outlook and challenges. World's Poultry Science Journal, 73, 245–256.
- Nakamura, Y., Usui, F., Ono, T., Takeda, K., Nirasawa, K., Kagami, H. & Tagami, T. (2010). Germline replacement by transfer of primordial germ cells into partially sterilized embryos in the Chicken. Biology of Reproduction, 83, 130–137.
- Nash, T.J., Morris, K.M., Mabbott, N.A. & Vervelde, L. (2021). Inside-out chicken enteroids with leukocyte component as a model to study host–pathogen interactions. Communications Biology, 4, 1–15.
- Nuñez, J.K., Chen, J., Pommier, G.C., Cogan, J.Z., Replogle, J.M., Adriaens, C., Ramadoss, G.N., Shi, Q., Hung, K.L., Samelson, A.J., Pogson, A.N., Kim, J.Y.S., Chung, A., Leonetti, M.D., Chang, H.Y., Kampmann, M., Bernstein, B.E., Hovestadt, V., Gilbert, L.A. & Weissman, J.S. (2021). Genome-wide programmable transcriptional memory by CRISPR-based epigenome editing. Cell, 184, 2503–2519.e17.
- O’Geen, H., Bates, S.L., Carter, S.S., Nisson, K.A., Halmai, J., Fink, K.D., Rhie, S.K., Farnham, P.J. & Segal, D.J. (2019). Ezh2-dCas9 and KRAB-dCas9 enable engineering of epigenetic memory in a context-dependent manner. Epigenetics & Chromatin, 12, 26.
- Pain, B., Clark, M.E., Shen, M., Nakazawa, H., Sakurai, M., Samarut, J. & Etches, R.J. (1996). Long-term in vitro culture and characterisation of avian embryonic stem cells with multiple morphogenetic potentialities. Development, 122, 2339–2348.
- Park, K.J., Kang, S.J., Kim, T.M., Lee, Y.M., Lee, H.C., Song, G. & Han, J.Y. (2010). Gamma-irradiation depletes endogenous germ cells and increases donor cell distribution in chimeric chickens. In Vitro Cellular & Developmental Biology - Animal, 46, 828–833.
- Park, T.S. & Han, J.Y. (2012). PiggyBac transposition into primordial germ cells is an efficient tool for transgenesis in chickens. Proceedings of the National Academy of Sciences, 109, 9337–9341.
- Pellegrino, E. & Gutierrez, M.G. (2021). Human stem cell-based models for studying host-pathogen interactions. Cellular Microbiology, 23, e13335.
- Pereira-Smith, O.M. & Smith, J.R. (1988). Genetic analysis of indefinite division in human cells: identification of four complementation groups. Proceedings of the National Academy of Sciences, 85, 6042–6046.
- Rath, N.C., Liyanage, R., Gupta, A., Packialakshmi, B. & Lay, J.O. (2018). A method to culture chicken enterocytes and their characterization. Poultry Science, 97, 4040–4047.
- Romito, A. & Cobellis, G. (2015). Pluripotent stem cells: current understanding and future directions. Stem Cells International, 2016, e9451492.
- Rothkamm, K., Krüger, I., Thompson, L.H. & Löbrich, M. (2003). Pathways of DNA double-strand break repair during the mammalian cell cycle. Molecular and Cellular Biology, 23, 5706–5715.
- Salter, D.W., Smith, E.J., Hughes, S.H., Wright, S.E., Fadly, A.M., Witter, R.L. & Crittenden, L.B. (1986). Gene insertion into the chicken germ line by retroviruses. Poultry Science, 65, 1445–1458.
- Sato, Y., Kasai, T., Nakagawa, S., Tanabe, K., Watanabe, T., Kawakami, K. & Takahashi, Y. (2007). Stable integration and conditional expression of electroporated transgenes in chicken embryos. Developmental Biology, 305, 616–624.
- Schusser, B., Collarini, E.J., Pedersen, D., Yi, H., Ching, K., Izquierdo, S., Thoma, T., Lettmann, S., Kaspers, B., Etches, R.J., van de Lavoir, M.-C., Harriman, W. & Leighton, P.A. (2016). Expression of heavy chain-only antibodies can support B-cell development in light chain knockout chickens. European Journal of Immunology, 46, 2137–2148.
- Schusser, B., Collarini, E.J., Yi, H., Izquierdo, S.M., Fesler, J., Pedersen, D., Klasing, K.C., Kaspers, B., Harriman, W.D., Lavoir, M.-C. van de., Etches, R.J. & Leighton, P.A. (2013). Immunoglobulin knockout chickens via efficient homologous recombination in primordial germ cells. Proceedings of the National Academy of Sciences, 110, 20170–20175.
- Seki, A. & Rutz, S. (2018). Optimized RNP transfection for highly efficient CRISPR/Cas9-mediated gene knockout in primary T cells. Journal of Experimental Medicine, 215, 985–997.
- Shalem, O., Sanjana, N.E., Hartenian, E., Shi, X., Scott, D.A., Mikkelson, T., Heckl, D., Ebert, B.L., Root, D.E., Doench, J.G. & Zhang, F. (2014). Genome-scale CRISPR-Cas9 knockout screening in human cells. Science (New York, N.Y.), 343, 84–87.
- Shay, J.W., Wright, W.E. & Werbin, H. (1991). Defining the molecular mechanisms of human cell immortalization. Biochimica et Biophysica Acta (BBA) - Reviews on Cancer, 1072, 1–7.
- Shi, J., Xue, C., Liu, W. & Zhang, H. (2019). Differentiation of human-induced pluripotent stem cells to macrophages for disease modeling and functional genomics. Current Protocols in Stem Cell Biology, 48, e74.
- Soice, E. & Johnston, J. (2021). Immortalizing cells for human consumption. International Journal of Molecular Sciences, 22, 11660.
- Spence, J.R., Mayhew, C.N., Rankin, S.A., Kuhar, M.F., Vallance, J.E., Tolle, K., Hoskins, E.E., Kalinichenko, V.V., Wells, S.I., Zorn, A.M., Shroyer, N.F. & Wells, J.M. (2011). Directed differentiation of human pluripotent stem cells into intestinal tissue in vitro. Nature, 470, 105–109.
- Takahashi, A., Ohtani, N. & Hara, E. (2007). Irreversibility of cellular senescence: dual roles of p16INK4a/Rb-pathway in cell cycle control. Cell Division, 2, 10.
- Trefil, P., Aumann, D., Koslová, A., Mucksová, J., Benešová, B., Kalina, J., Wurmser, C., Fries, R., Elleder, D., Schusser, B. & Hejnar, J. (2017). Male fertility restored by transplanting primordial germ cells into testes: a new way towards efficient transgenesis in chicken. Scientific Reports, 7, 14246.
- Tyack, S.G., Jenkins, K.A., O’Neil, T.E., Wise, T.G., Morris, K.R., Bruce, M.P., McLeod, S., Wade, A.J., McKay, J., Moore, R.J., Schat, K.A., Lowenthal, J.W. & Doran, T.J. (2013). A new method for producing transgenic birds via direct in vivo transfection of primordial germ cells. Transgenic Research, 22, 1257–1264.
- van de Lavoir, M.-C., Diamond, J.H., Leighton, P.A., Mather-Love, C., Heyer, B.S., Bradshaw, R., Kerchner, A., Hooi, L.T., Gessaro, T.M., Swanberg, S.E., Delany, M.E. & Etches, R.J. (2006). Germline transmission of genetically modified primordial germ cells. Nature, 441, 766–769.
- Wang, W., Zhang, T., Wu, C., Wang, S., Wang, Y., Li, H. & Wang, N. (2017). Immortalization of chicken preadipocytes by retroviral transduction of chicken TERT and TR. PLoS One, 12, e0177348.
- Welch, M.D. (2015). Why should cell biologists study microbial pathogens? Molecular Biology of the Cell, 26, 4295–4301.
- Whyte, J., Glover, J.D., Woodcock, M., Brzeszczynska, J., Taylor, L., Sherman, A., Kaiser, P. & McGrew, M.J. (2015). FGF, insulin, and SMAD signaling cooperate for avian primordial germ cell self-renewal. Stem Cell Reports, 5, 1171–1182.
- Williams, R.M., Senanayake, U., Artibani, M., Taylor, G., Wells, D., Ahmed, A.A. & Sauka-Spengler, T. (2018). Genome and epigenome engineering CRISPR toolkit for in vivo modulation of cis-regulatory interactions and gene expression in the chicken embryo. Development, 145, dev160333.
- Winding, P. & Berchtold, M.W. (2001). The chicken B cell line DT40: a novel tool for gene disruption experiments. Journal of Immunological Methods, 249, 1–16.
- Woodcock, M.E., Idoko-Akoh, A. & McGrew, M.J. (2017). Gene editing in birds takes flight. Mammalian Genome, 28, 315–323.
- Young, C.S., Hicks, M.R., Ermolova, N.V., Nakano, H., Jan, M., Younesi, S., Karumbayaram, S., Kumagai-Cresse, C., Wang, D., Zack, J.A., Kohn, D.B., Nakano, A., Nelson, S.F., Miceli, M.C., Spencer, M.J. & Pyle, A.D. (2016). A single CRISPR-Cas9 deletion strategy that targets the majority of DMD patients restores dystrophin function in hiPSC-derived muscle cells. Cell Stem Cell, 18, 533–540.
- Yue, H., Li, D., Fu, A., Ma, L., Yang, F. & Tang, C. (2008). ShRNA-triggered RNAi inhibits expression of NDV NP gene in chicken embryo fibroblast. Frontiers of Biology in China, 3, 433.
- Zetsche, B., Gootenberg, J.S., Abudayyeh, O.O., Slaymaker, I.M., Makarova, K.S., Essletzbichler, P., Volz, S.E., Joung, J., van der Oost, J., Regev, A., Koonin, E.V. & Zhang, F. (2015). Cpf1 is a single RNA-guided endonuclease of a class 2 CRISPR-Cas system. Cell, 163, 759–771.
- Zhang, H., Shi, J., Hachet, M.A., Xue, C., Bauer, R.C., Jiang, H., Li, W., Tohyama, J., Millar, J., Billheimer, J., Phillips, M.C., Razani, B., Rader, D.J. & Reilly, M.P. (2017). CRISPR/Cas9-mediated gene editing in human iPSC-derived macrophage reveals lysosomal acid lipase function in human macrophages—brief report. Arteriosclerosis, Thrombosis, and Vascular Biology, 37, 2156–2160.
- Zhang, Y., Luo, J., Tang, N., Teng, M., Reddy, V.R.A.P., Moffat, K., Shen, Z., Nair, V. & Yao, Y. (2019). Targeted editing of the pp38 gene in Marek’s disease virus-transformed cell lines using CRISPR/Cas9 system. Viruses, 11, 391.
- Zuo, Q., Wang, Y., Cheng, S., Lian, C., Tang, B., Wang, F., Lu, Z., Ji, Y., Zhao, R., Zhang, W., Jin, K., Song, J., Zhang, Y. & Li, B. (2016). Site-directed genome knockout in chicken cell line and embryos can use CRISPR/Cas gene editing technology. G3 Genes|Genomes|Genetics, 6, 1787–1792.