Abstract
Since aerosol inhalation is the most common mechanism for COVID-19 infection, the respiratory protective devices (RPDs) have the highest importance in personal protection. The aim of this study was to assess the efficiency of 10 different RPDs in shortening the travelling distance of exhaled air by range measurement using the schlieren imaging technique. When a RPD is worn by a person resting in a seated position, the expired air does not exceed the human convective boundary layer (CBL). Instead, the CBL lifts the expired aerosols vertically up. Thus, they have a prolonged travelling time in the surrounding air and become less harmful by several mechanisms of virus content decay. Coughing as well as expiration valves can cause far reaching expiration air clouds that cross horizontally the human CBL by opening leakage airway corridors into different directions. Measured by the range of expired air an FFP2 mask provided high security under all conditions tested. A non-vented full-face mask with two viral filters performed even better because of its airtight fit and the excellent filtering capacity of the viral filters during inspiration and expiration, even during cough manoeuvres.
1. Introduction
During the COVID-19 pandemic, the personal protective equipment (PPE) came in focus as a means to suppress the transmission of the virus. Since then, the quality and efficacy of PPE have been constantly discussed as the safety standards provided by PPE is of vital importance, especially for healthcare workers. To ensure these standards, the Regulation (EU) 2016/425 requires manufacturers of PPE to comply with “essential health and safety requirements” [Citation1].
Since aerosol inhalation is the most common mechanism for COVID-19 infection, a subset of the PPE, the filtering facepiece (FFP), has the highest importance in personal protection. These devices have to meet the PPE regulation and quality criteria specified in several European standards (EN). When these standards are met, the devices are marked with the European conformity CE mark and are then allowed to be traded in the EU internal market. The European standard EN 149 classifies masks according to their efficiency of filtering 0.3 μm particles [Citation2]. FFP1 filter 78% of the particles, while FFP2 and FFP3 filter 92% and 98% of the particles, respectively. Only FFP3 and FFP2 masks are recommended for protection when handling COVID-19-infected persons or material [Citation2]. However, FFP3 masks are sometimes equipped with expiration valves that keep the mask fixed in its position on the face during expiration. These valves prevent mask loosening from the skin which may occur due to the pressure of the generated air cushion against the tightly woven filtering material.
In the European standards EN 140 and EN 143, elastomer and techno-polymer half masks are specified as alternative respiratory protective devices (RPD, EN 140) [Citation3] together with their replaceable filters (EN 143) [Citation4]. These devices have the advantage of tight adaptation to the skin surface. The filtering efficiency is defined in the same way as for FFPs. The efficiency in filtering 0.3 μm particles is 92% for P2 filters and 98% for P3 filters. The EN 140 masks can be considered protective when equipped with a P2 or a P3 filter.
The US standard NIOSH (42 CFR 84) "N" [Citation5–9] and the Chinese standard GB2626 [Citation10] "KN" define similar protective levels. N95/KN95 masks filter ≥95% of 0.3 μm particles, N99/KN99 masks ≥99%, and N100/KN100 masks ≥99,97%. These masks are permitted for use in European countries as well since the beginning of the COVID-19 pandemic.
There are generally two groups of masks users, the healthcare workers and the general population in public areas. Healthcare personnel implements medical masks for which the protection requirements are specified in the European standard EN 14683 [Citation11]. These masks have the function to protect patients from contamination by medical staff. They can filter droplets and larger aerosols from the expired air but fail to protect the carrier against particles of virus size. The corresponding standard for medical masks is ASTM F2100 in the US and YY 0469 for China. For the second group, the general population, unclassified and unmarked masks were recommended in 2020. This included any kind of fabric of cloth that covers the nose and the mouth. This recommendation emerged after the acute shortage of PPE due to the lockdown in China, where the largest portion of the global PPE is produced [Citation12]. Depending on the density of the woven material, the filtering efficiency of such face coverings is 30–90% [Citation13].
Most studies that investigate the efficiency of RPD focus on the filtering efficiency of the material from which the RPD devices are made [Citation14–21]. This is often done by fitting the mask border airtight to the surface of a manikin; human test subjects are not typically used in such studies. In addition, most studies focus on the efficiency of RPD in protecting the person wearing the device from potentially harmful aerosols or particles in the surrounding air. During the COVID-19 pandemic, the RPD function of catching potentially harmful aerosols of an infected person wearing the device became more important, especially when they are used in public spaces to protect others against infection. This function of an RPD is the focus of this study.
This study aims to test the efficiency of the different RPD by visualising the range of human’s expired air leaking through RPDs. The visualisation and characterisation of the expiratory airflow was done using schlieren imaging technique [Citation22–25]. The airflow is visualised by capitalising the refraction of light when it passes through mediums of different densities. This is achieved using a schlieren mirror to visualise density gradients caused by differences in the temperature of the visualised flow compared to the surrounding room air. Several studies in the literature have implemented the schlieren imaging to study the transmission of expired air since it requires no tracer particles, as does particle image velocimetry (PIV), where particle seeding can be difficult to control. Tang et al. utilised Schlieren imaging to examine the spread of airborne contamination during talking and laughing [Citation24] and the dynamics of coughing [Citation25]. Xu et al. [Citation22] used schlieren imaging to investigate the transmission of exhaled air from standing and laying test subjects. Even though the Schlieren imaging provides safe, non-invasive measurements of the exhalation field of the test subject, it should be noted that it cannot visualise airborne particles. Hence, the spread of exhaled air but not of particles are within the scope of the present study. From previous imaging studies the range of expired air during spontaneous breathing and during coughing is well known to be 1 m [Citation24–27]. In coughing series, the range can be extended towards 2 m [Citation25]. The protective effect of mask wearing by shortening the range of expired air as a means of infection control has already been shown in Schlieren optical studies [Citation24,Citation25]. The focus of this study is on the effect of RPD in shortening the travelling distance of exhaled air as well as in influencing the direction and splitting of exhaled airstreams in dependence on mask type and filtering material.
2. Materials and methods
This study was conducted using the schlieren imaging system of the Bauhaus-University Weimar (). The implemented system is a single-mirror coincident schlieren system. It utilises a single large spherical field mirror; the light beams illuminating the mirror and forming the image in the camera are essentially coincident. The setup consists of four elements: concave spherical mirror (1 m in diameter, 3 m in focal length), LED light source + beam splitter, schlieren cut-off, and a digital camera (Canon EOS 5DS R, with 50.6 megapixels image size and a 135 mm focal-length lens) [Citation28,Citation29]. For the purpose of this investigation, video-imaging is captured at 50 frames/s.
Data were collected using a human test subject seated in front of the mirror (). This is advantageous in comparison to artificial body simulators and thermal manikins since the results may differ from human subjects. The test subject was a healthy 33 years old male, 177 cm height with a BMI of 20.4 kg/m2. Different types of RPD were tested including medical masks, community masks, and an AcuCare® full-face mask with Iso-Gard® filters [Citation30]. According to the manufacturer, the Iso-Gard® filters have a much higher quality than P3 filters of the EN 143 standard. The bacterial efficiency is 99.9999% and the viral efficiency >99.99%. The two surgical masks did not differ in filtering quality of the material, but in the way to fix them at the head. While the surgical mask green had flexible straps to fix it at the ears the Barrier® model had tight fastening straps that are tied behind the head and neck. Two community masks were made from 2-fold layer cloth and two from 2-fold layer finest knitwear with a filtering material between both layers. The FFP2, FFP3, and N95 masks were industrial made standard types. The tested RPD types are listed in .
Figure 2. Test subject equipped with respiratory inductance plethysmography (RIP) belts seating in front of the schlieren mirror during N95 mask (left picture) and Barrier® blue surgical mask (right picture) investigation.
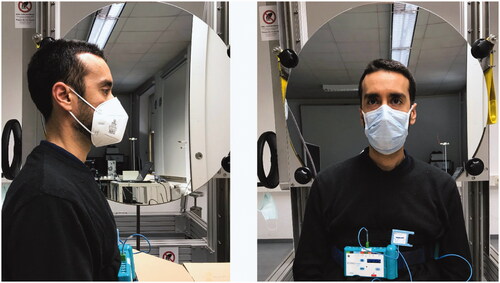
Table 1. Tested RPD types, standards, manufacturers, and photographs.
To demonstrate range and direction of the expired air, we recorded Schlieren image videos in two different planes (lateral and anterior). The test subject was asked to breathe calmly as well as to voluntarily cough while wearing the mask to investigate both scenarios. The volunteer was equipped with respiratory inductance plethysmography (RIP) belts that were calibrated before each measurement session with a pneumotachograph (belts and pneumotachograph: SOMNOmedics, Randersacker, Germany) attached to a non-vented full-face mask (AcuCare® Full Face Mask, ResMed Healthcare, Australia). The signals from the RIP belts were processed by a SOMNOscreen plus® recorder (SOMNOmedics, Randersacker, Germany) and transmitted wirelessly to a receiver connected to a standard notebook on which the respective recording/analysing Software DOMINO® (SOMNOmedics, Randersacker, Germany) was installed. This allowed the retrospective video-synchronized breath by breath analysis of airflow and expiratory tidal volume with a time resolution/sampling rate of 32 Hz ().
2.1 Range of expired air
For each RPD, a one-minute schlieren video was recorded with a framerate of 50 Hz. Since the acquired data are in video format, MATLAB® was used to convert it into single image frames (3000 image frames for each). Hence, selected frames were imported into AutoCAD to measure the spread distance from the source (face with) RPD to the wavefront of the expired air (). Similarly, the border of the expired air wavefront was marked, thus the areas are determined (). Moreover, since the time interval between the wavefronts is known (20 milliseconds), the expired air velocity can also be calculated.
However, in cases where the expired air exceeded the edge of the schlieren mirror, linear regression was used to extrapolate the air velocity down to zero (complete stasis) to calculate the maximum spread distance of the expired air.
The wave front of the expired air is clearly visible only during the lateral view investigation (); thus, the extrapolation was only possible for the lateral view. In the case of the anterior view, the convective flow rising from the body of the test subject mixes with the wave front of the exhaled breath, making it difficult to identify the wave front. Therefore, where the expired air exceeded the edge of the schlieren mirror during anterior investigations, the greater than symbol (“>”) were used to emphasise the potential spread distance based on the observable speed at the edge of the schlieren mirror ().
2.2 Direction and uplift-effect
The human body is constantly exchanging metabolic heat with the surrounding environment because of the human body’s thermoregulation process. Due to heat release from the body, the air adjacent to the skin or clothing is warmer than the surrounding air, resulting in a temperature or density gradient layer in the vicinity of the human body [Citation31]. This layer is known as the human convective boundary layer (CBL). Due to natural convection (buoyancy force), airflow in the CBL travels upwards and transforms into the so-called thermal plume above the body (). At the lower part of body, the CBL is characterised with a laminar flow. However, it gradually increases in thickness and velocity and finally converts to full turbulent flow above the head [Citation29] The CBL continuously alters the direction of expired air during exhalation. In this study, this alteration is referred to as the uplift-effect.
Figure 4. Schlieren image of thermal plume above thermal manikin. The thermal manikin is used to simulate heat release from the human body.
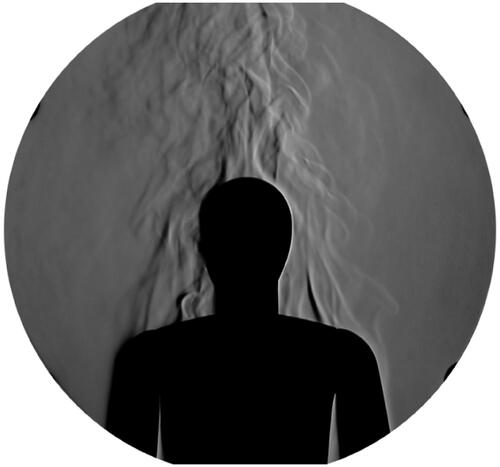
To investigate the major direction/s of the expired air and the uplift-effect, axes of a two-dimensional cartesian system were placed exactly at the RPD (). Hence, the area of the expired air is divided in to four quadrants. Due to the directions of the flow, only the first (I) and the second (II) quadrant expiration air corridors are categorised as the areas affected by the thermal plume convection, thus considered as uplift-effect areas.
Figure 5. Schlieren image of the lateral and anterior views of the coughing manoeuvre with an N95 face mask. The proportions of the amount of air expired into the different directions is calculated by the size of areas of expired air in the different directions defined by a two-dimensional cartesian system.
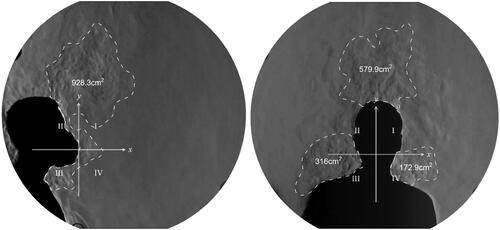
3. Results
When a RPD is worn by a person resting in a seated position, the expired air does not exceed the human convective layer. Instead, the thermal plume lifts the expired air vertically up. In order to estimate the efficiency of the different masks under challenging conditions, our volunteer performed coughing manoeuvres. and show the mean expired air range during 3–4 cough manoeuvres, major direction/s of the expiration air corridors, estimated proportions of expired tidal volume per air corridor, and calculated uplift-effect by thermal plume convection. The tables reveal vastly heterogeneous results.
Table 2. Lateral view investigations.
Table 3. Anterior view investigations.
illustrates the corresponding range of expired air in lateral and anterior views with the tested RPD types during the cough manoeuvres. As shown in I and II, the standard green surgical mask and the Barrier® surgical mask feature similar results in blocking the forward expired air flows (lateral views). In contrast, the anterior views reveal the disadvantages of the standard green surgical mask as it has a lower ability to slow down the speed of the air passing through leaks between skin and mask border into the lateral direction. The air leaving the surgical green mask even crosses the convective layer of the human body and reaches a range that exceeds 0.4 m to the sides of the test subject ( and Citation3). Therefore, the expired air is not fully lifted up vertically by the thermal plume when using the surgical green mask.
Table 4. Range of expired air in lateral and anterior views during cough manoeuvre.
The cloth mask (Unmarked 1) as well as knitwear masks (Unmarked 3 and 4) with filtering inlet, achieved a relatively low performance in blocking the exhaled air (, I, III, and IV). Nevertheless, the double-layer handmade cloth mask (Unmarked 2) had a very good performance in this test. It created an airway corridor upwards with an intervening angle of around 60°, which was within the diameter of the CBL (, II).
The FFP2 mask (, I) performed especially well in slowing down the airflow through the filtering material into all directions. Moreover, it fitted tight enough that no leakage occurred during coughing. On the other hand, the tested FFP3 mask has an inbuilt expiration valve that facilitates breathing by ejecting the expired air without having to force it through the filtering material (, II). The coughing manoeuvre showed in the schlieren images that this mask type should never be worn by an infected individuals because the unfiltered expired air passes the expiration valve with a high velocity in a small airflow corridor. This expired jet reaches a range far beyond the mirror range with a declining inclination of 28°.
Similar to the FFP2 mask, the N95 mask performed well in slowing down the airflow through the filtering material; the airflow was yet ascending especially at the mask borderlines in the region of cheeks and eyes thus producing far reaching airflows into this direction (). This should be considered when patients wearing such RPDs are handled in a supine position.
In contrast to all other RPDs, the AcuCare® full-face mask with two Iso-Gard® filters () always leads the expired air to the border of the CBL where it leaves the filter outlets with high velocity in a small air corridor in the horizontal direction (x-axis) and reaches a range beyond 0.4 m. Yet, this expired air is viral filtered and, thus, free from potentially harmful aerosols. As a result, only in this RPD the range of the expired air does not correspond to the range of potentially harmful expired aerosols. Unlike the other facepieces, in which the expired air produced pronounced refractory disturbances due to higher temperature and higher humidity than the surrounding air, the expired air passing the filters of the AcuCare® full-face mask was hardly visible. In this setting, the expired air is less visible because three effects adjust the refractory index of the filtered air: (1) The air leaving the filters is more laminarized (less turbulent) than the air leaking through the borderline between the other facepieces and the skin; (2) The expired air in the AcuCare® full-face mask has to pass a relatively large volume of dead space via the T-piece until reaching the filters. Therefore, the air is cooled down towards the ambient room temperature before exiting the filters; (3) A part of the air humidity does not pass the filters and accumulates in the AcuCare® mask. Thus, the air leaving the filters is less humid than the air leaking from the other facepieces and the refractive index becomes similar to the surrounding air.
4. Discussion
When a RPD is worn by a person resting in a seated position, the expired air does not exceed the human CBL and becomes vertically lifted up. Thus, the aerosol content has a prolonged travelling time in the surrounding air and becomes less harmful by several mechanisms. In an environment with a relative humidity of 40–60% the aerosols shrink to a smaller size but do not dry out completely. By this effect the concentration of soluble agents raises and causes effective damage to the SARS-CoV-2 content that is inactivated to a greater part by this process [Citation32]. Moreover, the exposure to visible light can also inactivate the SARS-CoV-2 content of the aerosols within 40-60 min [Citation33]. Complete drying leads to a decay of Corona viruses by time with a loss of viability of the last viruses after 72 h [Citation34]. Therefore, the danger of spreading potentially harmful aerosols through a resting or slowly moving person wearing a RPD at normal room temperature seems to be low due to the described uplift effect.
The schlieren mirror measurements of the RPDs allowed visualising their protective effect that has not been previously described when discussing the efficiency of filtering facepieces. Especially when wearing RPDs without expiration valves, the velocity of expired air is slowed down to a speed low enough that in most cases no horizontal crossing of the convective layer is observed. By this effect, up to 100% of the expired air is carried upwards which extends the aerosol travelling time in the surrounding air. In our experimental setting of a resting seated person with normal breathing frequency and tidal volume, this was confirmed for most FFPs.
During coughing manoeuvres, in most RPDs, an air cushion is created inside the mask and relatively large leakages were observed because the borderline of the RPD loses contact to the skin surface. This seems to be the major pathway for unfiltered expired air to spread into the surrounding space of a person. This effect is fully neutralised only in the FFP2 mask and the AcuCare® full-face mask with two Iso-Gard® filters. The double-layer cloth mask “Unmarked 2” came near to that result by opening an airway corridor upwards within the diameter of the convective layer. Only these three RPDs are effective in preventing expired air from an infected upright person from leaving the CBL. However, the FFP2 mask and the AcuCare® full-face mask with two Iso-Gard® filters will be effective when used by a supine transported person as well while the Unmarked 2 mask will not. In the supine position, the main outlet route of the Unmarked 2 mask for the expired air is turned from formally upright by 90° downwards and causes horizontally running currents of unfiltered air that can transport the virus content directly to health care workers walking behind the transported patient. The effectiveness of FFP2 masks for this purpose was confirmed by particle image velocimetry studies from Kähler and Hain [Citation35]. The other tested masks in the present study performed well during normal breathing and speaking only, but not during coughing. In the coughing manoeuvre, airflow corridors with high velocity opened in different directions allowing far-reaching travelling distances for the expired air.
Among all tested RPDs, the AcuCare® full-face mask with two Iso-Gard® filters had an outstanding performance. This RPD was the only one that provided a maximum of safety for both applications: A) When worn by an infected person, the expired air is forced to pass the viral filters. Due to its excellent fitting, it has minimum to no leakage during coughing. By this RPD, most of the potentially harmful aerosols are caught by the filters and eliminated from the expired air. This gives maximum security for surrounding people. B) When worn by healthy persons, it gives maximum security by forcing the potentially dangerous inspired air to pass the viral filters because of the tight fit to the face contour. Inspiring unfiltered air is avoided by having no leakage between the mask borders and the skin.
Our results emphasise the importance of the tight fit of the mask to protect surrounding individuals from being infected by expired aerosols. For this purpose, during coughing, an RPD must reduce the velocity of expired air in any direction down to zero within a distance of 0.1 m to 0.2 m from the mask surface. This will result in the most efficient protection of virus shedding by exhaled air. In the FFP3 mask, the expiration valve was proven to be an inacceptable health risk for the use in public spaces. Thus, while these masks are effective in protecting the wearer against potentially harmful aerosols and dust, they are not suitable to be used in the presence of infectious aerosols. This is of high importance because numerous individuals can be currently observed wearing this kind of FFPs in public spaces.
5. Conclusions
Most studies that investigate the efficiency of RPD focus on the filtering efficiency of the material from which the RPD devices are made. During the COVID-19 pandemic, the RPD function of catching potentially harmful aerosols of an infected person wearing the device became more important, especially when they are used in public spaces to protect others against infection. This function of an RPD is the focus of this study. This study aims to test the efficiency of the different RPD by visualising the range of human’s expired air leaking through RPDs. The effectivity of different mask types is described in this context and the observed positive effect of uplifting exhaled air by the human beings CBL is introduced. In our setting the FFP2 mask and the non-vented mask with two viral filters had an outstanding performance. However, maximum safety was provided by the non-vented mask with two viral filters.
Author contributions
Jens Kerl, Dominic Dellweg, and Conrad Voelker contributed to the study conception and design. Material preparation, data collection and analysis were performed by Jens Kerl, Amayu Wakoya Gena and Hayder Alsaad. The first draft of the manuscript was written by Jens Kerl and all authors commented on previous versions of the manuscript. All authors read and approved the final manuscript.
Disclosure statement
The authors report no conflict of interest.
References
- REGULATION (EU). 2016/425 of the European parliament and of the council of 9 march 2016 on personal protective equipment and repealing council directive 89/686/EEC. Official Journal of the European Union L. 2016.
- DIN EN 149. Respiratory protective devices – Filtering half masks to protect against particles – Requirements, testing, marking (includes Amendment A1:2009) English version of DIN EN 149. 2009. 08. DIN Deutsches Institut für Normung e.V., Berlin. Beuth Verlag GmbH, 10772 Berlin, Germany.
- DIN EN 140. Respiratory protective devices – Half masks and quarter-masks – Requirements, testing, marking; English version of DIN EN 140. 1998. 12. DIN Deutsches Institut für Normung e.V., Berlin. Beuth Verlag GmbH, 10772 Berlin, Germany.
- DIN EN 143. Respiratory protective devices – Particle filters – Requirements, testing, marking; English version of DIN EN 143. 2017. 08 - Draft. DIN Deutsches Institut für Normung e.V., Berlin. Beuth Verlag GmbH, 10772 Berlin, Germany.
- Office of the Federal Register. Code of federal regulations: part 84 - approval of respiratory protective devices. Washington, DC: Office of the Federal Register, National Archives and Records Administration, 1996. (42 CFR part 84).
- 42 CFR Part 84 Respiratory Protective Devices. Government Printing Office in Washington, DC. 1995. Federal Register 08.06.1995 Part II. Final rule effective on 10.07.1995.
- 42 CFR Part 84 - Approval of Respiratory Protective Devices. United States Government Publishing Office. 2020. www.ecfr.gov [Retrieved 9 Feb 2020].
- NIOSH Guide to the Selection and Use of Particulate Respirators. Cincinnati, OH: U.S. Department of Health and Human Services, Public Health Service, Centers for Disease Control and Prevention, National Institute for Occupational Safety and Health, DHHS (NIOSH) Publication No. 96-101, 1996.
- NIOSH Guide to the Selection and Use of Particulate Respirators. The National Institute for Occupational Safety and Health (NIOSH). Centers for Disease Control and Prevention. 6 June 2014. [January 1996]. [Retrieved 9 Feb 2020].
- GB 2626-2019. National Standard of the People’s Republic of China. ICS 13.340.30, C 73. Replacing GB 2626-2006. Respiratory protection – Non-powered air-purifing particle respirator. State Administration for Market Regulation; Standardization Administration of PRC. 2019.
- DIN EN 14683. Medical face masks - Requirements and test methods; English version EN 14683:2019 + AC. 2019. English translation of DIN EN 14683:2019-10. DIN Deutsches Institut für Normung e.V., Berlin. Beuth Verlag GmbH, 10772 Berlin, Germany.
- Cohen J, Rodgers Y. V D M. Contributing factors to personal protective equipment shortages during the COVID-19 pandemic. Prev Med. 2020;141:106263doi.org/10.1016/j.ypmed.2020.106263.
- Maurer L, Peris D, Kerl J, et al. Community masks during the SARS-CoV-2 pandemic: Filtration efficacy and air resistance. J Aerosol Med Pulm Drug Deliv. 2021;34(0):1–9.
- National Institute for Occupational Safety and Hygiene (NIOSH), NIOSH Guide to the Selection and Use of Particulate Respirators Certified Under 42 CFR 84, DHHS (NIOSH) Publication no. 96-101, National Institute for Occupational Safety and Hygiene (NIOSH), Cincinnati, Ohio, USA, 1996.
- Balazy A, Toivola M, Reponen T, et al. Manikin-based performance evaluation of N95 filtering-facepiece respirators challenged with nanoparticles. Ann Occup Hyg. 2006;50(3):259–269.
- Rengasamy S, Eimer BC, Shaffer RE. Comparison of nanoparticle filtration performance of NIOSH-approved and CE-Marked particulate filtering facepiece respirators. Ann Occup Hyg. 2009;53(2):117–128.
- Lee SA, Hwang DC, Li HY, et al. Particle Size-Selective assessment of protection of european standard FFP respirators and surgical masks against Particles-Tested with human subjects. J Healthcare Eng. 2016; 2016:1–12.
- Lee SA, Grinshpun SA, Reponen T. Respiratory performance offered by N95 respirators and surgical masks: human subject evaluation with NaCl aerosol representing bacterial and viral particle size range. Ann Occup Hyg. 2008;52(3):177–185.
- Lee SA, Adhikari A, Grinshpun SA, et al. Respiratory protection provided by N95 filtering facepiece respirators against airborne dust and microorganisms in agricultural farms. Journal of Occup Environ Hygiene. 2005;2(11):577–585.
- Lee SA, Grinshpun SA, Adhikari A, et al. Laboratory and field evaluation of a new personal sampling system for assessing the protection provided by the N95 filtering facepiece respirators against particles. Ann Occup Hyg. 2005;49(3):245–257.
- Leung NHL, Chu DKW, Shiu EYC, et al. Respiratory virus shedding in exhaled breath and efficacy of face masks. Nat Med. 2020;26(5):676–680.
- Xu C, Nielsen PV, Liu L, et al. Human exhalation characterization with the aid of schlieren imaging technique. Build Environ. 2017;112:190–199.
- Tang JW, Noakes CJ, Nielsen PV, et al. Observing and quantifying airflows in the infection control of aerosol- and airborne-transmitted diseases: an overview of approaches. J Hosp Infect. 2011;77(3):213–222.
- Tang JW, Liebner TJ, Craven BA, et al. A schlieren optical study of the human cough with and without wearing masks for aerosol infection control. J R Soc Interface. 2009;6:S727–S736.
- Tang JW, Nicolle ADG, Pantelic J, et al. Qualitative real-time schlieren and shadowgraph imaging of human exhaled airflows: an aid to aerosol infection control. PLoS One. 2011;6/(6):e21392.
- Dellweg D, Kerl J, Gena AW, et al. Exhalation spreading during nasal high-flow therapy at different flow rates. Crit Care Med. 2021;49(7):e693–e700.
- Tang JW, Nicolle ADG, Pantelic J, et al. Airflow dynamics of coughing in healthy human volunteers by shadowgraph imaging: an aid to aerosol infection control. PLoS One. 2012;7/(4):e34818.
- Alsaad H, Voelker C. Qualitative evaluation of the flow supplied by personalized ventilation using schlieren imaging and thermography. Build Environ. 2020;167:106450.
- Gena AW, Voelker C, Settles GS. Qualitative and quantitative schlieren optical measurement of the human thermal plume. Indoor Air. 2020;30(4):757–766.
- Dellweg D, Haidl P, Kerl J, et al. Bench testing of noninvasive ventilation masks with viral filters for the protection from inhalation of infectious respirable particles. J Occup Environ Hyg. 2021;18(3):118–127.
- Voelker C, Alsaad H. Simulating the human body's microclimate using automatic coupling of CFD and an advanced thermoregulation model. Indoor Air. 2018;28(3):415–425.
- Lin K, Marr LC. Humidity-dependent decay of viruses, but not bacteria, in aerosols and droplets follows disinfection kinetics. Environ Sci Technol. 2020;54(2):1024–1032.
- De Santis R, Luca V, Faggioni G, et al. Rapid inactivation of SARS-CoV-2 with LED irradiation of visible spectrum wavelengths. J Photochem Photobiol. 2021;8:100082. DOI:10.1016/j.jpap.2021.100082.
- Rabenau HF, Cinatl J, Morgenstern B, et al. Stability and inactivation of SARS coronavirus. Med Microbiol Immunol. 2005;194(1-2):1–6.
- Kähler CJ, Hain R. Flow analyzes for the SARS-CoV-2 protective mask debate (german: Strömungsanalysen zur SARS-CoV-2 Schutzmaskendebatte). 2020. Universität der Bundeswehr München, Institut für Strömungsmechanik und Aerodynamik, Werner-Heisenberg-Weg 39, 85577 Neubiberg.