Abstract
Five non-biocidal xerogel coatings were compared to two commercial non-biocidal coatings and a silicone standard with respect to antifouling (AF)/fouling-release (FR) characteristics. The formation and release of biofilm of the marine bacterium Cellulophaga lytica, the attachment and release of the microalga Navicula incerta, and the fraction removal and critical removal stress of reattached adult barnacles of Amphibalanus amphitrite were evaluated in laboratory assays. Correlations of AF/FR performance with surface characteristics such as wettability, surface energy, elastic modulus, and surface roughness were examined. Several of the xerogel coating compositions performed well against both microfouling organisms while the commercial coatings performed less well toward the removal of microalgae. Reattached barnacle adhesion as measured by critical removal stress was significantly lower on the commercial coatings when compared to the xerogel coatings. However, two xerogel compositions showed release of 89–100% of reattached barnacles. These two formulations were also tested in the field and showed similar results.
Introduction
Non-biocidal, antifouling (AF)/fouling-release (FR) commercial coatings were designed and developed to release fouling through hydrodynamic shear forces when vessels were under power (Patent GB1470465 Citation1975; Patent WO2002074870 Citation2002; Swain 1999). Several surface and bulk properties including elastic modulus, coating thickness, and critical surface energy (γ C) reportedly influence the mechanism of release of fouling organisms from these surfaces (Brady and Singer Citation2000; Berglin et al. Citation2003; Sun et al. Citation2004; Kim et al. Citation2007; Ramsay et al. Citation2008). Materials with a low elastic modulus deform readily and allow release by interfacial slippage of the adhesive on the surface, which can give unexpected FR characteristics from values of γ C alone (Newby et al. Citation1995). In contrast, more rigid materials cannot release fouling by the same peeling mechanism and, thus, release fouling organisms by shear. With either mechanism, FR occurs from interfacial failure of the bioadhesive with the substratum and/or cohesive failure within the layers of the bioadhesive (Berglin and Gatenholm Citation1999; Berglin et al. Citation2001; Sun et al. Citation2004; Kavanagh et al. Citation2005).
The interactions of bioadhesives with individual AF/FR surfaces will be influenced by the fouling-organism-dependent chemical composition of the bioadhesives as well their mode of secretion, cross-linking and curing (Kamino et al. Citation1996; Callow et al. Citation2002; Dickinson et al. Citation2009; Barlow et al. Citation2010; Wilker Citation2010). The strength and reversibility of attachment of fouling organisms to surfaces is also organism dependent. Zoospores of the macrofouling alga Ulva linza commit themselves to permanent attachment once they have selected a settlement site by secreting a preformed adhesive from cytoplasmic vesicles, which then undergoes a progressive curing process within minutes of secretion (Callow and Callow Citation2002). In contrast, barnacle cypris larvae attach and detach from a surface through temporary adhesives. The temporary adhesives remain on the surface and serve as settlement cues for other cyprids. After a permanent site is selected, the cyprid releases a proteinaceous cement, which cures within 1 to 3 h to affix the cyprid permanently to the surface (Walker 1981). The failure modes of these various adhesives, both interfacial and cohesive, have been examined from the perspective of adhesive left behind on the surface (Sun et al. Citation2004) as well as through visualization of the fracture mechanism such as the viscous fingering seen in Saffman–Taylor instabilities in viscous barnacle cement on low modulus silicone surfaces (Kavanagh et al. Citation2005).
To address the challenges presented by the myriad biological cements and adhesives secreted by microfouling and macrofouling organisms, commercial AF/FR coatings were based on thick, low-surface-energy, low-modulus silicone coatings to minimize adhesion of fouling organisms (Swain 1999). Intersleek 700 (IS700), a silicone-based, low-modulus, FR system (Patent GB1470465 Citation1975), is currently marketed for marine vessels, whose speed and activity ensures adequate shear for the removal of fouling organisms (Dobretsov and Thomason Citation2011). The Intersleek 900 (IS900) FR system is a second-generation, low-modulus FR system with a fluoropolymer-modified silicone composition with improved FR characteristics against micro- and macrofouling organisms (Patent WO2002074870 Citation2002; Dobretsov and Thomason Citation2011). The AF/FR characteristics of these two surfaces have recently been compared (Dobretsov and Thomason Citation2011).
Organically-modified, hybrid xerogel coatings have AF and FR characteristics (Tang et al. Citation2005; McMaster et al. Citation2009; Bennett et al. Citation2010; Finlay et al. Citation2010; Gunari et al. Citation2011), but have surface properties quite different in comparison to silicones, IS700, and IS900. The xerogel materials have tunable surface energies and are thinner (1–10 μm) with a higher elastic modulus (Bennett et al. Citation2010). Approximately 100 boats have been coated with a xerogel AF/FR coating (AquaFast®) and the same material has been used to minimize biofouling on the monitoring system of an underwater archaeological site (Selvaggio et al. Citation2009). One xerogel surface incorporating 1 mole-% of an n-octadecyltrimethoxysilane (C18) precursor in combination with n-octyltriethoxysilane (C8) and tetraethoxysilane (TEOS) released juvenile barnacles and sporelings of the macrofouling algae Ulva linza efficiently with performance comparable to that of a poly(dimethylsiloxane)elastomer standard (Gunari et al. Citation2011). The present authors have also recently described novel xerogel surfaces incorporating 1:4:45:50 mole-% and 1:14:35:50 mole-%, respectively, of C18, tridecafluoro-1,1,2,2-tetrahydrooctyl-triethoxysilane (TDF), C8, and TEOS that performed well with respect to the release of juvenile barnacles and Ulva sporelings (Sokolova et al. Citation2012). The xerogel surfaces are optically transparent and require no ‘tie’ coat for bonding to a variety of substrata, which makes them viable AF/FR candidates for specialized applications on surfaces involved in optics, sensors, solar panels, and related applications.
Herein, the AF and FR performance of several xerogel surfaces are compared with the AF and FR performance of the commercial coatings IS700 and IS900 and a Silastic® T2 (T2) poly(dimethylsiloxane)elastomer standard with respect to micro- and macrofoulers in laboratory assays. Specifically, the biofilm growth and removal of the marine bacterium Cellulophaga lytica, attachment and removal of the marine microalga (diatom) Navicula incerta, and the removal of reattached adult barnacles of Amphibalanus amphitrite were compared. Two of the xerogel coatings showed good FR behavior toward all three fouling organisms. The same two coatings were also tested at a static immersion test site in Morro Bay, California. In the field, both coatings exhibited good FR performance (ie removal of the barnacle Balanus crenatus) and cleaned back relatively easily using a standard water jet apparatus.
Materials and methods
Chemical reagents and materials
Deionized water was prepared to a specific resistivity of at least 18 MΩ using a Barnstead NANOpure Diamond UV ultrapure water system. 3-N,N-dimethylaminopropyltriethoxysilane (DMAP), TEOS, C18, C8 and TDF were purchased from Gelest, Inc. and were used as received. Ethanol was purchased from Quantum Chemical Corp. Hydrochloric acid and borosilicate glass microscope slides were obtained from Fisher Scientific Co.
Sol preparation
The sol/xerogel composition is designated in terms of the molar ratio of Si-containing precursors. Thus, a 50:50 C8/TEOS composition contains 50 mole-% C8 and 50 mole-% TEOS. In all of the sol preparations described below, the aqueous HCl was added last. Unless noted otherwise, all sols were stirred at ambient temperature.
Sol TEOS
TEOS (11.9 g, 51.3 mmol, 10.05 ml), water (1.62 ml, 90 mmol), ethanol (10.2 ml), and HCl (0.10 M, 45 ml) were stirred for 6 h.
Sol DMAP
DMAP (3.16 g, 14.5 mmol) was added dropwise to a mixture of 6.67 M HCl (2.87 ml) and ethanol (14.0 ml). The resulting solution was stirred for 40 min.
1:9 DMAP/TEOS
Sol DMAP (15.3 ml, 11.0 mmol) was added dropwise to sol TEOS (48.6 ml, 99.3 mmol). The mixture was stirred for 20 min.
50:50 C8/TEOS
A mixture of TEOS (20.9 g, 22.4 ml, 0.10 mol), C8 (27.8 g, 31.6 ml, 0.10 mol), isopropanol (40.0 ml), and 0.100 N HCl (12.3 ml, 1.23 mmol) was stirred for 24 h.
1:49:50 C18/C8/TEOS
A mixture of C18 (0.54 g, 1.4 mmol, 0.61 ml), C8 (19.5 g, 70.6 mmol, 22.1 ml), TEOS (15.0 g, 72.0 mmol, 16.1 ml), 0.1 N HCl (9.1 ml, 0.91 mmol), and isopropanol (46.2 ml) was stirred at ambient temperature for 24 h.
1:4:45:50 C18/TDF/C8/TEOS
A mixture of C18 (0.405 g, 1.08 mmol), TDF (2.20 g, 4.3 mmol), C8 (13.4 g, 48.6 mmol), TEOS (11.25 g, 54.0 mmol), ethanol (35.7 ml), and 0.1 M HCl (6.8 ml, 0.68 mmol) was stirred for 24 h.
1:14:35:50 C18/TDF/C8/TEOS
A mixture of C18 (0.405 g, 1.08 mmol), TDF (7.71 g, 15.1 mmol), C8 (10.4 g, 38.1 mmol), TEOS (11.25 g, 54.0 mmol), ethanol (34.5 ml) and 0.1 M HCl (6.8 ml, 0.68 mmol) was stirred for 24 h.
Preparation of coatings
Xerogel films were formed by spin casting 400 μl of the sol precursor onto 25-mm×75-mm glass microscope slides. Prior to use, the slides were soaked in piranha solution for 24 h, rinsed with copious quantities of deionized water, soaked in isopropanol for 10 min, air dried and stored at ambient temperature. A model P6700 spincoater (Specialty Coatings Systems, Inc.) was used at 100 rpm for 10 s to deliver the sol and at 3000 rpm for 30 s to coat.
The xerogel coatings were applied to aluminum Q-panels® (102 × 204 mm, 0.6-mm thickness, type A, alloy 3003 H14, obtained from Q-lab) that had been primed by air-assisted spray with Intergard® 264 at a thickness of 70–80 μm. The sol solutions were applied to the surface by dip-coating and air dried for 7 days to give xerogel surfaces of a thickness of ∼10 μm as determined by profilometry. Discs of the xerogel-coated aluminum were adhered to the bottom of each well of 24-well polystyrene plates as described previously (Stafslien et al. Citation2006, Citation2011).
The xerogel coatings were also applied to G10 fiberglass panels (102×204×4.8 mm, Piedmont Plastics, Inc.) that had been primed by brushing with Interlux® Epoxy Primekote at a thickness of 70–80 μm. The sol solutions were applied to the surface by dip-coating and the resulting surfaces were air dried for 7 days to give xerogel surfaces of a thickness of ∼10 μm as determined by profilometry.
In addition to the experimental coatings, reference T2 poly(dimethylsiloxane) elastomer coatings and the commercial coatings IS700 and IS900 were deposited (0.25 ml) using an Eppendorf Repeater plus pipetter into the wells of 24-well plates (6 columns and 4 rows) modified with primed aluminum discs in each well. The primer used was Intergard® 264. Deposition or adhesion of the discs was done such that a given coating composition occupied three entire columns of the 24-well plate (12 replicate samples per plate). Coatings were allowed to cure for 24 h at room temperature and then heated at 50°C for 24 h to ensure full cure. (While heating to 50°C is not in the manufacturer's specifications, 8 years' experience with this procedure has always given good agreement between laboratory and field testing.) For the barnacle reattachment assay, 1.0 ml of each coating was deposited into three wells of a stamped aluminum panel (Stafslien et al. Citation2011).
Surface energy analysis
Contact angles were measured for all xerogel formulations with a contact angle goniometer (Rame-Hart, Model NRL 100) at room temperature using the sessile drop technique with water and diiodomethane. Surface free energies (γ S) as well as dispersive (γ D) and polar components (γ P) were determined by the method of Owens and Wendt (Citation1969) using static contact angles for xerogel surfaces on glass slides that had been air-dried for 7 days.
Adult barnacle reattachment assay
An adult barnacle reattachment assay was utilized to gauge the FR performance of the coatings with respect to macrofouling (Rittschof et al. Citation2008; Stafslien et al. Citation2011). Adult barnacles of A. amphitrite with a basal diameter of ∼5 mm were removed from a coating of T2 on glass and placed on the coating surfaces that had been pre-conditioned for 14 days in running tap water to remove leachates from the coatings prior to the biological assay. Nine barnacles were allowed to reattach to the coating surfaces by immersing the panels in artificial sea water (ASW) for 14 days with daily feedings of brine shrimp nauplii. The reattached barnacles were dislodged from the coating surfaces using a hand held digital force gauge in accordance with ASTM standard D5618-94. In this regard, the force gauge was placed at the barnacle base plate, parallel to the coating surface, and pushed laterally (ie in shear) until the barnacle became detached from the surface. Once detached, the area of the barnacle base plates were measured using digital image analysis (Sigma Scan Pro 5.0) and barnacle adhesion strengths (ie critical removal stress in shear [CRS] values) were calculated from the removal force and the area of the barnacle basal plates. The adhesion values for each coating were reported as the mean of the total number of barnacles exhibiting a measurable removal force. The number of reattached barnacles that exhibited any detectable shell breakage or baseplate damage during force gauge removal was recorded for each coating (ie fraction removal) and excluded from the CRS calculations.
C. lytica biofilm retention and adhesion
The high-throughput assessment of bacterial biofilm retention on coatings prepared in 24-well plates has been described previously (Stafslien et al. Citation2006, Citation2007a; Majumdar et al. Citation2008). After pre-conditioning for 14 days in running tap water to remove leachates, the coating plates were inoculated with 1.0 ml of a 107 cells ml−1 suspension of C. lytica in biofilm growth medium (0.1 g yeast extract and 0.5 g of peptone 1−1 of ASW) and incubated at 28°C for 24 h. Following incubation, the planktonic growth was discarded and the plates were rinsed three times with ASW to remove unattached cells or biofilm that was only weakly attached to the coating surfaces. The retained biofilms were dried at ambient laboratory conditions for ∼1 h and stained with crystal violet (0.3% w/v in deionized water). The crystal violet stain was subsequently extracted from the biofilm retained on the coating surfaces with 0.5 ml of 33% glacial acetic acid and the resulting eluates were transferred to a 96-well plate and measured for absorbance at 600 nm using a multi-well plate spectrophotometer. The absorbance values (λ = 600 nm) were reported as the mean of three replicate wells and considered to be directly proportional to the amount of biofilm retained on the coating surfaces.
C. lytica biofilm adhesion was determined after incubation for 24 h using an automated water jet apparatus (Stafslien et al. Citation2007b, Citation2011). The first column of each coating was not treated with the water jet and served as the measure of the biofilm retained on the coating surface before water jetting. The second and third column of each coating was jetted for 5 s at a pressure of 69 and 138 kPa, respectively. The percentage removal was recorded as the difference in absorbance values between the coating replicates that were exposed to the water-jet and those which were not.
Attachment and adhesion of N. incerta
The assay for attachment and adhesion of the diatom N. incerta was carried out in a similar manner to the assay for retention and adhesion of C. lytica biofilm (Cassé et al. Citation2007; Stafslien et al. Citation2011). After pre-conditioning for 14 days in running tap water to remove leachates, the coating plates were inoculated with 1.0 ml of a 105 cells ml−1 suspension of N. incerta in F/2 medium (Guillard and Ryther Citation1962) and incubated at ambient laboratory conditions for 2 h. Water jetting followed the incubation of the plates. The first column of each coating was not treated with the water jet and served as the measure of cell attachment of N. incerta on the coating surface before water jetting. The second and third column of each coating was jetted for 5 s at a pressure of 69 and 138 kPa, respectively. One milliliter of DMSO was immediately added to each well and the plates were incubated in darkness for 30 min. A homogeneous solution was obtained by gentle shaking and 0.2 ml of the solution was transferred to a 96-well plate for fluorescence measurements of chlorophyll using a multi-well plate spectrophotometer (excitation wavelength: 360 nm, emission wavelength: 670 nm). The percentage removal was recorded as the difference in relative fluorescence units (RFU) between the coating replicates that were exposed to the water-jet and those which were not.
Field testing
The 1:4:45:50 C18/TDF/C8/TEOS and 1:14:35:50 C18/TDF/C8/TEOS xerogel coatings were applied to fiberglass panels as described above and deployed at a static immersion test site in Morro Bay, CA for a series of field tests. Panels were submerged ∼1 m below the surface from a floating dock. Panels were deployed on 20 April 2011 at the beginning of the heaviest fouling season and were observed monthly over ∼6 months. Panels were subjected to tests quantifying AF and FR performance. AF performance was quantified in accordance with ASTM standard D6990-05. FR performance was estimated using two separate tests, viz. removal of adult barnacles in accordance with ASTM standard D5618-94 and an adaptation of water jet testing (Swain and Schultz Citation1996; Swain 1997).
Adult barnacles (Balanus crenatus) were recruited directly onto panels in the field and then removed from test panels in accordance with ASTM standard D5618-94. Barnacle removal testing was conducted on 24 August 2011 once all individuals met the minimum size requirements for testing according to the ASTM standard. Values of CRS were calculated for all barnacles that could be removed from test panels with less than an estimated 10% basal plate remaining on the coating surface as per the ASTM standard. All barnacles that were isolated and met the minimum size requirements were removed from each panel replicate so sample sizes were not equal. Few barnacles settled and grew on the IS900 control panel so this sample size was much smaller than for the other two test coatings. However, the CRS was consistent with what has been observed in other field trials.
One replicate panel of each formulation was reserved for water-jet testing to provide another measure of FR performance. A pressurized stream of water was applied perpendicular to and ∼25 mm away from the surface as evenly as possible across the entire surface of the panel at a series of pressures (40, 80, 120, 180 and 240 psi). Panels were observed after each water pressure was applied and assessed for complete removal of organisms or estimated percentage cover remaining. These estimates were then used to rank coatings based on how easily biofouling could be removed. All water jet testing was done on about 1 months' accumulated fouling.
AF and physical performance of coatings were evaluated as outlined in ASTM standard D6990-05. Each panel was given a fouling rating with 100 being completely free of fouling. Each panel was also given a physical deterioration rating (PDR), with 100 being completely free of deterioration. Fouling rating is only reported for 21 July 2011 because after that time all panels were altered in order to remove barnacles to measure CRS values.
Results
Characterization of xerogel surfaces
Static water contact angles, θWs, are compiled in along with values of surface free energy (γ S) and the corresponding dispersive (γ D) and polar (γ P) components determined by the method of Owens and Wendt (Citation1969) for the 10:90 DMAP/TEOS (referred to as DMAP/TEOS in the remainder of the manuscript), 50:50 C8/TEOS (referred to as C8/TEOS in the remainder of the manuscript), 1:1:48:50 C18/C8/TEOS (referred to as C18/C8/TEOS in the remainder of the manuscript), 1:4:45:50 C18/TDF/C8/TEOS, and 1:14:35:50 C18/TDF/C8/TEOS xerogel coatings. Literature values of θWs and γ S for T2 (Feinberg et al. Citation2003) and IS700 and IS900 (Dobretsov and Thomason Citation2011) are compiled in as are values of Young's modulus for the 1:4:45:50 and 1:14:35:50 C18/TDF/C8/TEOS xerogels. Literature values of Young's modulus for the remaining xerogels (Bennett et al. Citation2010; Sokolova et al. Citation2012), T2 (Feinberg et al. Citation2003) and the commercial standards IS700 and IS900 (Dobretsov and Thomason Citation2011) are also compiled in as are literature values of root-mean-square roughness for the five xerogel coatings (Bennett et al. Citation2010; Gunari et al. Citation2011; Sokolova et al. Citation2012) and the IS700 and IS900 coatings (Dobretsov and Thomason Citation2011).
Table 1. Experimental and reported values of static water contact angles (θWs), surface energies (γ S) with dispersive (γ S) and polar components (γ S), modulus, and surface roughness for the xerogel surfaces of this study, commercial IS700 and IS900 coatings, and T2 standard.
Values of the root-mean-square roughness for the xerogel surfaces (∼10−9–10−10 m) were calculated from AFM measurements (Bennett et al. Citation2010; Gunari et al. Citation2011; Sokolova et al. Citation2012). AFM images of the 1:14:35:50 C18/TDF/C8/TEOS xerogel prior to and after immersion in water are shown in of the Supplementary material [Supplementary material is available via a multimedia link on the online article webpage]. AFM images of the C8/TEOS, C18/C8/TEOS, and 1:4:45:50 C18/TDF/C8/TEOS xerogels have been previously reported (Gunari et al. Citation2011; Sokolova et al. Citation2012). Values of the root-mean-square roughness for the IS700 and IS900 surfaces (∼6 × 10−5 m) were determined by laser profilometry as described by Dobretsov and Thomason (Citation2011).
AF/FR properties in laboratory testing
Three organisms were used in laboratory testing to characterize the AF and FR properties of the xerogel, T2, IS700, and IS900 coatings. Biofilm growth and removal of the marine bacterium C. lytica and the attachment and removal of the marine microalga N. incerta were measured in this study to determine AF and FR properties towards microfoulers while the adhesion strength of reattached barnacles (A. amphitrite) was used to evaluate the FR properties of the coatings toward a macrofouler.
The significance level, α, for the statistical tests described below was α = 0.05. There were significant differences among the surfaces in the quantity of biofilm attaching to the material (ANOVA p < 0.0001, ) and the amount of biofilm remaining after water-jetting at 69 kPa (ANOVA p < 0.0001, ) and 138 kPa (ANOVA p < 0.0001, ). Biofilm from C. lytica was significantly heavier on the IS700 surface relative to all other surfaces before and after water jetting. Biofilm formation was lower and comparable on all the remaining surfaces (). In terms of percentage removal, the IS900 surface was significantly better in comparison to any of the other surfaces and gave >90% removal of biofilm at a water-jet pressure of either 69 or 138 kPa (). The 1:4:45:50 and 1:14:35:50 C18/TDF/C8/TEOS xerogels exhibited >80% removal at 138 kPa, which was significantly greater in comparison to all other surfaces other than IS900 and C18/C8/TEOS. The C18/C8/TEOS xerogel surface was not significantly different from the two TDF-containing xerogel surfaces. The IS700 and DMAP/TEOS xerogel coatings with <50% biofilm removal at 69 or 138 kPa were significantly poorer performers in comparison to all of the other coatings.
Figure 1. Evaluation of bacterial (C. lytica) biofilm retention on xerogel, T2 and commercial surfaces using crystal violet staining. The absorbance value is directly proportional to the amount of biofilm retained on the coating surface (a) prior to exposure to the water jet (black bars); (b) after a 5-s exposure to a water jet pressure of 69 kPa (gray bars); and (c) after a 5-s exposure to a water jet pressure of 138 kPa (white bars). Absorbance values are reported as the average of three replicates and error bars represent ±1 SD of the mean.
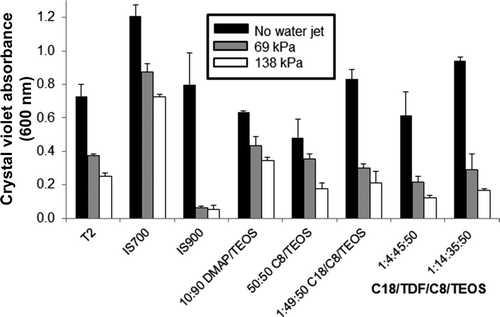
Figure 2. Evaluation of bacterial biofilm adhesion on xerogel, T2 and commercial surfaces. Percentage removal of C. lytica biofilm (a) after a 5-s exposure to a water jet pressure of 69 kPa (black bars) and (b) after a 5-s exposure to a water jet pressure of 138 kPa (white bars). Percentage removal values are reported as the average of three replicates and error bars are ±1 SD of the mean. Coatings that share a letter have values of biofilm removal that are not significantly different from one another.
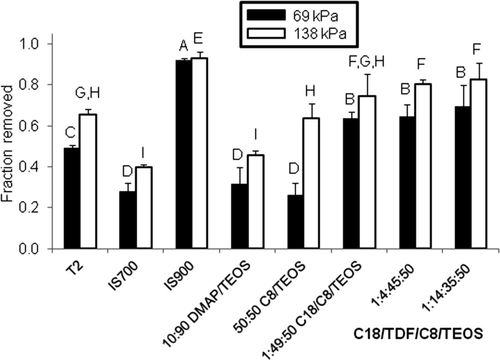
There were significant differences among the surfaces with respect to attachment of the microalga (ANOVA p < 0.0001, ) and the removal of the microalga following water-jetting at 69 kPa (ANOVA p < 0.0001, ) and 138 kPa (ANOVA p < 0.0001, ). Initial microalgal attachment was significantly lower on the C8/TEOS surface relative to all other surfaces, with the exception of IS900. Attachment on all of the xerogel surfaces was significantly lower in comparison to attachment on IS700. The removal of N. incerta biomass was significantly greater on all of the xerogel surfaces (50–72% removal at 69 kPa, 89–97% removal at 138 kPa) in comparison to the T2, IS700, and IS900 surfaces (13–25% removal at 69 kPa, 38–53% removal at 138 kPa, ) at both pressures. At 69 kPa, removal from the 1:14:35:50 C18/TDF/C8/TEOS xerogel coating was significantly greater in comparison to removal from all other coatings, with the exception of the DMAP/TEOS coating. At 138 kPa, removal from the 1:14:35:50 C18/TDF/C8/TEOS xerogel coating was significantly greater in comparison to removal from all other coatings.
Figure 3. Evaluation of microalgal cell attachment on xerogel, T2 and commercial surfaces. The fluorescence value reported (in relative fluorescence units, RFU) is directly proportional to the number of cells of N. incerta attached to the coating surface (a) prior to exposure to the water jet (black bars); (b) after a 5-s exposure to a water jet pressure of 69 kPa (gray bars); and (c) after a 5-s exposure to a water jet pressure of 138 kPa (white bars). Each value is reported as the mean of three replicate measurements. Error bars represent ±1 SD of the mean.
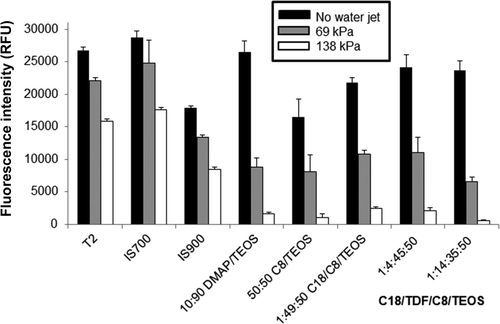
Figure 4. Evaluation of microalgal cell adhesion on xerogel, T2 and commercial surfaces. Percent removal of N. incerta (a) after a 5-s exposure to a water jet pressure of 69 kPa (black bars) and (b) after a 5-s exposure to a water jet pressure of 138 kPa (white bars). Percentage removal values are the average of three replicates and error bars represent ±1 SD of the mean. Coatings that share a letter have values of microalgal cell removal that are not significantly different from one another.
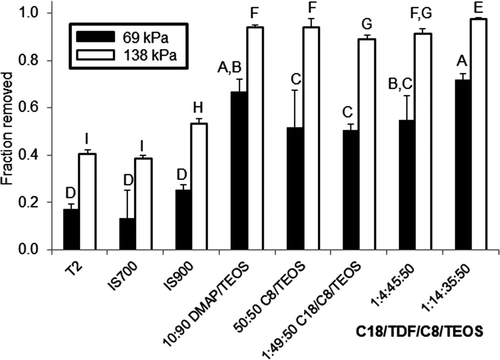
The strength of attachment of reattached adult barnacles was measured via force-gauge measurements with forces applied in shear. Nine barnacles were removed from each surface and the fraction removed completely is shown in a. Complete removal was defined as no detectable shell breakage or basal plate remaining on the coating surfaces after force gauge measurements. The CRS for removal of barnacles from each surface is shown in b. Only those barnacles with <10% basal plate remaining were used in the calculation of CRS as specified by ASTM D 5618-94 1994.
Figure 5. Nine adult barnacles (A. amphitrite) were reattached to the xerogel, T2 and commercial coatings for 2 weeks. (a) Fraction of reattached barnacles removed completely via shear pressure and (b) critical removal stress (CRS) in MPa for barnacles removed completely. Values of CRS are reported as the mean of barnacle displacements that resulted in a measureable force (no shell breakage or base plate damage, <10% basal plate remaining) and error bars represent ±1 SD of the mean. Coatings that share a letter have values of CRS that are not significantly different from one another.
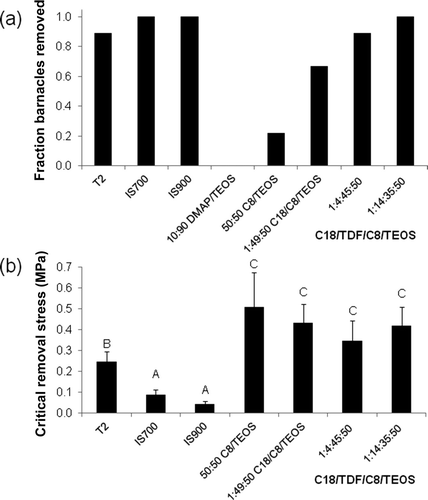
The T2, IS700, IS900, 1:4:45:50 C18/TDF/C8/TEOS xerogel, and 1:14:35:50 C18/TDF/C8/TEOS xerogel surfaces all released ≥89% of the reattached barnacles completely (a). The DMAP/TEOS xerogel surface and the C8/TEOS xerogel surface displayed poor FR behavior (0/9 and 2/9 barnacles, respectively, released completely). The C18/C8/TEOS xerogel was intermediate in behavior (6/9 barnacles released completely).
One-way analysis of variance and Tukey tests indicate that there were significant differences among the surfaces of this study with respect to the CRS (ANOVA p < 0.0001). Values of CRS were significantly lower on the IS700 (0.090 ± 0.025 MPa) and IS900 surfaces (0.043 ± 0.015 MPa) relative to all the other surfaces (b). The CRS for the T2 surface (0.25 ± 0.05 MPa) was significantly lower than for all of the xerogel surfaces. The CRS for the 1:4:45:50 C18/TDF/C8/TEOS xerogel (0.35 ± 0.10 MPa) was the lowest among the xerogels that released barnacles, but none of the values of CRS were significantly different from one another (CRS of 0.35–0.51 MPa).
AF/FR properties in field testing
The 1:4:45:50 C18/TDF/C8/TEOS and 1:14:35:50 C18/TDF/C8/TEOS xerogel coatings were also assessed for AF and FR properties under static immersion conditions in the field. Analysis using a one-way ANOVA and Tukey tests indicate that there were significant differences in the CRS of barnacles among coating surfaces exposed at the static field site (ANOVA p < 0.00001). In the field tests, only those barnacles with <10% basal plate remaining following removal were used in the calculation of CRS. Values of CRS were significantly lower on the IS900 surfaces (0.046 ± 0.012 MPa) in comparison to the 1:4:45:50 C18/TDF/C8/TEOS (0.322 ± 0.018 MPa) or 1:14: 35:50 C18/TDF/C8/TEOS (0.299 ± 0.014 MPa) xerogel coatings, which were not statistically different from one another ().
Figure 6. Adult barnacles (B. crenatus) recruited were allowed to grow and then removed from test panels at the Morro Bay static immersion test site. Sample sizes were not equal due to random recruitment and were 70, 78, and 6 for each coating, respectively. (a) Fraction of barnacles removed completely via shear pressure and (b) critical removal stress (CRS) for barnacles removed completely. Values of CRS are reported as the mean of those animals with no shell breakage or basal plate damage (<10% basal plate remaining). Error bars represent ±1 SD from the mean. Coatings that share a letter have values of CRS that are not significantly different from one another.
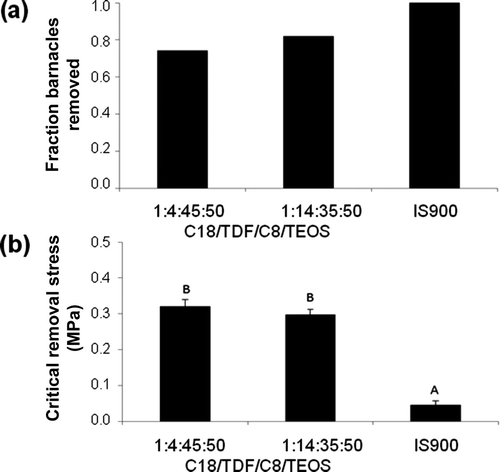
The results for water-jet testing of the 1:4:45:50 C18/TDF/C8/TEOS and 1:14:35:50 C18/TDF/C8/TEOS xerogel surfaces showed that the coatings cleaned back relatively well with only small amounts of hard foulers (in this case encrusting bryozoans) and slime remaining on the surfaces after the highest pressure was applied (). The IS900 control panel cleaned back completely at low pressures and had much less fouling accumulation initially.
Table 2. Summary of water-jet testing.
shows fouling ratings and PDR for the 1:4:45:50 C18/TDF/C8/TEOS and 1:14:35:50 C18/TDF/C8/TEOS xerogel coatings and the IS900 surface. The fouling ratings of the 1:4:45:50 C18/TDF/C8/TEOS and 1:14:35:50 C18/TDF/C8/TEOS xerogel surfaces were significantly lower in comparison to the IS900 control surfaces (ANOVA, p < 0.001) but were not significantly different from each other. No defects were observed on any of the coatings for the duration of testing giving a PDR of 100 for all coatings.
Table 3. Fouling rating (after 3-months testing), physical deterioration rating (PDR, after 6-months testing), number of barnacles removed completely (pushed) and critical removal stress (CRS) for barnacles removed completely for each coating formulation.
Discussion
Surface energy (Becker 1998; Allion et al. Citation2006; Bennett et al. Citation2010; Finlay et al. Citation2010), elastic modulus (Berglin et al. Citation2003; Sun et al. Citation2004), wettability (Bennett et al. Citation2010; Bhushan and Jung Citation2010; Finlay et al. Citation2010), and roughness (Allion et al. Citation2006; Schumacher et al. Citation2007; Mitik-Dineva et al. Citation2009; Bhushan and Jung Citation2010; Myint et al. Citation2010) have all been demonstrated to be important surface properties determining the settlement and the ease of removal of fouling. The elastic modulus in particular influences the fracture mechanism of fouling organisms (Brady and Singer Citation2000; Berglin et al. Citation2003; Kim et al. Citation2007; Ramsay et al. Citation2008). As shown in , T2, IS700, and IS900 (all with an elastic modulus of ∼1 MPa) are low modulus materials. They are typically applied as ‘thick’ coatings (thickness ≥150 μm) and macrofoulers often are released by a peeling mechanism involving interfacial slippage with failure at the bioadhesive-surface interface. The interfacial slippage involves both extensional deformation of the adhesive and shrinkage at the base area of contact (Newby et al. Citation1995). In contrast, the harder (elastic modulus >60 MPa, ), thinner xerogel coatings (∼1–10 μm by profilometry) cannot undergo similar deformation, and release of fouling will be more dependent upon shear (Newby et al. Citation1995; Berglin and Gatenholm Citation1999; Berglin et al. Citation2001). On poly(methyl methacrylate) (PMMA), which is a high-modulus surface (Berglin and Gatenholm Citation1999; Berglin et al. Citation2001), cohesive failure of the adhesive rather than interfacial slippage has been described as the primary mode of adhesive failure for the removal of fouling organisms.
In harder, thinner coatings where interfacial slippage does not facilitate FR, adhesive failure has been correlated with surface energy (γ S) (Newby et al. Citation1995; Brady and Singer Citation2000). The ‘Baier curve’ of adhesion strength vs surface energy has a region from 20–25 mN m−1 where minimal bioadhesion has been reported due to the formation of weak boundary layers between the surface and the adhesives of fouling organisms (Baier et al. Citation1968; Baier 1984).
The coatings of this study provide an interesting contrast between high- (C8/TEOS, C18/C8/TEOS, and 1:4:45:50 C18/TDF/C8/TEOS xerogels) and low-modulus coatings (T2) with surface energies near the Baier minimum (21.3–23.1 mN m−1, ), high- (DMAP/TEOS xerogel) and low-modulus coatings (IS700 and IS900) with surface energies higher in comparison to the Baier minimum (34–54.7 mN m−1, ), and a high-modulus coating (1:14:35:50 C18/TDF/C8/TEOS xerogel) with surface energy below the Baier minimum (17.6 mN m−1, ). The removal of barnacles from these surfaces offers a comparison of the importance of these two parameters, modulus and surface energy. All three low-modulus coatings (T2, IS700, IS900) displayed excellent FR behavior with respect to reattached adult barnacles in the laboratory assay as did the high-modulus C18/TDF/C8/TEOS and 1:14:35:50 C18/TDF/C8/TEOS xerogel coatings (a). When tested in the field the 1:4:45:50 C18/TDF/C8/TEOS and 1:14:35:50 C18/TDF/C8/TEOS xerogels also showed good FR behavior with many barnacles removed completely (52/70 and 64/78 respectively) as did the IS900 surface (6/6 barnacles removed completely).
While both low- and high-modulus coatings released barnacles, the energy required in the removal process as measured by CRS both in the laboratory assay and field testing indicate the importance of modulus to the removal process. Values of CRS for the 1:4:45:50 C18/TDF/C8/TEOS xerogel (0.35 MPa) and 1:14:35:50 C18/TDF/C8/TEOS xerogel (0.42 ± 0.08 MPa) coatings are 4- to 5-fold greater in comparison to CRS for the IS700 coating (0.090 MPa) and 8- to 10-fold greater in comparison to CRS for the IS900 coating (0.043 MPa). The field testing gave similar results with values of CRS for the 1:4:45:50 C18/TDF/C8/TEOS xerogel (0.32 MPa) and 1:14:35:50 C18/TDF/C8/TEOS xerogel (0.30 MPa) coatings roughly 7-fold greater than CRS for the IS900 coating (0.046 MPa). These results show a similar relationship to the CRS values reported for the low surface energy Teflon® and UHM polyethylene with high modulus (0.588 MPa and 0.776 MPa, respectively) when compared to polydimethylsiloxane coatings of lower modulus (0.09 to 0.29 MPa) (Swain et al. Citation1994).
The FR characteristics are contrary to those predicted by surface energy and the Baier curve where the IS700 and IS900 coatings have significantly higher surface energies (p < 0.01, Student t-test) than either the 1:4:45:50 C18/TDF/C8/TEOS or 1:14:35:50 C18/TDF/C8/TEOS xerogel and would be expected to be poorer performers based on surface energy alone (Baier et al. Citation1968; Baier 1984). The T2 standard has a value of surface energy similar to those of the two C18/TDF/C8/TEOS xerogels and within the Baier minimum. However, CRS for the T2 surface (0.25 MPa) is significantly lower (p < 0.03, Student t-test) in comparison to CRS for either C18/TDF/C8/TEOS xerogel surface. It must be remembered, however, that the Baier curve was developed from observations of microfouling and not barnacle attachement (Swain 1999). The results from this research emphasize the importance of a thick, low-modulus surface to provide lower values of CRS for the removal of barnacles.
Since the xerogels are all harder, high-modulus surfaces, the question can be asked whether release of barnacles follows a trend based on surface energies. The strength of protein adhesion to a series of xerogel coatings reproduced the Baier curve with respect to either surface tension (γ S) or critical surface tension (γ C) (Finlay et al. Citation2010). However, the strength of attachment of Ulva sporelings as measured by water-jet pressure for removal did not show similar agreement with the Baier curve for the same series of xerogels (Bennett et al. Citation2010). In the Ulva sporeling study, it was postulated that the amine-containing xerogels might interact chemically with the sporeling adhesive. When the amine-containing xerogels were excluded from the correlation, linear correlations of adhesion strength with either water contact angle or polar contributions to the surface energy (γ P) were then observed (Bennett et al. Citation2010).
The removal of reattached barnacles from the xerogel surfaces roughly correlated with surface energy. The DMAP/TEOS xerogel coating has a value of γ S (54.7 mN m−1) that is well above the Baier zone of minimal bioadhesion and this surface did not release barnacles at all. The basal plate remained affixed to the surface. The 1:4:45:50 C18/TDF/C8/TEOS, C8/TEOS, and C18/C8/TEOS xerogels have values of γ S within the Baier zone of minimal bioadhesion (21.3–23.1 mN m−1) and all three surfaces released some barnacles completely (2/9 for C8/TEOS, 6/9 for C18/C8/TEOS, and 8/9 for 1:4:45:50 C18/TDF/C8/TEOS). The value of γ S for the 1:14:35:50 C18/TDF/C8/TEOS xerogel (17.6 mN m−1) was well outside the Baier zone of minimal bioadhesion, but on the low energy side, yet released 9/9 reattached barnacles completely. All of the values of CRS for the xerogel surfaces that released barnacles were statistically equivalent (0.35–0.51 mN m−1, p > 0.20, Student t-test).
With respect to the xerogel surfaces, factors influencing adhesion strength other than modulus and surface energy can be considered. Surface roughness is one parameter with statistically significant differences in all pair-wise comparisons of the xerogel coatings (, p < 0.005, Student t-test). Among xerogel coatings that released reattached barnacles, the percentage released completely increased with surface roughness. The C8/TEOS xerogel had the lowest value of root-mean-square roughness and released the fewest barnacles, while the 1:14:35:50 C18/TDF/C8/TEOS xerogel, whose topography as measured by AFM is shown in Figure S1 [Supplementary material is available via a multimedia link on the online article webpage], had the highest root-mean-square roughness and released all reattached barnacles.
In comparisons of the C8/TEOS, C18/C8/TEOS, and the two C18/TDF/C8/TEOS xerogels, the increase in root-mean-square roughness is also accompanied by chemical segregation of functionality. The C8/TEOS xerogel is ‘smooth’ topographically by AFM imaging of topography and chemically by IR microscopy of bulk chemical composition (Gunari et al. Citation2011). The C18/C8/TEOS xerogel in contrast shows a network of nanoscale pores by AFM imaging of topography and regions of higher and lower hydrocarbon content in the bulk surface by IR microscopy (Gunari et al. Citation2011). In the two C18/TDF/C8/TEOS xerogels, AFM phase images show islands of harder and softer material, which also appear as chemical inhomogeneities in hydrocarbon and fluorocarbon content in the IR microscopy images (Sokolova et al. Citation2012). Perhaps the chemical ‘mosaic’ presented by the C18/C8/TEOS and the two C18/TDF/C8/TEOS xerogels contributes to the increased fraction of reattached barnacles removed via shear.
The idea of a chemical ‘mosaic’ also comes into play in comparisons of the commercial IS700 and IS900 coatings. With respect to biofilm growth and removal of the C. lytica biofilm, there is no apparent correlation of surface energy (γ S), water contact angle (θWs), elastic modulus or surface roughness with either the AF or FR character. The IS700 and IS900 coatings, with nearly identical values of elastic modulus, surface energy, and roughness were the ‘best’ and ‘worst’ surfaces, respectively, for FR behavior with C. lytica biofilm among the seven coatings of this study. However, the fluoropolymer-modified silicone composition of IS900 provides a chemical ‘mosaic’ (enforced by the block co-polymer structure) not present in the silicone-based IS700, and chemical interactions of the surface with the biofilm may be quite different. It is also interesting that the C18/C8/TEOS and the two C18/TDF/C8/TEOS xerogels, all with demonstrated chemical segregation of functionality, also outperformed the other chemically homogenous xerogel surfaces, T2 silicone, and IS700 silicone with respect to C. lytica removal and were comparable to IS900.
The attachment of diatoms to a surface involves the initial secretion of an adhesive consisting of various proteoglycans (Cooksey and Wigglesworth-Cooksey 1995; Molino and Wetherbee 2008). Attachment of diatoms appears to be stronger on hydrophobic surfaces relative to hydrophilic surfaces (Molino and Wetherbee 2008; Finlay et al. 2010). However, the seven coatings of this study suggest that factors other than surface energy and wettability may be involved. The low-modulus and ‘rough’ commercial T2, IS700, and IS900 coatings all gave poorer FR performance than all of the xerogel coatings with respect to percentage removal of microalgae biomass () and/or the remaining microalgal biomass (). These data suggest that the diatoms adhere more strongly to the low elastic modulus/higher roughness materials relative to the higher modulus/smoother materials and that the strength of adhesion is independent of the surface energy of the coatings. Surface roughness may be an important contributor to these differences. The IS700 and IS900 coatings have a roughness on the order of 6 × 10−5 m, which is much greater than the xerogel surfaces with roughness on the order of 10−9–10−10 m (). The rougher IS700 and IS900 coatings may contribute to stronger ‘mechanical locking’ of the secreted adhesives to these surfaces relative to the smoother xerogel surfaces (Swain 1999), which might be more significant than factors such as surface energy in determining the strength of adhesion of diatoms to these surfaces.
Conclusions
Overall, where do xerogel coatings stand with respect to AF and FR performance relative to commercial IS700 and IS900 coatings and the T2 standard? They appear to provide improved performance with respect to microalgal release, but at the present state of technology, xerogels will not compete with the thicker, lower modulus commercial coatings for general applications to minimize biofouling. However, the tunability of the xerogel surfaces may show improved release of barnacles and other hard foulers through control of surface energy, chemical homogeneity/heterogeneity, and roughness in future technological advances. While the production of ‘thick’ xerogel coatings for the release of macrofoulers might be one aim of future research, it could only be a significant improvement if other advantages of the xerogel coatings were not compromised. The xerogel coatings may be particularly useful as AF/FR surfaces in applications where thicker coatings are not optimal or practical. The xerogel coatings of this study, as with other xerogel coatings, are optically transparent (Brinker and Sherer 1990; Avnir 1995; Ingersoll and Bright Citation1997) and have applications as AF/FR coatings where optical transparency is important (marine sensors, underwater cameras, submersible solar panels) (Selvaggio et al. Citation2009). Typically, coatings for these applications are thinner and the xerogels are appropriate materials.
The performance of 1:4:45:50 and 1:14:35:50 C18/TDF/C8/TEOS xerogel coatings in the field with respect to barnacle removal and, after 6 months in the field, physical condition (PDR = 100 for both) indicates that these materials are quite robust. The xerogel coatings may be excellent candidates for deployment where grooming in the field will be used to control the buildup of biofouling and coatings will need to survive the physical demands of grooming (Tribou and Swain Citation2010).
The control of chemical heterogeneities in the xerogel surfaces, surface energies, and roughness as well as the control of the physical characteristics of the coatings (thickness, modulus) all provide means for improving the AF/FR performance of the xerogel surfaces. The path forward is characterized by defining the surface properties and physical characteristics necessary to minimize the settlement and adhesion of both microfoulers and macrofoulers under a variety of field conditions.

gbif_a_690197_sup_26304730.pdf
Download PDF (73.9 KB)Acknowledgements
A.S., F.V.B. and M.R.D. thank the Office of Naval Research for supporting this research through award N0014-09-1-0217. N.C., J.D. and S.J.S. thank the Office of Naval Research for supporting this research through award N00014-11-1-0032. L.H.B. and D.E.W thank the Office of Naval Research for supporting this research through award N00014-09-1-0350. The authors thank Jill Fornalik (Eastman Kodak Co., Rochester, NY, USA) for the AFM experiments described in the Supplementary Information and Daniel Rittschof (Duke University Marine Laboratory, Beaufort, NC, USA) for supplying adult barnacles for the reattachment studies.
References
- Allion , A , Baron , J-P and Boulange-Petrmann , L . 2006 . Impact of surface energy and roughness on cell distribution and viability . Biofouling , 22 : 269 – 278 .
- ASTM D 5618-94 . 1994 . “ Standard test method for measurement of barnacle adhesion strength in shear ” . In ASTM, paint-tests for formulated products and applied coatings 06.01 , West Conshohocken , PA : American Society for Testing and Materials International .
- ASTM D 6990-05 . 2005 . “ Standard practice for evaluating biofouling resistance and physical performance of marine coating systems ” . In ASTM, paint-Tests for formulated products and applied coatings 06.01 , West Conshohocken , PA : American Society for Testing and Materials International .
- Avnir , D . 1995 . Organic chemistry within ceramic matrices: doped sol-gel materials . Acc Chem Res , 28 : 328 – 341 .
- Baier , RE . 1984 . “ Initial events in microbial film formation ” . In Marine biodeterioration: an interdisciplinary study , Edited by: Costlow , JD and Tipper , RC . 57 – 62 . Annapolis , MD : US Naval Institute Press .
- Baier , RE , Shaffin , EG and Zisman , WA . 1968 . Adhesion: mechanisms that assist or impede it . Science , 162 : 1360 – 1368 .
- Barlow , D , Dickinson , GH , Orihuela-Diaz , B , Kulp , J , Rittschof , D and Wahl , K . 2010 . Functional amyloid and bio-adhesion: characterization of amyloid-like nanofibrils composing the adhesive plaque of the barnacle Balanus amphitrite . Langmuir , 26 : 6549 – 6556 .
- Becker K . 1998 . Detachment studies of microfouling in natural biofilms on subtrata with different surface tensions . Int Biodeterior Biodegr , 41 : 93 – 100 .
- Bennett , SM , Finlay , JA , Gunari , N , Wells , DD , Meyer , AE , Walker , GC , Callow , ME , Callow , JA , Bright , FV and Detty , MR . 2010 . The role of surface energy and water wettability in aminoalkyl/fluorocarbon/hydrocarbon-modified xerogel surfaces in the control of marine biofouling . Biofouling , 26 : 235 – 246 .
- Berglin , M and Gatenholm , P . 1999 . The nature of bioadhesive bonding between barnacles and fouling-release silicone coatings . J Adhes Sci Technol , 13 : 713 – 727 .
- Berglin , M , Lönn , N and Gatenholm , P . 2003 . Coating modulus and barnacle bioadhesion . Biofouling , 19 ( Suppl ) : 63 – 69 .
- Berglin , M , Larsson , A , Jonsson , PR and Gatenholm , P . 2001 . The adhesion of the barnacle, Balanus improvisus, to poly (dimethylsiloxane) fouling-release coatings and poly(methyl methacrylate) panels: the effect of barnacle size on strength and failure mode . J Adhes Sci Technol , 15 : 1485 – 1502 .
- Bhushan , B and Jung , YC . 2010 . Natural and biomimetic artificial surfaces for superhydrophobicity, self-cleaning, low adhesion, and drag reduction . Prog Mater Sci , 56 : 1 – 108 .
- Brady , RF and Singer , IL . 2000 . Mechanical factors favoring release from fouling release coatings . Biofouling , 15 : 73 – 81 .
- Brinker , CJ and Scherer , GW . 1990 . Sol-gel science: the physics and chemistry of sol-gel processing , New York , NY : Academic Press. 908 pp .
- Callow , JA , Osborne , MP , Callow , ME , Baker , F and Donald , AM . 2002 . Use of environmental scanning electron microscopy to image the spore adhesive of the marine alga Enteromorpha in its natural hydrated state . Biocolloids Surf B Interfaces , 27 : 315 – 321 .
- Callow , ME and Callow , JA . 2002 . Marine biofouling: a sticky problem . Biologist , 49 : 1 – 5 .
- Cassé , F , Stafslien , SJ , Bahr , JA , Daniels , J , Finlay , JA , Callow , JA and Callow , ME . 2007 . Combinatorial materials research applied to the development of new surface coatings V. Application of a spinning water-jet for the semi-high throughput assessment of the attachment strength of marine fouling algae . Biofouling , 23 : 121 – 130 .
- Cooksey , KE and Wigglesworth-Cooksey , B . 1995 . Adhesion of bacteria and diatoms to surfaces in the sea: a review . Aquat Microb Ecol , 9 : 87 – 96 .
- Dickinson , GH , Vega , IE , Wahl , KJ , Orihuela , B , Beyley , V , Rodriguez , EN , Everett , RK , Bonaventura , J and Rittschof , D . 2009 . Barnacle cement: a polymerization model based on evolutionary concepts . J Exp Biol , 212 : 3499 – 3510 .
- Dobretsov , A and Thomason , JC . 2011 . The development of marine biofilms on two commercial non-biocidal coatings: a comparison between silicone and fluoropolymer technologies . Biofouling , 27 : 869 – 880 .
- Feinberg , AW , Gibson , AL , Wilkerson , WR , Seegert , CA , Wilson , LH , Zhao , LC , Baney , RH , Callow , JA , Callow , ME and Brennan , AB . 2003 . “ Investigating the energetics of bioadhesion on microengineered siloxane elastomers: characterizing the topography, mechanical properties, and surface energy and their effect on cell contact guidance ” . In Synthesis and properties of silicones and silicone-modified materials Edited by: Clarson , SJ , Fitzgerald , JJ , Owen , MF , Smith , SD and van Dyke , ME . Am Chem Soc 838:196–211
- Finlay , JA , Bennett , SM , Brewer , LH , Sokolova , A , Clay , G , Gunari , N , Meyer , AE , Walker , GC , Wendt , DE Callow , ME . 2010 . Barnacle settlement and the adhesion of protein and diatom microfouling to xerogel films with varying surface energy and water wettability . Biofouling , 27 : 657 – 666 .
- Guillard , RRL and Ryther , JH . 1962 . Studies of marine planktonic diatoms. I. Cyclotella nana Hustedt and Detonula confervacea Cleve . Can J Microbiol , 8 : 229 – 239 .
- Gunari , N , Brewer , LH , Bennett , SM , Sokolova , A , Kraut , ND , Finlay , JA , Meyer , AE , Walker , GC , Wendt , DE Callow , ME . 2011 . The control of marine biofouling on xerogel surfaces with nanometer-scale topography . Biofouling , 27 : 137 – 149 .
- Ingersoll , CM and Bright , FV . 1997 . Using sol-gel-based platforms for chemical sensors . Chemtech , 27 : 26 – 35 .
- Kamino , K , Odo , S and Maruyama , T . 1996 . Cement proteins of the acorn barnacle, Megabalanus rosa . Biol Bull , 190 : 403 – 409 .
- Kavanagh , KJ , Quinn , RD and Swain , GW . 2005 . Observations of barnacle detachment from silicones using high-speed video . J Adhesion , 81 : 843 – 868 .
- Kim , J , Chisholm , BJ and Bahr , J . 2007 . Adhesion study of silicone coatings: the interaction of thickness, modulus, and shear rate on adhesion force . Biofouling , 23 : 113 – 120 .
- Majumdar , P , Lee , E , Patel , N , Ward , K , Stafslien , SJ , Daniels , J , Boudjouk , P , Callow , ME , Callow , JA and Thompson , SEM . 2008 . Combinatorial materials research applied to the development of new surface coatings IX: an investigation of novel antifouling/fouling-release coatings containing quaternary ammonium salt groups . Biofouling , 24 : 185 – 200 .
- McMaster , DM , Bennett , SM , Tang , Y , Finlay , JA , Kowalke , GL , Nedved , B , Bright , FV , Callow , ME , Callow , JA Wendt , DE . 2009 . Antifouling character of ‘active’ hybrid xerogel coatings with sequestered catalysts for the activation of hydrogen peroxide . Biofouling , 25 : 21 – 33 .
- Mitik-Dineva , N , Wang , J , Truong , VK , Stoddart , PR , Malherbe , F , Crawford , RJ and Ivanova , EP . 2009 . Differences in colonisation of five marine bacteria on two types of glass surfaces . Biofouling , 25 : 621 – 623 .
- Molino , PJ and Wetherbee , R . 2008 . Mini-review: The biology of biofouling diatoms and their role in the development of microbial slimes . Biofouling , 24 : 365 – 379 .
- Myint , AA , Lee , W , Mun , S , Ahn , CH , Lee , S and Yoon , J . 2010 . Influence of membrane surface properties on the behavior of initial bacterial adhesion and biofilm development onto nanofiltration membranes . Biofouling , 26 : 313 – 321 .
- Newby , BZ , Chaudhury , MK and Brown , HR . 1995 . Macroscopic evidence of the effect of interfacial slippage on adhesion . Science , 269 : 1407 – 1409 .
- Owens , DK and Wendt , RC . 1969 . Estimation of the surface free energy of polymers . J Appl Polymer Sci , 13 : 1741 – 1747 .
- Patent GB1470465 . 1975 . Intersleek 700 finish: Coated marine surfaces. Filed 20 Jan 1975 , International Paint Co Ltd. UK Patent office .
- Patent WO2002074870 . 2002 . Antifouling compositions with a fluorinated alkyl-or alkoxy-containing polymer or oligomer. Filed 18 March 2002 , Akzo Nobel NV. UK Patent office .
- Ramsay , DB , Dickinson , GH , Orihuela , B , Rittschof , D and Wahl , KJ . 2008 . Base plate mechanics of the barnacle Balanus amphitrite (=Amphibalanus amphitrite) . Biofouling , 24 : 109 – 118 .
- Rittschof , D , Orihuela , B , Staflsien , S , Daniels , J , Christianson , D , Chisholm , B and Holm , E . 2008 . Barnacle reattachment: a tool for studying barnacle adhesion . Biofouling , 24 : 1 – 9 .
- Schumacher , JF , Carman , ML , Estes , TG , Feinberg , AW , Wilson , LH , Callow , ME , Callow , JA , Finlay , JA and Brennan , AB . 2007 . Engineered antifouling microtopographies – effect of feature size, geometry, and roughness on settlement of zoospores of the green alga Ulva . Biofouling , 23 : 55 – 62 .
- Selvaggio , P , Tusa , S , Detty , MR , Bright , FV , Ciriminna , R and Pagliaro , M . 2009 . Ecofriendly protection from biofouling of the monitoring system at Pantelleria's Cala Gadir underwater archaeological site, Sicily . Int J Naut Arch , 38 : 417 – 421 .
- Sokolova , A , Bailey , JJ , Waltz , GT , Finlay , JA , Meyer , AE , Fornalik , J , Wendt , DE , Callow , ME , Callow , JA and Bright , FV . 2012 . Spontaneous multiscale phase separation within fluorinated xerogel coatings for fouling-release surfaces . Biofouling , 28 : 143 – 157 .
- Stafslien , S , Daniels , J , Chisholm , B and Christianson , D . 2007a . Combinatorial materials research applied to the development of new surface coatings III. Utilization of a high-throughput multiwell plate screening method to rapidly assess bacterial biofilm retention on antifouling surfaces . Biofouling , 23 : 37 – 44 .
- Stafslien , SJ , Bahr , J , Daniels , J , Christianson , DA and Chisholm , BJ . 2011 . High-throughput screening of fouling-release properties: an overview . J Adhes Sci Technol , 25:2239–2253. DOI:10.11 63/016942411X574934
- Stafslien , SJ , Bahr , JA , Feser , JM , Weisz , JC , Chisholm , BJ , Ready , TE and Boudjouk , P . 2006 . Combinatorial materials research applied to the development of new surface coatings I: a multiwell plate screening method for the high-throughput assessment of bacterial biofilm retention on surfaces. J . Comb Chem , 8 : 156 – 162 .
- Stafslien , SJ , Bahr , JA , Daniels , JW , Vander Wal , L , Nevins , J , Smith , J , Schiele , K and Chisholm , B . 2007b . Combinatorial materials research applied to the development of new surface coatings VI: an automated spinning water jet apparatus for the high-throughput characterization of fouling-release marine coatings . Rev Sci Instrum , 78 : 1 – 6 .
- Sun , Y , Guo , S , Walker , GC , Kavanagh , KJ and Swain , GW . 2004 . Surface elastic modulus of barnacle adhesive and release characteristics from silicone surfaces . Biofouling , 20 : 279 – 289 .
- Swain , G . 1999 . Redefining antifouling coatings . J Protect Coat Lin – Protect Mar Coat (JPCL-PMC) Sept , 1999 : 26 – 35 .
- Swain , GW . 1997 . Field evaluations of non-toxic antifouling coatings: new field technologies and performance criteria . Naval Res Rev , XLIX : 46 – 50 .
- Swain , GW and Schultz , MP . 1996 . The testing and evaluation of non-toxic surfaces . Biofouling , 10 : 187 – 197 .
- Swain , GWJ , Schultz , MP and Vincent , HL . 1994 . “ Shear force measurements of barnacle adhesion for field evaluation of non-toxic foul release surfaces ” . In Recent developments in biofouling control , Edited by: Thompson , M-F , Nagabhushanam , R , Sarojini , R and Fingerman , M . New Delhi (India) : Oxford & IBH Publishing Company. p. 335–344 .
- Tang , Y , Finlay , JA , Kowalke , GL , Meyer , AE , Bright , FV , Callow , ME , Callow , JA , Wendt , DE and Detty , MR . 2005 . Hybrid xerogel films as novel coatings for antifouling and fouling release . Biofouling , 21 : 59 – 71 .
- Tribou , M and Swain , GM . 2010 . The use of proactive in-water grooming to improve the performance of ship hull antifouling coatings . Biofouling , 26 : 47 – 56 .
- Walker , G . 1981 . The adhesion of barnacles . J Adhesion , 12 : 51 – 58 .
- Wilker , JJ . 2010 . The iron-fortified adhesive system of marine mussels . Angew Chem Int Ed , 49 : 8076 – 8078 .