ABSTRACT
Six potato cultivars grown in Turkey in boron-prone areas and differing in their tolerance towards high boron were studied to reveal whether boron causes oxidative stress. To assess stress level, chlorophyll fluorescence and growth parameters were measured. Oxidative damage was assessed as malondialdehyde level, and antioxidant protection was evaluated as ascorbate (AA), dehydroascorbate, reduced glutathione (GSH) and oxidized glutathione amounts and superoxide dismutase, catalase, ascorbate peroxidase (APX) and glutathione reductase (GR) activities. High boron stress affected photosynthesis negatively in a threshold-dependent manner and inhibited growth. No pronounced changes in oxidation of lipids occurred in any cultivar. Activation of APX suggested the involvement of an ascorbic acid–reduced glutathione cycle in the protection against oxidative stress caused by high boron. Efficient work of this antioxidant system was probably hindered by boron complexation with NAD(P)+/NAD(P)H and resulted in the inhibition of GR and a decrease in AA and GSH. Hence, oxidative stress associated with high boron is a secondary component of boron toxicity which arises from metabolic changes caused by boron interference with major metabolites. Potato cultivars tolerate excess boron stress well and show damage only in very high boron concentrations. The potato cvs best suited for high boron soils/breeding purposes are cvs Van Gogh and Agria.
Abbreviations: AA: ascorbic acid; APX: ascorbate peroxidase; CAT: catalase; DHA: dehydroascorbic acid; DHAR: dehydroascorbate reductase; DTNB: 5; 5′-dithiobis-2-nitrobenzoic acid; DTT: dithiotreitol; Fv/Fm: photosynthetic efficiency at the dark-adapted state; GR: glutathione reductase; GSH: reduced glutathione; GSSG: oxidized glutathione; MDA: malondialdehyde; ROS: reactive oxygen species; SOD: superoxide dismutase; TCA: trichloroacetic acid
Introduction
Boron (B) was found to be an essential micronutrient for plant growth and development as late as in 1923 (Warington Citation1923). Since then the interest in boron has been focussed largely on its physiological role in plants. While research has been ongoing for 80 years it was only in the last decade that the first physiological function for boron was defined. Boron is now known to be essential for cell wall structure and function through its role as a stabilizer of the cell wall pectin network and subsequent regulation of cell wall pore size (Brown et al. Citation2002). Other possible functions for boron include a wide variety of cellular events from carbohydrate metabolism to lignification and from membrane integrity to phenolic metabolism (Parr & Loughman Citation1983).
Excessive boron in the soil or in the irrigation water occurs in many dry areas of the world, including Turkey, Iraq, Egypt, Jordan, Syria, Morocco, Chile, California and South Australia, and causes boron toxicity (Torun et al. Citation2001; Landi et al. Citation2012; Ayvaz et al. Citation2013). Turkey is the foremost country in Middle East in potato cultivation and has a 195,000-hectare planting area (FAO Citation2014). Boron toxicity causes stress in potato plants and leads to reduced yield quality and quantity. However, the primary cause and mechanisms of boron toxicity are not understood. It seems probable that boron is involved in one or two key metabolic areas and their impairment leads to a cascade effect with a multitude of toxicity symptoms. It is also known that high boron causes disruption of the cell wall development and many metabolic events by interference with ATP, NADH and NADPH, and disturbs cell division and plant development by forming complexes with ribose (Reid et al. Citation2004).
Boron has a complicated uptake and distribution pattern within a plant that depends on boron concentration and activity of specific transporters, MIPs, which are in turn regulated by transpiration, and on xylem loading (Dannel et al. Citation2002; Landi et al. Citation2013). There are differences also between apoplastic and symplastic boron in different cell types. Under high boron stress the tendency of preferential boron accumulation in leaves as compared to roots has been observed in several studies on boron toxicity (Reid et al. Citation2004). The resultant boron concentration and, hence, the degree of stress, depend on a combination of the above-mentioned factors.
One of the biochemical changes occurring when plants are subjected to environmental stress is the production of reactive oxygen species (ROS) such as superoxide, hydrogen peroxide (H2O2) and hydroxyl radicals, which may also be a secondary effect (Römheld & Marschner Citation1991; Mittler Citation2002). However, our knowledge on oxidative stress caused by boron is limited and somewhat contradictory. Under high boron the outcome of oxidative stress assessed as lipid peroxidation products and/or antioxidant activities has been shown to depend on multiple factors such as plant species, cultivars and even plant organs where oxidative processes are determined and, last but not the least, on the severity of boron stress (Nable et al. Citation1990; Karabal et al. Citation2003; Landi et al. Citation2013). In an extensive pilot study with eight different potato cultivars we have shown that the increase in lipid peroxidation positively correlated with applied boron concentration in only half of the cultivars tested (Ayvaz et al. Citation2013). This prompted a broader study on antioxidative protection of potato plants under boron stress. Our aim was to investigate further the impact of high boron stress on lipid peroxidation and the response of enzymatic and non-enzymatic antioxidants in order to elucidate to what extent oxidative stress is a factor in boron toxicity.
Materials and methods
Plant material and growth conditions
The six potato cultivars in this study were chosen from 22 different certified potato (Solanum tuberosum) cultivars provided by Aegean Agricultural Research Institute. The choice was done according to the tolerance to excess boron as judged by chlorophyll fluorescence data (data not shown). The preliminary data showed a consistent but narrow range of tolerance to boron within 2.5–12.5 mM concentration when assessed through chlorophyll fluorescence. The potato cultivars used in this study were cvs Van Gogh, Resy and Sultan, Agria, Agatha and Granola.
Plants were grown from tubers in quartz sand (0.1–0.6 mm, SP-Minerals LTD, Nilsiän kvartsi, Finland (Chemical composition of the quartz sand: SiO2 (99.4%), Al2O3 (0.2%), Fe2O3 (0.02%) and K2O (0.04%)) in plastic pots (Soparco, code 6820, 16.7 cm × 13.1 cm (diameter × height), 2 L in volume) in a greenhouse and fertilized with Hoagland and Arnon (Citation1950) nutrient solution (containing 0.05 mM boron) altogether for 7 weeks. Plastic trays under the pots prevented leaching of nutrients and boron from the pot. An additional control was included where the plants were initially given the nutrient and boron solutions and watered with distilled water only thereafter to prevent nutrient accumulation in the sand. As no differences in the results were observed between these two controls, only the results of control plants with constant nutrient solution watering are shown. Additional illumination was provided by 400 W high-pressure sodium lamps (Lucalox, LU400/HO/T/40NG) for 16 h day−1 to provide a flux density of 220 μmol m−2 s−1 at the upper leaf canopy. Daytime humidity was 50% and temperature 20°C (day) and 16°C (night).
Boron treatment
Boron was applied 32 days after planting the tubers in six different concentrations (0.5, 2.5, 5, 12.5, 25 and 50 mM, with five replicates each). Plants were harvested at the end of 15 days of excess boron application. Boron concentration was not determined in the potato plants as it has been noticed that there are large variations in boron contents in leaves depending on the position in the leaf, on transpiration rate and on leaching from leaves due to rain or irrigation (Nable et al. Citation1990).
Stress level recording: fresh weight, chlorophyll fluorescence and growth
Chlorophyll fluorescence was measured with an FMS-2-pulse-modulated fluorometer (Hansatech, UK) in the morning. The minimal fluorescence (Fo) was determined by a weak modulated light, and a 0.7 s saturating light of 20,000 μmol m−2 s−1 was used on the dark-adapted leaves (30 min) to determine the maximal fluorescence (Fm), and Fv calculated as Fm−Fo. Maximum quantum efficiency of PSII (Fv/Fm) was determined. Plants were sampled randomly from each treatment group at the harvest days with at least three replicates. The length of the shoots was measured, and shoot and root fresh weights (RFW) were recorded.
Determination of lipid peroxidation
Lipid peroxidation was measured according to Rubin et al. (Citation1976) where the amount of malondialdehyde (MDA), the product of lipid peroxidation, was estimated with the thiobarbituric acid reaction method. Leaves (0.1 g) were homogenized in 3 ml 0.05 M Tris-HCl buffer, pH 7.4. After filtration and centrifugation (20 min, 13,200 rpm) thiobarbituric acid (0.5% [w/v] in 20% [w/v] trichloroacetic acid (TCA) was added to an aliquot of the supernatant and the mixture heated in a boiling water bath (over 90°C) for 30 min. After cooling and centrifugation (20 min, 13,200 rpm) absorbance of the supernatant was measured at 532 nm and corrected for non-specific turbidity by subtracting the absorbance at 600 nm. Thiobarbituric acid 0.5% [w/v] in 20% [w/v] TCA was used as blank. The amount of MDA was calculated per g fresh weight (FW) by using MDA extinction coefficient of 0.156 μM−1 cm−1.
Determination of non-enzymatic antioxidants
Ascorbic acid
Ascorbic acid (AA) and dehydroascorbic acid (DHA) contents were determined according to Knörzer et al. (Citation1996). The absorbance at 525 nm was recorded and total AA and AA contents were calculated on the basis of standard curves (AA in the range of 10–60 μM in 5% metaphosphoric acid (HPO3)). DHA content was calculated by subtracting AA content from total AA content.
Fresh leaves (0.1 g) were homogenized in 2.5 ml 5% HPO3 and centrifuged (30 min, 19000 g) at 4°C. Supernatant was used for the analysis. For determination of AA 125 μl of the sample extract was neutralized with 25 μl 1.5 M triethanolamine. After vigorous mixing, 150 μl sodium phosphate buffer (100 mM, pH 7.4) and 150 μl H2O were added and mixed further.
For determination of total AA 125 μl of the sample extract was neutralized as above, mixed with 150 μl sodium phosphate buffer (100 mM, pH 7.4) and 75 μl of 10 mM dithiotreitol (DTT) and incubated at room temperature for 15 min to reduce the DHA present in the extract. To remove excess DTT, 75 μl of 0.5% (w/v) N-ethylmaleimide was added. Subsequently, the samples were mixed and incubated for at least 30 s at room temperature. After this both samples were treated identically. The following agents were added successively: 300 μl TCA (10% [w/v]), 300 μl phosphoric acid (44% [v/v]), 300 μl 2,2′-dipyridyl (4% [v/v] in 70% ethanol) and 150 μl FeCl3 (3% [w/v]). After mixing, samples were incubated for 1 h at 37°C and absorbance recorded at 525 nm.
Glutathione: Reduced glutathione (GSH) and oxidized glutathione (GSSG) analysis
GSH and GSSG levels were determined according to Knörzer et al. (Citation1996), by an enzymatic recycling procedure in which GSH is sequentially oxidized by 5,5′-dithiobis-2-nitrobenzoic acid (Ellmans Reagent, DTNB) and reduced by NADPH in the presence of glutathione reductase (GR). GSSG can be determined by the reduction of GSSG to GSH, which is then determined by the reaction with DTNB. The extent or rate of 2-nitro-5-thiobenzoic acid formation is monitored at 412 nm and the amount of glutathione present was calculated with a standard curve.
For the determinations, 0.1 g of fresh leaves of three plants in each treatment were homogenized in 2.5 ml, 5% HPO3 and centrifuged (30 min, 19,000g) at 4°C. Supernatants were used for the GSH and GSSG assay. The HPO3 extract was neutralized with 1 M triethanolamine. For GSSG, HPO3 extract was derivatized by 4-vinylpyridine. The assay medium contained 50 mm K-phosphate (pH 7.5), 2.5 mM EDTA, 1 mM DTNB, 0.5 units of GR for GSH (1 unit for GSSG), 0.2 mM NADPH and enzyme extract (20 μl for GSH and 50 μl for GSSG) in a final volume of 1 ml. Increase in absorbance was recorded at 412 nm for 4 min at 25°C.
Protein determination
Protein content of the extracts was determined according to Bradford (Citation1976) using bovine serum albumin as standard.
Assays of antioxidative enzyme activity
Ascorbate peroxidase
Ascorbate peroxidase (APX) (EC 1.11.1.11) activity was determined according to Nakano and Asada (Citation1987) by measuring the decrease in absorbance at 290 nm as AA is oxidized. The reaction solution contained 50 mM K-phosphate buffer (pH 7), 0.1 mM H2O2, 0.5 mM AA and enzyme extract in a final volume of 1 ml. Enzyme activity was calculated from the initial rate of the reaction using molar extinction coefficient of AA (2.8 mM−1 cm−1 at 290 nm).
For the determination, 0.05 g of fresh leaves were homogenized in 1.5 ml of extraction solution containing 50 mM potassium phosphate buffer (pH 7.6), 1 mM Na2-EDTA, 1 mM AA and 0.1% Triton X-100 at 4°C. Homogenates were then filtered and centrifuged (25 min, 13,200 rpm) at 4°C and supernatants used for the assays.
Glutathione reductase
GR (EC 1.6.4.2) activity was determined according to Sgherri et al. (Citation1994) with some modifications. The rate of the decrease in the absorbance of oxidized glutathione (GSSG) was recorded at 340 nm. Corrections were made for any NADPH oxidation in the absence of GSSG. Enzyme activity was calculated from the initial rate of the reaction after subtracting any NADPH oxidation using the molar extinction coefficient of NADPH (6.2 mM−1 cm−1 at 340 nm).
For the determination, 0.05 g fresh leaves were homogenized in 1.5 ml of extraction solution consisting of 100 mM potassium phosphate buffer (pH 7) and 1 mg ml−1 Na2-EDTA. Homogenates were then filtered and centrifuged (25 min, 13,200 rpm) at 4°C and supernatants used for the assay. The assay medium contained 200 mM K-Phosphate (pH 7.5), 0.2 mM Na2-EDTA, 0.5 mM GSSG, 0.2 mM NADPH and leaf extract in a final volume of 1 ml at 20°C. Decrease in absorbance was recorded at 412 nm.
Catalase
Catalase (CAT) (EC 1.11.1.6) activity was determined according to Chance et al. (Citation1979) by measuring the decrease in absorbance at 240 nm in a reaction medium containing 50 mM potassium phosphate buffer (pH 7.2) and 2 mM H2O2. Enzyme activity was calculated from the initial rate of the reaction using the molar extinction coefficient of H2O2 (40 mM−1 cm−1). The same leaf extract as was used for APX activity determination was used here.
Superoxide dismutase
Superoxide dismutase (SOD) (EC 1.15.1.1) activity was determined according to Mc Cord and Fridovich (Citation1969) by the ferricytochrome c assay method using xanthine/xanthine oxidase as the source of superoxide radicals, and one unit of activity was defined as the amount of enzyme required to cause 50% inhibition of the rate of cytochrome c reduction. The same leaf extract as was used for APX activity determination was used here. The assay medium consisted of 0.01 mM cytochrome c and 0.1 mM xanthine, and xanthine oxidase in 50 mM potassium phosphate buffer, pH 7.8, containing 1 mM NaN3and 0.1 mM Na2EDTA, and enzyme extract in a final volume of 1 ml at 25°C and measured for 7 min.
Statistical analysis
The experimental results were expressed as mean ± standard error. Statistical analysis was carried out using analysis of variance (ANOVA) followed by Bonferroni post hoc test (p < 0.05). Statistical package IBM SPSS v.20 was used for data analyses.
Results
Effect of excess boron on growth in potato
Effect of excess boron on growth in potato, assessed as shoot height, S/RFW ( –), revealed a threshold boron concentration, when boron toxicity affected growth parameters negatively: 12.5–25 mM for the S/RFW ( and ) and 25–50 mM for shoot height (). Shoot fresh weight (SFW) was the most affected parameter which showed a significant reduction in response to increasing boron concentrations and exhibited a sharp decrease at 12.5 mM boron (). No significant differences were observed between tolerant and intolerant cultivars, except for RFW in tolerant cv. Resy, which was able to maintain the root biomass throughout all the treatments ().
Figure 1. Shoot height of the potato (S. tuberosum L.) cultivars grown under increasing boron concentrations for 15 days.
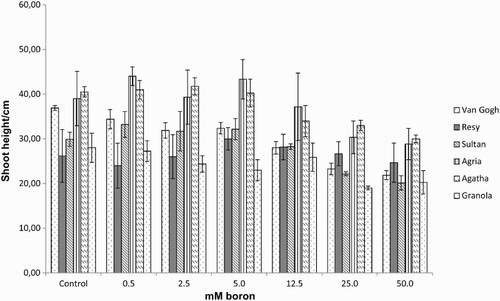
Effect of excess boron on chlorophyll fluorescence
Interestingly, the effect of excess boron on chlorophyll fluorescence () followed the pattern of SFW decline (), with the same sharp decrease at 12.5 mM boron. However, at 12.5 mM of boron in the tolerant cultivars Van Gogh and Resy, a decline in Fv/Fm was either not observed or was much less than in the intolerant cultivars, suggesting efficient photosystem II photochemistry even at this condition. In other cultivars the efficiency of photosynthesis dropped dramatically to ca. 10% of initial level (). Further increase in boron concentration affected negatively the Fv/Fm parameter in all cultivars. To assess the indirect effect of photosynthetic decline on protein content, a determination of soluble proteins was performed. With increasing boron concentrations, total protein content did not change significantly in cvs Van Gogh, Agria and Granola, decreased in cvs Resy and Agatha, and increased in cv. Sultan (data not shown).
Effect of excess boron on lipid peroxidation
One of the consequences of PSII inhibition may be the formation of ROS in the chloroplast, namely singlet oxygen and superoxide anion, which can initiate a cascade of lipid peroxidation reactions. To assess structural integrity of membranes and to elucidate the degree of oxidative stress occurring under our experimental treatments, the effect of excess boron on lipid peroxidation was investigated (). In the intolerant cvs Agria and Granola a decrease in lipid peroxidation was observed at 0.5 and 2.5 mM boron, respectively, suggesting activation of antioxidative processes. A small increase was observed in the tolerant Van Gogh but at a much higher boron concentration (50 mM). In general, there was a trend for diminishing MDA levels in increasing boron concentrations except in the tolerant cultivar Van Gogh.
Effect of excess boron on non-enzymatic antioxidants
To further characterize the oxidative stress component under boron stress, the accumulation of small molecular weight antioxidants AA and glutathione were studied. Total AA and AA had a tendency to decrease under increasing boron concentrations in most cultivars, except the intolerant Agria. However, the decreasing trend was not statistically significant (). In Van Gogh AA levels were stable throughout the treatments (). Interestingly, a relatively high content of oxidized form DHA was detected in all cultivars in both control and experimental plants ().
Figure 6. dehydroascorbic acid, ascorbic acid (Asc.) and total ascorbic acid (Tot.) content of the potato (S. tuberosum L.) cultivars grown under increasing boron concentrations for 15 days. Different letters denote significant differences in comparison to the control, assessed by ANOVA.
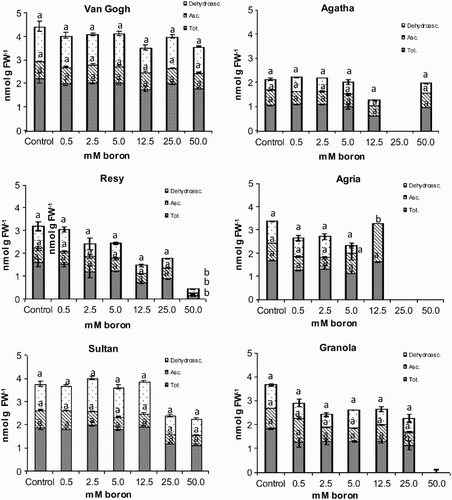
Boron stress did not cause dramatic changes either in total glutathione or in its conjugated form GSSG in most cultivars, except Resy and Agatha, where the total glutathione increased towards the highest boron concentrations (). Similar to the AA pool, the redox state of glutathione was shifted towards the oxidized form GSSG: the ratio of GSSG to GSH was close to one and was not affected by boron treatment. The unusual, more oxidized redox state noticed both in AA/DHA ratio and GSSG to GSH ratio is supported by the fact that it has been determined with two independent methods.
Effect of excess boron on activities of antioxidative enzymes
SOD activity varied among different cultivars depending on boron concentrations and tolerance of cultivar. Tolerant cv. Van Gogh was characterized by the highest SOD activity among all the cultivars under high boron conditions but the changes were not statistically significant (). However, no increase in SOD activity was recorded in response to boron stress with the exception of intolerant cv. Agria, where strong activation was recorded at 0.5 mM boron, the weakest treatment. In Resy, a cultivar of intermediate tolerance according to our data, the induction of SOD was observed starting from 5 mM boron treatment. APX and GR, two essential components of the H2O2-eliminating ascorbate–glutathione cycle (AA–GSH), showed differential response to high boron. Typical of oxidative stress, increase in APX activity was observed in boron-intolerant cultivars (). Interestingly, Van Gogh had low APX activities throughout the boron treatments.
Table 1. SOD, CAT, APX and GR activities of the leaves of the potato (S. tuberosum L.) cultivars grown under increasing boron concentrations for 15 days. Dash denotes leaves that died in the boron treatment and determinations were not made.
Changes in GR activity were inconsistent with boron concentration and cultivar tolerance: e.g. a significant decrease in cv. Agria and an increase in cv. Agatha were observed, both under high boron concentrations (). The activity of another H2O2-detoxifying enzyme, CAT, was significantly reduced by boron stress, especially in boron-tolerant cultivars.
Statistical analysis
An ANOVA test performed for the parameters related to oxidative stress and antioxidative defence revealed that both boron concentration and cultivar were significant factors affecting the parameters of oxidative stress. The output of ANOVA was quite uniform, once again suggesting that oxidative stress is an integral part of boron toxicity and that the experimental design, that is, choice of dependable variables to monitor, was adequate. SOD was the only exception to the rule: boron concentration did not significantly affect this enzyme activity, whereas factor cultivar and interaction of factors cultivar and boron concentration had a significant effect on SOD activity.
Discussion
According to the results, responses to increasing boron concentrations varied among the Turkish potato cvs Van Gogh, Resy, Sultan, Agria, Agatha and Granola. In general, it seems that there is a small concentration range between boron deficiency and toxicity; however, toxicity owing to excess boron is much less common in the environment than boron deficiency (Howe Citation1998; Landi et al. Citation2012). Yet, excessive boron in the soil or in the irrigation water occurs in many dry areas of the world, especially in Turkey, and causes boron toxicity (Torun et al. Citation2001).
Plant species which have been subjected to excessive boron concentrations such as Helianthus annuus L. (Ruiz et al. Citation2003; Keleş et al. Citation2011), Solanum lycopersicum (Gunes et al. Citation1999; Alpaslan & Gunes Citation2001; Cervilla et al. Citation2007, Citation2009; Keleş et al. Citation2011), Cucumis sativus L. (Alpaslan & Gunes Citation2001), Cucumis melo L. (Goldberg et al. Citation2003), Actinidia deliciosa L. (Sotiropoulos et al. Citation2003), Vitis vinifera L. (Gunes et al. Citation2006), Rubus idaeus L. (Neocleous & Vasilakakis Citation2008), Malus domestica Borkh. (Molassiotis et al. Citation2006; Sotiropoulos et al. Citation2006), Zea mays L. (Gunes & Alpaslan Citation2000), Nicotiana tabacum L. (Garcia et al. Citation2001), Citrus nobilis Lour. (Papadakis et al. Citation2004), Hordeum vulgare L. (Karabal et al. Citation2003), Cicer arietinum L. (Ardıc et al. Citation2009), Artemisia annua L. (Aftab et al. Citation2010), Lactuca sativa L. (Eraslan et al. Citation2007) and Citrus grandis L. (Han et al. Citation2009) all have shown that growth and fresh–dry biomass decreased similarly to our experiments ( and ). Interestingly, the most tolerant cultivars exhibited more reduction in growth as compared to the intolerant cvs, which can be an adaptive response.
In accordance with the literature, our results confirmed a negative correlation between photosynthetic efficiency and increasing boron concentrations. Interestingly, the pattern of decline in SFW followed that of photosynthetic performance ( and ). The most profound effect was observed for chlorophyll fluorescence (Fv/Fm) with a sharp decrease at the threshold boron concentration of 12.5 mM. The biomass decrease followed the pattern of chlorophyll fluorescence, and our results showed that in high boron concentrations SFW was negatively affected. Similar results have been found earlier in Solanum esculentum plants by Ferro et al. (Citation2009) and in Ocimum basilicum (Landi et al. Citation2013). A combination of excess boron and salinity applied to R. idaeus (Neocleous & Vasilakakis Citation2008) led to decreased chlorophyll content and fluorescence, while in Citrus × paradisi only chlorophyll fluorescence decreased (Han et al. Citation2009). These observations suggest that assessment of chlorophyll fluorescence is a reliable tool in the estimation of toxic boron levels. In our study the differences in boron tolerance between cultivars, estimated as Fv/Fm, were evident and confirmed by further analyses.
Together with dimished chlorophyll content and photosynthesis, various other physiological effects of boron toxicity in plants have been detected such as decreases in cell divisions in roots (Liu et al. Citation2000; Choi et al. Citation2007), inhibition of shoot and root growth (Lovatt & Bates Citation1984; Nable et al. Citation1990), and reduced stomatal conductance (Lovatt & Bates Citation1984; Aktas et al. Citation2004; Sotiropoulos et al. Citation2006). In our experiments a dramatic decrease in RFW especially in intolerant cvs () took place. In addition, increased lignification and suberinization (Ghanati et al. Citation2002), leakage of substances through the plasma membrane, peroxidation of lipids and antioxidant pathway shifts (Karabal et al. Citation2003) have been observed.
Changes in climatic and edaphic factors in the plant growth environment may disrupt the equilibrium of the cell, and directly or indirectly lead to increased production of ROS. It is well known that oxidative stress caused by ROS leads to increased membrane lipid, nucleic acid and protein degradation which may end up in cell death (Davis Citation1987; Wise & Naylor Citation1987; McKersie & Leshem Citation1994; Imlay & Linn Citation1998; Mittler Citation2002; Blokhina et al. Citation2003; Kawano Citation2003). It has been reported earlier that excessive boron applied to S. lycopersicum (Cervilla et al. Citation2007), to M. domestica rootstocks (Molassiotis et al. Citation2006) and to V. vinifera (Gunes et al. Citation2006) and Triticum aestivum shoots (Karabal et al. Citation2003) resulted in increased lipid peroxidation determined as MDA production, while T. aestivum roots were not significantly affected (Karabal et al. Citation2003). On the other hand, MDA declined in S. esculentum subjected to excess boron (Ferro et al. Citation2009). In our work MDA content was not significantly affected by boron stress except in the highest boron treatment (25 and 50 mM) which was not very pronounced (). These findings are similar to those of Karabal et al. (Citation2003) where MDA in shoots and root tissues of H. vulgare differed, and to our earlier study on lipid peroxidation levels in a variety of potato cultivars (Ayvaz et al. Citation2013). It seems that MDA as a marker of oxidative stress caused by excessive boron may follow the complicated pattern of boron distribution in the plant (Dannel et al. Citation2002; Landi et al. Citation2013). On the whole, the changes in MDA contents were not very large, indicating that lipid peroxidation was not severe in the potato shoots under boron stress ().
Ascorbate is one of the most abundant antioxidants in the apoplast and inside cell compartments. Generally, plants with increased ascorbate concentration have also increased resistance to stress and increased plant growth (Smirnoff & Wheeler Citation2000). The AA–GSH cycle is the main mechanism for ascorbate regeneration against the formation of ROS in plants (Mittler Citation2002). When antioxidant molecules are abundantly present in the chloroplast and other cell compartments, they are effective and form the main defence mechanism against oxidative stress (Noctor & Foyer Citation1998).
In some studies, a close relationship between boron and ascorbate has been established (Blevins & Lukaszewski Citation1998; Brown et al. Citation2002). Cervilla et al. (Citation2007) have found that in S. lycopersicum grown in Hoagland nutrient medium containing 100- and 400-fold boron levels, ascorbate and DHA amount increased, that is, stress caused by boron toxicity led to an increase in ascorbate and ascorbate oxidation (). Also in boron-stressed Citrus sinensis and S. tuberosum cv. Ontario the increase in the amount of oxidants was interpreted as a defence reaction against ROS formation (Mondy & Munshi Citation1993; Keles et al. Citation2004). In contrast, in Citrus × paradisi (Han et al. Citation2009), in S. esculentum (Ferro et al. Citation2009) and in L. sativa L. (Eraslan et al. Citation2007) excessive boron application led to decreased AA amount. In Pyrus comunis leaves AA amount increased to some extent and started to decline with excess boron application (Wang et al. Citation2011) similarly to our study. In our data there was a tendency to decreased total AA and ascorbate pools during the seven-week experiment. Together with the trend observed for GSSG and GSH, the accumulation of DHA may signal the redox shift towards more oxidized state in potato cultivars. This abnormal redox behaviour for green leaves was seen for two different redox-active compounds measured with two different techniques, and therefore most probably reflects the real situation. In this regard, our findings are similar to the results of Han et al. (Citation2009), Ferro et al. (Citation2009), and Wang et al. (Citation2011) obtained with Citrus x paradisi, S. esculentum and Pyrus communis under excessive boron application. Significant accumulation of DHA can also reflect efficient transport of ascorbate in potato, since DHA is considered as the preferred transport form of ascorbate from shoots to roots and tubers, and from the apoplast to the cytoplasmic side of the plasma membrane (Horemans, Asard, et al. Citation1998; Horemans, Potters, et al. Citation1998). Involvement of the apoplast in the response to boron toxicity is interesting, because the main physiological function ascribed to boron so far is the formation of complexes with sugar alcohols in the cell wall (Dannel et al. Citation2002). It has been suggested also that under normal conditions most of dissolved boron is located in the apoplastic space (Matoch Citation1997).
Glutathione (GSH), a multi-functional metabolite in plants, plays an important role in cell defence and protection under oxidative stress conditions. GSH protects thiol groups of proteins from oxidation during stress (Noctor et al. Citation2002). It has been found that GSH synthesis is hindered in H. annuus under boron toxicity conditions. However, external antioxidant application alleviated high boron toxicity (Ruiz et al. Citation2003). In a study by Cervilla et al. (Citation2007) with two S. lycopersicum species exposed to boron stress, GSH amount increased in one, while no significant change was observed in the other. Han et al. (Citation2009) have found that GSH content in C. grandis and in S. esculentum (Ferro et al. Citation2009) correlated negatively with increasing boron concentrations. In Pyrus communis leaves GSH increased to some extent and then started to decline in response to excess boron application (Wang et al. Citation2011). In our study, the highest boron concentrations (12.5, 25 and 50 mM) resulted in significant increases in GSH in cvs. Resy and Agatha (). The redox state of the glutathione pool, although shifted slightly towards the oxidized form, GSSG, was not affected by increasing boron concentrations most probably due to impaired GR activity ().
It has been shown many times before that SOD is induced when ROS levels increase (Mittler Citation2002). In a study with two different barley cultivars grown in toxic boron concentrations, SOD activity in boron-tolerant cultivar did not change significantly. However, in shoot and root cells of the boron-susceptible H. vulgare cv. Hamidiye SOD activity showed a differential response (Karabal et al. Citation2003). A similar trend was observed for two S. lycopersicum cultivars (Cervilla et al. Citation2007). It has been reported also that SOD activity increased in M. domestica rootstocks (Molassiotis et al. Citation2006; Sotiropoulos et al. Citation2006), in V. vinifera leaves (Gunes et al. Citation2006), in C. grandis (Han et al. Citation2009), in A. annua L. (Aftab et al. Citation2010) in L. sativa L. (Eraslan et al. Citation2007) and in H. annuus and S. esculentum (Keles et al. Citation2011) under excessive boron application. According to our findings SOD activity showed a differential response: It was higher in the tolerant cvs Van Gogh and Sultan and lower in the intolerant cvs Agria and Agatha with the exception of Granola. Statistically significant activation was observed in several cultivars but the amplitude was not large. We can conclude that SOD activity generally is not a main factor in antioxidative protection under boron stress. These results are similar to those of Wang et al. (Citation2011) and Keles et al. (Citation2004).
CAT activity has been shown to decrease in excess boron-treated (1–6 mM) M. domestica root buds (Molassiotis et al. Citation2006), in Citrus sinensis leaves (Keles et al. Citation2004), in C. grandis (Han et al. Citation2009) and in S. esculentum (Keles et al. Citation2011). On the other hand, CAT activity increased in excess boron-treated M. domestica root buds (Sotiropoulos et al. Citation2006), in V. vinifera (Gunes et al. Citation2006) and in S. lycopersicum (Cervilla et al. Citation2007). CAT activity levels in leaves of boron-tolerant and -susceptible H. vulgare cultivars did not change significantly but activity in roots of susceptible cultivar increased (Karabal et al. Citation2003). In general, in our study, increasing boron concentration led to decreased CAT activity especially in excessive boron application groups. Such a decrease can be due to CAT compartmentalization predominantly in peroxisomes; if boron causes oxidative stress localized to other cellular compartments (e.g. apoplast), then a decrease in CAT activity is feasible.
APX which eliminates H2O2 in the AA–GSH cycle is one of the antioxidative enzymes against oxidative stress (Asada Citation1999). APX activity in excessive boron-treated S. lycopersicum (Cervilla et al. Citation2007), H. vulgare (Karabal et al. Citation2003), L. sativa (Eraslan et al. Citation2007), C. grandis (Han et al. Citation2009) and C. arietinum (Ardıc et al. Citation2009) increased significantly but it has also been shown to decrease significantly in V. vinifera (Gunes et al. Citation2006) and in S. esculentum (Ferro et al. Citation2009). Recently, in a study with excessive boron-treated Pyrus pyrifolia APX activity increased to some extent (Wang et al. Citation2011). In our investigation all the cultivars differed in their levels from the control APX activity. During boron treatment there was a significant increase in APX activity in all cultivars except Van Gogh (). In general, increasing boron concentration led to increased APX activity in all cultivars especially in excessive boron application groups, which is in line with the above-mentioned findings.
In an earlier study on boron stress with boron-tolerant and -susceptible H. vulgare cultivars, GR activity in leaf and root tissues of boron-tolerant Hordeum cultivar did not change. On the other hand, in the boron-susceptible Hordeum cultivar GR activity increased in leaf tissues, while the opposite trend was observed in roots (Karabal et al. Citation2003). Fluctuations in GR activity have been measured in a number of plant species under boron stress (Keles et al. Citation2004; Cervilla et al. Citation2007; Ardıc et al. Citation2009; Han et al. Citation2009; Ferro et al. Citation2009; Keles et al. Citation2011; Wang et al. Citation2011). In our study GR activity in the control plants of different potato cultivars was in a decreasing range starting from cvs Agria, Resy, Granola, Sultan, Agatha, and ending with cv. Van Gogh with the lowest activity (). GR activity responses to increasing boron concentrations varied insignificantly in cv. Van Gogh, Resy, Agatha and Granola. The main factor affecting GR activity and other antioxidant enzyme activities under high boron stress may be related to boron ability to effectively bind NAD(P)+ and, to a lesser extent NAD(P)H (Reid et al. Citation2004), thus eliminating the substrate from GR-catalysed reduction of GSSH.
Contradictory results on oxidative stress under low and high boron have been observed earlier. A proteomic investigation of Arabidopsis plants subjected to a range of boron concentrations (from deficient to toxic) suggested that photosynthetic/carbohydrate metabolism was negatively affected by high boron (Chen et al. Citation2014). No significant changes were observed in several oxidative stress-related proteins: Cu-Zn SOD, Mn SOD and chloroplast peroxiredoxin; the enzymes which protect from superoxide and peroxides in the cytosol, mitochondria and chloroplast (Chen et al. Citation2014). On the contrary, in a study by Landi et al. (Citation2013), boron toxicity promoted oxidative stress in O. basilicum and resulted in the enhancement of antioxidative enzymatic activity.
In our study we have shown that different potato cultivars vary in their tolerance to excessive boron and this is reflected in oxidative stress damage and antioxidant status of the plants mainly in the highest boron concentrations. As a conclusion it can be stated that under mild boron stress the plants can cope with normal antioxidant levels and no oxidative damage to biological membranes can be recorded. Once the boron levels rise, the antioxidative protection increases only slightly, and once gross toxicity levels are reached, the antioxidative protection cannot be maintained and the plants show strong stress symptoms seen in the decreased FW and photosynthesis level.
Judged by our data on the redox state of the pairs AA/DHA and GSH/GSSG, the intracellular redox state is shifted towards an oxidized state. It is possible that high boron disturbs the redox balance of the cell, rather than causes oxidative stress. However, because of the high sensitivity of ROS-detoxifying machinery to redox changes, this effect of boron is difficult to dissect. GR turnover directly depends on NAD(P)H and inevitably would be affected by the shift in the redox state towards oxidation, that is, the decrease in NAD(P)H/NAD(P)+ ratio. Several findings, such as abrupt decrease in photosynthetic capacity at 12.5 mM boron, discrepancies in enzyme activities and a shift towards oxidation in the redox pairs AA/DHA and GSH/GSSG, suggest that the progression of oxidative stress is not quite straightforward under high boron. One of the main physiological functions for boron, besides interference with the cell wall structure, is the ability to form stable complexes with sugars, including ribose, a constituent of many important metabolites. Therefore, boron can interfere with the availability of NAD+, NADH and, to a lesser extent, NADPH to enzymatic reactions (Reid et al. Citation2004). This ability can be the main reason for the dramatic effect of high boron on photosynthesis and can lie beneath many discrepancies observed in oxidative stress markers (e.g. GR in the current study). It appears that oxidative stress associated with high boron is one of the secondary components of boron toxicity. Oxidative stress rather arises from the metabolic changes caused by boron interference with the major metabolic pathways, including antioxidant protection.
Disclosure statement
No potential conflict of interest was reported by the authors
ORCID
Muavviz Ayvaz http://orcid.org/0000-0002-1776-0730
Kurt V. Fagerstedt http://orcid.org/0000-0002-6839-2958
Additional information
Funding
References
- Aftab T, Khan MMA, Idrees M, Naeem M, Ram M. 2010. Boron induced oxidative stress, antioxidant defence response and changes in artemisinin content in Artemisia annua L. J Agron Crop Sci. 196:423–430. doi: 10.1111/j.1439-037X.2010.00427.x
- Aktaş LY, Gemici M, Türkyılmaz B, Güven A. 2004. Yüksek bor konsantrasyonlarının Triticum durum Desf. cv. Gediz'de büyüme ve protein metabolizması üzerine etkileri. Anadolu J AARI. 14:88–89.
- Alpaslan M, Gunes A. 2001. Interactive effects of boron and salinity on the growth, membrane permeability and mineral composition of tomato and cucumber plants. Plant Soil. 236:123–128. doi: 10.1023/A:1011931831273
- Ardic M, Sekmen AH, Tokur S, Ozdemir F, Turkan I. 2009. Antioxidant responses of chickpea plants subjected to boron toxicity. Plant Biol. 11:328–338. doi: 10.1111/j.1438-8677.2008.00132.x
- Asada K. 1999. The water–water cycle in chloroplasts: scavenging of active oxygen and dissipation of excess photons. Ann Rev Plant Physiol Plant Mol Biol. 50:601–639. doi: 10.1146/annurev.arplant.50.1.601
- Ayvaz M, Avci M, Yamaner C, Koyuncu M, Guven A, Fagerstedt K. 2013. Does excess boron affect the malondialdehyde levels of potato cultivars? EurAsian J Biosci. 7:47–53. doi: 10.5053/ejobios.2013.7.0.6
- Blevins DG, Lukaszewski KM. 1998. Boron in plant structure and function. Ann Rev Plant Physiol Plant Mol Biol. 49:481–500. doi: 10.1146/annurev.arplant.49.1.481
- Blokhina O, Virolainen E, Fagerstedt KV. 2003. Antioxidants, oxidative damage and oxygen deprivation stress: a review. Ann Bot. 91:179–194. doi: 10.1093/aob/mcf118
- Bradford MM. 1976. A rapid and sensitive method for the quantitation of microgram quantities of protein utilizing the principle of protein dye binding. Anal Biochem. 72:248–254. doi: 10.1016/0003-2697(76)90527-3
- Brown PH, Bellaloui N, Wimmer MA, Bassil ES, Ruiz J, Hu H, Pfeffer H, Dannel F, Römheld V. 2002. Boron in plant biology. Plant Biol. 4:205–223. doi: 10.1055/s-2002-25740
- Cervilla LM, Blasco BA, Rios JJ, Romero L, Ruiz JM. 2007. Oxidative stress and antioxidants in tomato (Solanum lycopersicum) plants subjected to boron toxicity. Ann Bot. 100:747–756. doi: 10.1093/aob/mcm156
- Cervilla LM, Blasco B, Rios JJ, Rosales MA, Rubio-Wilhelmi MM, Sanchez-Rodriguez E, Romero L, Ruiz JM. 2009. Response of nitrogen metabolism to boron toxicity in tomato plants. Plant Biol. 11:671–677. doi: 10.1111/j.1438-8677.2008.00167.x
- Chance B, Sies H, Boveris A. 1979. Hydroperoxide metabolism in mammalian organs. Physiol Rev. 59: 527–605.
- Chen M, Mishra S, Heckathorn SA, Jonathan MF, Krause C. 2014. Proteomic analysis of Arabidopsis thaliana leaves in response to acute boron deficiency and toxicity reveals effects on photosynthesis, carbohydrate metabolism, and protein synthesis. J Plant Physiol. 171:235–242. doi: 10.1016/j.jplph.2013.07.008
- Choi EY, Kolesik P, Mcneill A, Collins H, Zhang Q, Huynh BL, Graham R, Stangoulis J. 2007. The mechanism of boron tolerance for maintenance of root growth in barley (Hordeum vulgare L.). Plant Cell Environ. 30:984–993. doi: 10.1111/j.1365-3040.2007.01693.x
- Dannel F, Pfeffer H, Römheld V. 2002. Update on boron in higher plants – uptake, primary translocation and compartmentalisation. Plant Biol. 4:193–204. doi: 10.1055/s-2002-25730
- Davis KJA. 1987. Protein damage and degradation by oxygen radicals, I. General aspects. J Biol Chem. 262:9895–9901.
- Eraslan F, Inal A, Savasturk O, Gunes A. 2007. Changes in antioxidative system and membrane damage of lettuce in response to salinity and boron toxicity. Sci Hortic. 114:5–10. doi: 10.1016/j.scienta.2007.05.002
- [FAO] Food and Agriculture Organization. 2014. Agricultural production statistics. Rome: Food and Agriculture Organization of the United Nations Statistics (FAOSTAT).
- Ferro M, Sgherri C, Romani M, Carvalho MF, Izzo R. 2009. Tomato irrigated with boron enriched fresh water effects on leaves and berries. Agrochimica. 53:52–67.
- Garcia PC, Rivero RM, Lopez-Lefebre LR, Sanchez E, Ruiz JM, Romero L. 2001. Response of oxidative metabolism to the application of carbendazim plus boron in tobacco. Aust J Plant Physiol. 28:801–806.
- Ghanati F, Morita A, Yokota H. 2002. Induction of suberin and increase of lignin content by excess boron in tobacco cells. Soil Sci Plant Nutr. 48:357–364. doi: 10.1080/00380768.2002.10409212
- Goldberg S, Shouse PJ, Lesch SM, Grieve CM, Poss JA, Forster HS, Suarez DL. 2003. Effect of high boron application on boron content and growth of melons. Plant Soil. 256:403–411. doi: 10.1023/A:1026186311974
- Gunes A, Alpaslan M. 2000. Boron uptake and toxicity in maize genotypes in relation to boron and phosphorus supply. J Plant Nutr. 23:541–550. doi: 10.1080/01904160009382038
- Gunes A, Alpaslan M, Cikili Y, Ozcan H. 1999. Effect of zinc on the alleviation of boron toxicity in tomato. J Plant Nutr. 22:1061–1068. doi: 10.1080/01904169909365695
- Gunes A, Soylemezoglu G, Inal A, Bagci EG, Coban S, Sahin O. 2006. Antioxidant and stomatal responses of grapevine (Vitis vinifera L.) to boron toxicity. Sci Hortic. 110:279–284. doi: 10.1016/j.scienta.2006.07.014
- Han S, Tang N, Jiang HX, Yang LT, Li Y, Chen LS. 2009. CO2 assimilation, photosystem II photochemistry, carbohydrate metabolism and antioxidant system of citrus leaves in response to boron stress. Plant Sci. 176:143–153. doi: 10.1016/j.plantsci.2008.10.004
- Hoagland DR, Arnon DI. 1950. The water-culture method for growing plants without soil. Berkeley: California Agricultural Experiment Station – Circular 347.
- Horemans N, Asard H, Van Gestelen P, Caubergs RJ. 1998. Facilitated diffusion drives transport of oxidised ascorbate molecules into purified plasma membrane vesicles of Phaseolus vulgaris. Physiol Plant. 104:783–789. doi: 10.1034/j.1399-3054.1998.1040439.x
- Horemans N, Potters G, Caubergs RJ, Asard H. 1998. Transport of ascorbate into protoplasts of Nicotiana tabacum Bright Yellow-2 cell line. Protoplasma. 205:114–121. doi: 10.1007/BF01279301
- Howe PD. 1998. A review of boron effects in the environment. Biol Trace Elements Res. 66:153–156. doi: 10.1007/BF02783135
- Imlay JA, Linn S. 1998. DNA damage and oxygen radical toxicity. Science 240:1302–1309. doi: 10.1126/science.3287616
- Karabal E, Yücel M, Hüseyin AÖ. 2003. Antioxidant responses of tolerant and sensitive barley cultivars to boron toxicity. Plant Sci. 164:925–933. doi: 10.1016/S0168-9452(03)00067-0
- Kawano T. 2003. Roles of the reactive oxygen species-generating peroxidase reactions in plant defence and growth induction. Plant Cell Rep. 21:829–837.
- Keleş Y, Ergün N, Öncel I. 2011. Antioxidant enzyme activity affected by high boron concentration in sunflower and tomato seedlings. Comm Soil Sci Plant Anal. 42:173–183. doi: 10.1080/00103624.2011.535068
- Keles Y, Oncel I, Yenice N. 2004. Relationship between boron content and antioxidant compounds in Citrus leaves taken from fields with different water source. Plant Soil. 265:345–353. doi: 10.1007/s11104-005-0646-8
- Knörzer C, Durner J, Böger P. 1996. Alterations in the antioxidative system of suspension-cultured soybean cells (Glycine max) induced by oxidative stress. Physiol Plant. 97:388–396. doi: 10.1034/j.1399-3054.1996.970225.x
- Landi M, Degl'Innocenti E, Pardossi A, Guidi L. 2012. Antioxidant and photosynthetic responses in plants under boron toxicity: a review. Am J Agric Biol Sci. 7:255–270. doi: 10.3844/ajabssp.2012.255.270
- Landi M, Pardossi A, Remorini D, Guidi L. 2013. Antioxidant and photosynthetic response of a purple-leaved and a green-leaved cultivar of sweet basil (Ocimum basilicum) to boron excess. Environ Exp Bot. 85:64–75. doi: 10.1016/j.envexpbot.2012.08.008
- Liu D, Jiang W, Zhang L, Li L. 2000. Effects of boron ions on root growth and cell division of broad bean (Vicia faba L.). Israel J Plant Sci. 48:47–41. doi: 10.1560/C74E-VYKD-FKYK-TQWK
- Lovatt CJ, Bates LM. 1984. Early effects of excess boron on photosynthesis and growth of Cucurbita pepo. J Exp Bot. 35:297–305. doi: 10.1093/jxb/35.3.297
- Matoh T. 1997. Boron in plant cell walls. Plant Soil. 193:59–70. doi: 10.1023/A:1004207824251
- McCord JM, Fridovich I. 1969. Superoxide dismutase: an enzymic function for erythrocuprein (hemocuprein). J Biol Chem. 244:6049–6055.
- McKersie BD, Leshem YY. 1994. Stress and stress coping in cultivated plants. Dordrecht: Kluwer Academic Publishers.
- Mittler R. 2002. Oxidative stress, antioxidants and stress tolerance. Trends Plant Sci. 7:405–410. doi: 10.1016/S1360-1385(02)02312-9
- Molassiotis A, Sotiropoulos T, Tanou G, Diamantidis G, Therios I. 2006. Boron induced oxidative damage and antioxidant and nucleolytic responses in shoot tips culture of the apple rootstock EM9 (Malus domestica Borkh). Environ Exp Bot. 56:54–62. doi: 10.1016/j.envexpbot.2005.01.002
- Mondy NI, Munshi CB. 1993. Effect of boron on enzymatic discoloration and phenolic and ascorbic acid content of potatoes. J Agri Food Chem. 41:554–556. doi: 10.1021/jf00028a009
- Nable RO, Paull JG, Cartwrith B. 1990. Problems associated with the use of foliar analysis for diagnosing boron toxicity in barley. Plant Soil. 128:225–232. doi: 10.1007/BF00011113
- Nakano Y, Asada K. 1987. Purification of ascorbate peroxidase in spinach chloroplasts: its inactivation in ascorbate depleted medium and reactivation by monodehydroascorbate radical. Plant Cell Physiol. 28:131–140.
- Neocleous D, Vasilakakis M. 2008. Effects of boron and salinity on red raspberry in vitro. Int J Fruit Sci. 8:216–225. doi: 10.1080/15538360802529807
- Noctor G, Foyer CH. 1998. Ascorbate and glutathione: keeping active oxygen under control. Ann Rev Plant Physiol Plant Mol Biol. 49:249–279. doi: 10.1146/annurev.arplant.49.1.249
- Noctor G, Gomez L, Vanacker H, Foyer CH. 2002. Interactions between biosynthesis, compartmentation and transport in the control of glutathione homeostasis and signalling. J Exp Bot. 53:1283–1304. doi: 10.1093/jexbot/53.372.1283
- Papadakis IE, Dimassi KN, Bosabadilis AM, Therios IN, Patakas A, Giannakoula A. 2004. Boron toxicity in “Clementine” mandarin plants grafted on two rootstocks. Plant Sci. 166:539–547. doi: 10.1016/j.plantsci.2003.10.027
- Parr AJ, Loughman BC. 1983. Boron and membrane function in plants. In: Robb DA, Pierpoint WS, editors. Metals and micronutrients, uptake and utilisation by plants. New York (NY): Academic Press; p. 87–108.
- Reid RJ, Hayes JE, Post A, Stangoulis JCR, Graham RD. 2004. A critical analysis of the causes of boron toxicity in plants. Plant Cell Environ. 25:1405–1414. doi: 10.1111/j.1365-3040.2004.01243.x
- Römheld V, Marschner H. 1991. Functions of micronutrients in plants. In: Mordvedt, JJ, Cox FR, Schuman LM, Welch RM, editors. Micronutrients in agriculture. 2nd ed. Book Series No. 4. Madison (WI): Soil Science Society of America; p. 297–328.
- Rubin BA, Merzlyak MN, Juferova SG. 1976. Oxidation of lipid components in isolated chloroplasts under lighting: the substrates and products of lipid peroxidation. Fiziol Rast. 23:254–261.
- Ruiz JM, Rivero RM, Romero L. 2003. Preliminary studies on the involvement of biosynthesis of cysteine and glutathione in the resistance to boron toxicity in sunflower plants. Plant Sci. 165:811–817. doi: 10.1016/S0168-9452(03)00276-0
- Sgherri CLM, Loggini B, Puliga S, Navari-Izzo F. 1994. Antioxidant system in Sporobolus stapfianus: changes in response to desiccation and rehydration. Phytochemistry. 35:561–565. doi: 10.1016/S0031-9422(00)90561-2
- Smirnoff N, Wheeler GL. 2000. Ascorbic acid in plants: biosynthesis and function. Crit Rev Plant Sci. 19:267–290. doi: 10.1016/S0735-2689(00)80005-2
- Sotiropoulos TE, Molassiotis A, Almaliotis D, Mouhtaridou G, Dimassi K, Therios I, Diamantidis G. 2006. Growth, nutritional status, chlorophyll content, and antioxidant responses of the apple rootstock MM 111 shoots cultured under high boron concentrations in vitro. J Plant Nutr. 29:575–583. doi: 10.1080/01904160500526956
- Sotiropoulos TE, Therios IN, Dimassi KN. 2003. Boron toxicity in kiwifruit plants (Actinidia deliciosa), treated with nitrate, ammonium, and a mixture of both. J Plant Nutr Soil Sci. 166:529–532. doi: 10.1002/jpln.200320264
- Torun A, Gultekin I, Kalayci M, Yilmaz A, Eker S, Cakmak I. 2001. Effects of zinc fertilization on grain yield and concentrations of zinc, boron and toxic-boron soil. J Plant Nutr. 24:1817–1829. doi: 10.1081/PLN-100107314
- Wang JZ, Tao ST, Qi KJ, Wu J, Wu HQ, Zhang SL. 2011. Changes in photosynthetic properties and antioxidative system of pear leaves to boron toxicity. Afr J Biotechnol. 10:19693–19700.
- Warington K. 1923. The effect of boric acid and borax on the broad bean and certain other plants. Ann Bot. 37:629–672.
- Wise RR, Naylor AW. 1987. Chilling-enhanced photooxidation: evidence for the role of singlet oxygen and superoxide in the breakdown of pigments and endogenous antioxidants. Plant Physiol. 83:278–282. doi: 10.1104/pp.83.2.278