Abstract
(E)-4-hydroxy-3-methylbut-2-en-1-yl diphosphate (HMBPP) is an intermediate of the methylerythritol phosphate pathway. Utilization of HMBPP by lycopene elongase from Corynebacterium glutamicum, which is a UbiA-family prenyltransferase responsible for C50 carotenoid biosynthesis, was investigated using an Escherichia coli strain that contained the exogenous mevalonate pathway as well as the carotenoid biosynthetic pathway. Inhibition of the endogenous methylerythritol phosphate pathway resulted in loss of the production of C50 carotenoid flavuxanthin, while C40 lycopene formation was retained. Overexpression of E. coli ispH gene, which encodes HMBPP reductase, also decreased the production of flavuxanthin in E. coli cells. These results indicate the preference of lycopene elongase for HMBPP instead of the previously proposed substrate, dimethylallyl diphosphate. Furthermore, several (all-E)-prenyl diphosphate synthases, which are classified in a distinct family of prenyltransferase, were demonstrated to accept HMBPP, which implies that the compound is more widely used as a prenyl donor substrate than was previously expected.
Graphical abstract
The reaction of Corynebacterium glutamicum lycopene elongase CrtEb.

C50 carotenoids have been discovered in various organisms such as part of the bacteria of class Actinobacteria and most of the archaea of class Halobacteria [Citation1].Their structures are varied and could be either cyclic or acyclic, but they generally have hydroxyl groups at both ends of a molecule, as with hydroxylated C40 carotenoids such as zeaxanthin and lutein. The biosynthetic routes of the C50 carotenoids are similar (Figure ): (1) Phytoene, an acyclic, colorless C40 precursor of carotenoid, is formed from two molecules of geranylgeranyl diphosphate, and then is desaturated to form a reddish C40 carotenoid, lycopene. (2) Two C5 isoprene units are condensed with lycopene at its terminal double bonds to form a C50 skeleton. (3) Cyclization occurs, if needed, at the termini of the lycopene moiety, which does not include newly added C5 units. The ways hydroxyl groups are acquired, however, differ among organisms. Bacterioruberin, an acyclic C50 carotenoid from halophilic archaea such as Halobacterium salinarum and Haloarcula japanica, possesses four hydroxyl groups at its 1, 1′, 3″, and 3″′ positions. The two at the 1 and 1′ positions are acquired when C5 dimethylallyl groups are transferred from dimethylallyl diphosphate (DMAPP) to the C2 and C2′ of lycopene by the action of the UbiA-family lycopene elongase Lye, which suggests that hydroxylation is the result of carbocation quenching by water [Citation2,3]. The hydroxyl groups at the 3″ and 3″′ positions are added by the action of a homolog of CruF-type carotenoid 1,2-hydratase to the transferred dimethylallyl groups. In contrast, bacterial C50 carotenoids generally have a hydroxyl group at the ω-methyl group of each of the transferred C5 isoprene units, rather than at the termini of C40 skeletons, which are often cyclized. In other words, C50 carotenoids such as decaprenoxanthin, sarcinaxanthin, and C.p. 450 produced by Corynebacterium glutamicum, Micrococcus luteus, and Dietzia sp. CQ4, respectively, possess 4-hydroxy-3-methylbut-2-enyl groups [Citation4–6]. The acquisition of hydroxyl groups is thought to be catalyzed by lycopene elongase after the transfer of dimethylallyl groups from DMAPP, because the recombinant expression of C. glutamicum lycopene elongase CrtEb in a lycopene-producing Escherichia coli strain results in the formation of monohydroxylated C45 acyclic carotenoid nonaflavuxantion and dihydroxylated C50 acyclic carotenoid flavuxanthin, both of which are the intermediates of decaprenoxanthin biosynthesis [Citation6,7]. This UbiA-family prenyltransferase, however, obviously has no homology to known hydroxylases that would specifically act toward the ω-methyl group of acyclic isoprenoids, such as geraniol 8-hydroxylase [Citation8,9], farnesol ω-hydroxylase [Citation10], and methyl-branched lipid ω-hydroxylase [Citation11]. Given these findings and the fact that some bacterial C50 carotenoid producers such as C. glutamicum and M. luteus possess the methylerythritol phosphate (MEP) pathway, we devised the simple idea that the prenyl donor substrate used by bacterial lycopene elongases is (E)-4-hydroxy-3-methylbut-2-en-1-yl diphosphate (HMBPP), an intermediate of the MEP pathway, rather than DMAPP.
Figure 1. Biosynthetic pathways of C50 carotenoids in C. glutamicum and H. salinarum. The prenyltransfer reactions catalyzed by lycopene elongases CrtEb and Lye are indicated by the broad arrows.
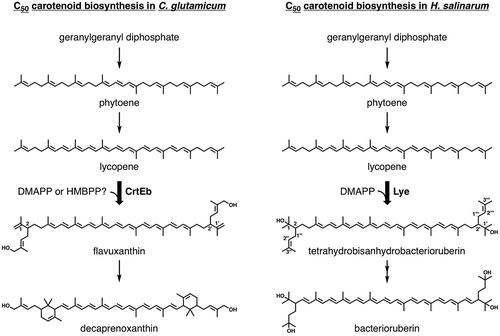
Figure 2. Analyses of carotenoids produced in recombinant E. coli. (A) Normal-phase TLC analysis of carotenoids extracted from Top10/pBAD-crtEbEBI. ori., origin; s.f., solvent front. (B) FAB-MS analysis of the relatively hydrophilic [Rf = ~0.6 in (A)] carotenoid recovered from a TLC plate. (C) HPLC elution profile at the A472 of carotenoids extracted from E. coli Top10/pBAD-crtEbEBI/pJBEI-2999 cultivated in the absence (solid line) and presence (dotted line) of FR-900098. (D) UV–visible spectra of the carotenoid eluted at ~8 min in (C) measured by the PDA detector attached to HPLC.
![Figure 2. Analyses of carotenoids produced in recombinant E. coli. (A) Normal-phase TLC analysis of carotenoids extracted from Top10/pBAD-crtEbEBI. ori., origin; s.f., solvent front. (B) FAB-MS analysis of the relatively hydrophilic [Rf = ~0.6 in (A)] carotenoid recovered from a TLC plate. (C) HPLC elution profile at the A472 of carotenoids extracted from E. coli Top10/pBAD-crtEbEBI/pJBEI-2999 cultivated in the absence (solid line) and presence (dotted line) of FR-900098. (D) UV–visible spectra of the carotenoid eluted at ~8 min in (C) measured by the PDA detector attached to HPLC.](/cms/asset/c6cd1c05-79bc-4271-bdae-7454be9c83ee/tbbb_a_1398064_f0002_oc.gif)
Figure 3. The effect of the overexpresson of HMBPP reductase on flavuxanthin formation. Shown are the HPLC elution profiles at the A472 of carotenoids extracted from E. coli Top10/pBAD-crtEbEBI (A) and Top10/pBAD-crtEbEBI-ispH (B).
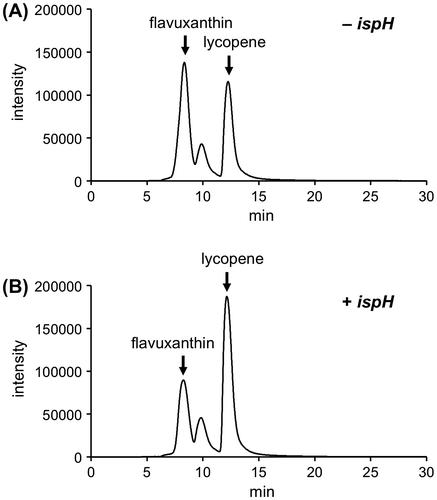
Figure 5. Analyses of the products of (all-E)-prenyl diphosphate synthases (A and B). Radio-TLC analysis of the hydrolyzed products that are synthesized from DMAPP (A) and HMBPP (B). After enzyme reaction, radiolabeled products, i.e. (all-E)-prenyl diphosphates in (A) and probably ω-hydroxylated prenyl diphosphates in (B), were extracted with 1-butanol, and then hydrolyzed by acid phosphatase. Resultant alcohols were analyzed by reversed-phase TLC. ori., origin; s.f., solvent front. (C and D) Ion profiles from the negative-ESI-MS (C) and MS/MS (D) analyses of the products of S. acidocaldarius GGPS from HMBPP. Ions with asterisks were also detected in the analysis of a control sample from the reaction using DMAPP instead of HMBPP. The hypothetical structures of the molecular ion and fragment ions were represented.
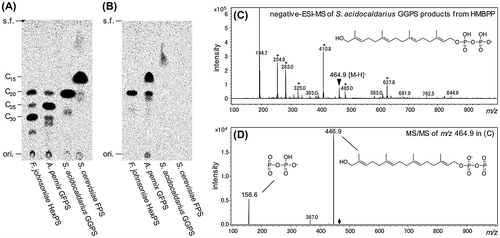
In this study, we intended to elucidate the prenyl donor substrate of lycopene elongase from C. glutamicum, by constructing a flavuxanthin-producing E. coli strain that also possesses the exogenous mevalonate (MVA) pathway, which enabled the inhibition of the endogenous MEP pathway. In addition, the effect of the overexpression of HMBPP reductase on carotenogenesis was investigated. We also evaluated the ability of HMBPP to act as a substrate for (all-E)-prenyl diphosphate synthases, which utilize DMAPP as a proper substrate and are known to catalyze the carbocation-involving prenyltransfer reaction.
Materials and methods
Cultivation of micro organisms and preparation of genome DNA
C. glutamicum was provided by Dr Takaaki Kojima, Nagoya University, and was cultivated in a 5 mL of medium containing 5 g/L polypeptone, 2 g/L NaCl, 10 g/L glucose, 1 g/L K2HPO4, 200 mg/L Mg2SO4·7H2O, and 3 g/L beef extract, at 30 °C overnight prior to being harvested. Lactobacillus brevis was provided by the RIKEN BRC through the Natural Bio-Resource Project of the MEXT, Japan, and was cultivated in a 5 mL of MRS medium containing 5 g/L Bacto Peptone (Difco), 1 g/L beef extract, 5 g/L yeast extract, 20 g/L glucose, 1 g/L Tween 80, 2 g/L K2HPO4, 5 g/L CH3COONa, 2 g/L ammonium citrate, 200 mg/L Mg2SO4·7H2O, and 50 mg/L MnSO4·5H2O, at 30 °C overnight prior to being harvested. Flavobacterium johnsoniae was cultivated as described elsewhere [Citation12]. The E. coli Top10 strain was cultivated in a LB medium. Genome DNA was extracted from the micro-organisms using a Cica Geneus® DNA extraction reagent (Kanto Chemical, Japan). Genome DNA of Saccharomyces cerevisiae was extracted as previously reported [Citation13].
Construction of plasmids for C50 carotenoid production
The gene of C. glutamicum lycopene elongase, crtEb, was amplified from C. glutamicum genome using KOD plus DNA polymerase (Toyobo, Japan) and origonucleotide primers 5′-attcgagctcggtacaaggagatatattatgatggaaaaaataagactaatt-3′ and 5′-aggatccccgggtaccttatatctgatgaattgctattagc-3′. The amplified DNA fragment was inserted into a KpnI-treated pBAD18 plasmid using an InFusion HD Cloning Kit (TaKaRa Bio, Japan) to construct pBAD-crtEb. Then the region containing carotenogenic genes from Pantoea ananatis, i.e. crtE (geranylgeranyl diphosphate synthase gene), crtB (phytoene synthase gene), and crtI (phytoene desaturase gene), was amplified from the plasmid pACYC-IBE [Citation14] using primers 5′-ttcatcagatataagttataaggacagcccgaatgac-3′ and 5′-ctagaggatccccggtacctagagcgggcgctgc-3′. The amplified fragment was inserted in a KpnI-treated pBAD-crtEb using an InFusion HD Cloning Kit to construct pBAD-crtEbEIB.
The E. coli ispH gene encoding HMBPP reductase was amplified from E. coli genome using origonucleotide primers 5′-cagcgcccgctctaggaaggaagattataatgcagatcctgttggcc-3′ and 5′-tctagaggatccccggtaccttaatcgacttcacgaatatcgac-3′. The amplified fragment was inserted in a KpnI-treated pBAD-crtEbEIB using an InFusion HD Cloning Kit to construct pBAD-crtEbEIB-ispH.
Extraction of carotenoids produced in E. coli
The E. coli Top10 strain was transformed using the pBAD-crtEbEIB plasmid. The Top10/pBAD-crtEbEIB cells were cultivated overnight at 37 °C in 1 L of a LB medium supplemented with 100 mg/L ampicillin and 0.02% L-arabinose. Harvested cells were dissolved with 10 mL of methanol/0.3% NaCl (9:1) solution and disrupted by sonication. Lipid containing carotenoids was extracted from the solution with 10 mL n-pentane. The solvent was evaporated under a N2 stream, and the residual was dissolved with 10 mL 2-propanol. The sample was analyzed by normal-phase TLC using a silica gel 60 F254 plate (Merck Millipore). Carotenoid extraction from Top10/pBAD-crtEbEIB-ispH cells was performed with the same procedure.
FAB-MS analysis of C50 carotenoid
The solution of carotenoid extracted by n-pentane from Top10/pBAD-crtEbEIB was concentrated under a N2 stream and dissolved with an excess volume of acetone. The acetone-soluble fraction was spotted on a normal-phase TLC using a partisil LK6 plate (Whatmann), and the plate was developed with hexane/acetone (2:1). An orange-colored spot with a Rf of ~0.5 was scraped from the plate, and the carotenoid that it contained was eluted with acetone. After the solvent was removed by evaporation, the residual was subjected to fast-atom bombardment (FAB)-MS analysis, which was performed with a MStation JMS-700 mass spectrometry system (JEOL, Japan) using a 6 keV Xe beam at a 10 kV accelerating voltage. The positive FAB-MS spectrum was obtained using 3-nitrobenzylalcohol as a matrix.
HPLC analysis of carotenoids
One hundred μL of the carotenoid sample dissolved in 2-propanol was analyzed with a Shimadzu Prominence HPLC system equipped with a SPD-20A UV–visible detector and a SPD-M20A photodiode array (PDA) detector. A COSMOSIL 5C18-PAQ column (4.6 ID × 250 mm, Nacalai Tesque, Japan) was connected, and samples were eluted with an isocratic methanol/2-propanol (1:1) mixture with flow rate of 0.5 mL/min.
Carotenoid production under the MEP pathway inhibition
Top10/pBAD-crtEbEIB was transformed using a pJBEI-2999 plasmid [Citation15], which contained MVA pathway-related genes from S. cerevisiae and was purchased from Addgene, MA. The cells were cultivated at 37 °C in 100 mL of a LB medium supplemented with 100 mg/L ampicillin, 30 mg/L chloramphenicol, 2 g/L lithium acetate, and 0.02% arabinose. When OD600 reached ~1.0, 5 mg of FR-900098 monosodium salt (Sigma–Aldrich) was added into the medium, and the cells were harvested after an additional 24 h of cultivation.
Construction of plasmids for the expression of (all-E)-prenyl diphosphate synthases
The gene of a hypothetical (all-E)-geranylgeranyl diphosphate synthase (GGPS) from F. johnsoniae, fjoh_1263, was amplified from the F. johnsoniae genome using oligonucleotide primers 5′-ggtgccgcgcggcagccatatgcacgatattagccagtacc-3′ and 5′-cagccggatcctcgagcatacttaaacttttctacccattaaattttc-3′. The gene of C. glutamicum GGPS, idsA [Citation16], was amplified from the C. glutamicum genome using oligonucleotide primers 5′-ggtgccgcgcggcagccatatgaaggacgtctccttgagc-3′ and 5′-cagccggatcctcgagcatactacatccgacgttcggttg-3′. The gene of (all-E)-farnesyl diphosphate synthase (FPS) from L. brevis [Citation17], lvis_0975, was amplified from the L. brevis genome using oligonucleotide primers 5′-ggtgccgcgcggcagccatatgccgattaatgcacgg-3′ and 5′-cagccggatcctcgagcatacttagttcacccgttccgtatc-3′. The gene of S. cerevisiae FPS, erg20 [Citation18], was amplified from the S. cerevisiae genome using oligonucleotide primers 5′-ggtgccgcgcggcagccatatggcttcagaaaaagaaattagg-3′ and 5′-cagccggatcctcgagcatacctatttgcttctcttgtaaactttg-3′. By electroporation, the NdeI-treated pET-15b plasmid (Novagen) and each of the amplified DNA fragments were simultaneously introduced into the E. coli AQ3625 strain, which was provided by the National BioResource Project and enabled in vivo E. coli cloning (iVEC, https://shigen.nig.ac.jp/ecoli/strain/). A portion of the colonies selected with ampicillin-containing plates contained plasmids with each gene appropriately inserted. The plasmids for the expression of F. johnsoniae hypothetical GGPS, C. glutamicum GGPS, L. brevis FPS, and S. cerevisiae FPS were designated as pET15b-fjggps, pET15b-cgggps, pET15b-lbfps, and pET15b-scfps, respectively.
Recombinant expression and purification of (all-E)-prenyl diphosphate synthases
Each of the plasmids for the expression of (all-E)-prenyl diphosphate synthases were introduced into the E. coli BL21(DE3) strain. The transformed E. coli cells were cultivated at 37 °C in a 200 mL LB medium supplemented with 100 mg/L ampicillin. When OD600 reached 0.4, 1 mM IPTG was added for induction. Then, the cells were cultivated overnight at 20 °C. The enzymes from the harvested cells was purified using a 1 mL HisTrap FF crude affinity column (GE Healthcare) following the manufacturer’s protocol, using 20 and 500 mM imidazole for the binding and elution buffers, respectively. Recombinant expression and purification of Geobacillus stearothermophilus FPS, Sulfolobus acidocaldarius GGPS, and Aeropyrus pernix (all-E)-geranylfarnesyl diphosphate synthase (GFPS) were performed as described elsewhere [Citation19–21].
Assay of (all-E)-prenyl diphosphate synthases
Each of the purified enzymes was reacted with 5 nmol DMAPP or HMBPP (Sigma–Aldrich) and 5 nmol [1–14C]IPP (2.2 Ci/mol, American Radiolabeled Chemicals Inc.) for 1 h, in a 200 μL volume of reaction mixture containing 250 μmol MgCl2 and 20 μmol of a Tris-HCl buffer, pH8.5, for G. stearothermophilus FPS, 20 μmol of a MES-NaOH buffer, pH5.8, for S. acidocaldarius GGPS, or a MOPS-MaOH buffer, pH7.0, for the other enzymes. The reaction temperature was 55 °C for G. stearothermophilus FPS, S. acidocaldarius GGPS, and A. pernix GFPS, and 30 °C for the other enzymes. The amounts of the enzymes were controlled so that no more than 20% of the substrates reacted. After the reaction, 200 μL of saturated saline and 600 μL of 1-butanol saturated with saline were added, and the reaction products were extracted into the 1-butanol layer. Radioactivity in an aliquot of the 1-butanol layer was measured with an LSC-5100 liquid scintillation counter (Aloka, Japan). The radioactivity extracted in the butanol layer was used to calculate the specific activity of the (all-E)-prenyl diphosphate synthases, which is expressed by an unusual unit: nmol IPP min−1 mg protein.
For the kinetic analysis of S. acidocaldarius GGPS, 33 ng of the enzyme was used for the reaction with 1–20 μM DMAPP against 25 μM IPP; 330 ng of the enzyme was used for the reaction with 5–80 μM HMBPP against 25 μM IPP; 6.3 ng of the enzyme was used for the reaction with 1–20 μM IPP against 25 μM DMAPP; and, 33 ng of the enzyme was used for the reaction with 1–20 μM IPP against 25 μM HMBPP. The enzyme amounts were set to assure that no more than 20% of the substrates would react. Kinetic parameters were calculated using a KaleidaGraph software (Synergy software) by fitting the Michaelis-Menten equation to the data.
Analysis of the products of (all-E)-prenyl diphosphate synthases
For the radio-TLC analysis of the reaction products, 0.2 nmol [1–14C]IPP and either 0.2 nmol DMAPP or HMBPP were used for the reaction. Other conditions were the same as those used in the enzyme assay to obtain specific activity. When significant radioactivity was extracted with 1-butanol, the reaction products in the butanol layer were hydrolyzed with potato acid phosphatase (Sigma–Aldrich) using a method developed by Fujii et al. [Citation22]. The resultant alcohols were analyzed by reversed-phase TLC using a precoated RP18 plate (Merck Millipore) developed with acetone/water (9:1).
The products of S. acidocaldarius GGPS were analyzed using an Esquire 3000 electron spray ionization (ESI)-ion trap MS (Bruker Daltonics) by direct infusion. The butanol-extracted products from the reaction of 3.3 μg S. acidocaldarius GGPS with 10 nmol non-labeled IPP and either 10 nmol DMAPP or HMBPP were evaporated under a N2 stream and then dissolved with acetonitrile/100 mM NH4CO3 (3:1). The samples were injected with a flow rate of 180 μL/h and analyzed using the parameters below: analysis mode, negative; sheathe gas, N2 (10 psi); dry gas, N2 (5.0 L min−1, 300 °C); scan range, 100–1500 m/z; scan speed, 13,000 m/z s−1; ion charge control target, 20,000; and, maximum accumulation time, 200 ms.
Results
Prenyl donor substrate used by lycopene elongase
The E. coli strain producing a C50 carotenoid, flavuxanthin, was constructed by introducing crtEb, the gene of lycopene elongase from C. glutamicum, into E. coli with other carotenogenic genes, which was sufficient for lycopene production. For this purpose, the C. glutamicum crtEb gene was first inserted into the pBAD18 vector to construct pBAD-crtEb. Then, carotenogenic genes from P. ananatis, crtE, crtB, and crtI, encoding geranylgeranyl diphosphate synthase, phytoene synthase, and phytoene desaturase, respectively, were inserted into pBAD-crtEb to construct pBAD-crtEbEBI. The carotenoids produced in E. coli harboring the plasmid were separated by normal-phase TLC (Figure (a)). Two major spots, which were colored orange and thus expected to be derived from carotenoids, were observed. One of the spots co-migrated with the spot of authentic lycopene, with a Rf of ~0.95. In contrast, the other one had a Rf of ~0.63, which suggested a more hydrophilic property. Thus, a corresponding spot was scraped from a TLC plate, on which a larger amount of the sample had been loaded, in order to recover the probable carotenoid compound via elution with acetone. The recovered compound was analyzed via FAB-MS and gave a positive ion with an m/z of 705, which corresponded well with the [M + H]+ ion of a dihydroxylated C50 carotenoid, flavuxanthin, which was the expected product of C. glutamicum lycopene elongase (Figure (b)).
To investigate the substrate preference of lycopene elongase, we constructed an E. coli strain in which both the C50 carotenoid biosynthetic pathway and the MVA pathway were introduced. The strain Top10/pBAD-crtEbEBI/pJBEI-2999 allowed us to inhibit the endogenous MEP pathway using a drug without killing the microorganism, to evaluate the effect of the depletion of HMBPP on carotenogenesis. The carotenoids produced in the strain, which were grown in a medium supplied with lithium acetoacetate to enhance isoprenoid biosynthesis via the MVA pathway, were analyzed via HPLC equipped with UV–visible and PDA detectors. The elution profile of the sample at 472 nm gave two major peaks and one minor peak (Figure (c)). One of the major peaks eluted at ~8 min, which corresponded with the elution time of flavuxanthin recovered from TLC, and the other eluted at ~12 min, which corresponded with the peak of authentic lycopene. The UV–visible spectrum of the compound eluted at ~8 min, which was measured by the PDA detector, had maximum absorption peaks at 469 and 500 nm, which was in good agreement with the reported spectrum of flavuxanthin [Citation5] (Figure (d)). The minor peak that eluted at ~10 min remains unidentified, but was likely derived from a C45 carotenoid, nonaflavuxanthin, which is an intermediate of C50 carotenoid biosynthesis. Inhibition of the MEP pathway was conducted using an inhibitor of 1-deoxy-d-xylulose 5-phosphate reductoisomerase, FR-900098 [Citation23]. To avoid significant growth inhibition, the inhibitor was added when OD600 reached ~1.0, which enabled the same level of growth as that in the absence of the inhibitor. The elution profile of the carotenoids from the cells demonstrated that the inhibition of the MEP pathway caused nearly a complete loss of flavuxanthin, in contrast with the retention of about half of the lycopene production. A slight amount of flavuxanthin still formed, which could have accumulated prior to inhibition because a slight red coloration of the cells was observed when the inhibition began. There was no production of new carotenoids due to the inhibition. The selective decrease in flavuxanthin strongly suggested that HMBPP, which would be depleted by the inhibition of the MEP pathway, was utilized as the prenyl donor substrate by C. glutamicum lycopene elongase.
At that point, however, we could not exclude the possibility that the MEP-pathway inhibitor FR-900098 also inhibits lycopene elongase. To clarify this problem, in vitro assay of lycopene elongase was performed using a crude extract from the E. coli Top10/pBAD-crtEb strain, but the production of C50 (or C45) carotenoids from lycopene was not detected when either HMBPP or DMAPP was used as the prenyl donor substrate (data not shown). We also conducted NMR analysis of flavuxanthin extracted from the Top10/pBAD-crtEbEBI/pJBEI-2999 strain fed with [1–13C]glucose, to establish the labeling pattern of all C5 units in the carotenoid in order to accurately determine if each of the isoprene units was derived from either the MEP or MVA pathways. However, the results were unclear because of an inadequate amount of purified flavuxanthin (data not shown). Finally, we tried to control the metabolic flow through the MEP pathway to see its effect on carotenogenesis. The overexpression of an HMBPP-metabolizing enzyme is considered to reduce the intracellular concentration of HMBPP. E. coli ispH gene encoding HMBPP reductase, which can convert HMBPP into IPP and DMAPP, was additionally inserted into the plasmid for flavuxanthin production. The comparison of carotenoids produced in E. coli cells transformed with the new plasmid pBAD-crtEbEBI-ispH and those with the original plasmid pBAD-crtEbEBI demonstrated that the overexpression of HMBPP reductase caused significant decrease in a flavuxanthin/lycopene ratio (Figure ). This result also supported our hypothesis that C. glutamicum lycopene elongase uses HMBPP as the prenyl donor substrate.
Substrate specificities of (all-E)-prenyl diphosphate synthases
The use of HMBPP as a prenyl donor substrate has been reported with trans-zeatin synthase from Agrobacterium tumefaciens, which catalyzes the transfer of a C5 prenyl group to AMP and prefers HMBPP to DMAPP as the donor substrate for the reaction [Citation24–26]. The utilization of HMBPP by lycopene elongase is, however, still surprising because a few structural studies have proposed an SN1-type carbocation mechanism for UbiA-family prenyltransferases [Citation27,28], in contrast to trans-zeatin synthase that is classified in p-loop containing nucleoside triphosphate hydrolase superfamily and has been suggested to catalyze prenyltransfer reaction with an SN2-type concerted mechanism [Citation25] (Figure ). If we can ignore the difference in the affinity with which a prenyltransferase recognizes a substrate, the preference for HMBPP to DMAPP through the SN1-type reaction seems curious because the existence of an electron-withdrawing 4-hydroxyl group can destabilize the carbocation intermediate. Therefore, we attempted to evaluate the capability of such prenyltransferases to accept HMBPP as the donor substrate instead of DMAPP, their natural substrate. For this purpose, we prepared several short-chain (all-E)-prenyl diphosphate synthases: FPSs from S. cerevisiae [Citation18], L. brevis [Citation17], and G. stearothermophilus [Citation29]; GGPSs from S. acidocaldarius [Citation30] and C. glutamicum [Citation16], GFPS from A. pernix [Citation31]; and, hypothetical GGPS from F. johnsoniae. These synthases accept DMAPP as the primary donor substrate for producing (all-E)-prenyl diphosphates with various carbon chain lengths via consecutive condensation of IPP. Importantly, trans-prenyltransferase-family enzymes including (all-E)-prenyl diphosphate synthases are considered to employ the SN1-type mechanism [Citation32–35]. Among the prepared enzymes, L. brevis FPS, G. stearothermophilus FPS, and C. glutamicum GGPS showed no activity toward HMBPP, but they accepted DMAPP as the substrate (data not shown). Therefore, specific activities of the other enzymes toward 5 μM HMBPP or DMAPP and 5 μM IPP were measured to compare their preferences for the prenyl donor substrates (Table ). The ratios of the specific activities toward HMBPP and DMAPP were relatively high for the archaeal enzymes, at 0.58 for A. pernix GFPS and 0.16 for S. acidocaldarius GGPS, but lower for F. johnsoniae hypothetical GGPS and S. cerevisiae FPS, at 0.018 and 0.011, respectively. Kinetic studies were conducted only with S. acidocaldarius GGPS. As shown in Table , the KM for HMBPP was about threefold larger than that for DMAPP, while the KM for IPP against 25 μM HMBPP was fourfold smaller than that against DMAPP. When HMBPP was used as the substrate, the value for kcat was 6- to 16-fold smaller than that when DMAPP was used. Overall, the 4- to 18-fold lower kcat/KM of the reaction with HMBPP compared with that of the reaction with DMAPP was mainly caused by the difference in kcat, rather than that in KM.
Table 1. Specific activities of (all-E)-prenyl diphosphate synthases.
Table 2. Kinetic parameters of S. acidocaldarius GGPS.
The products of the HMBPP-accepting enzymes were analyzed by radio-TLC after phosphatase treatment. When DMAPP was used as the donor substrate, the chain lengths of the major products from F. johnsoniae hypothetical GGPS, A. pernix GFPS, S. acidocaldarius GGPS, and S. cerevisiae FPS were C30, C25, C20, and C15, respectively (Figure (a)). Contrary to our previous expectation, and also to annotations on public databases such as KEGG, the hypothetical GGPS from F. johnsoniae was shown to be (all-E)-hexaprenyl diphosphate synthase (HexPS), which might be involved in the biosynthesis of menaquinone-6 [Citation36]. When HMBPP was used, the major products of F. johnsoniae HexPS, A. pernix GFPS, and S. acidocaldarius GGPS seemed to get shorter in that order, probably by one isoprene unit (Figure (b)). The radioactive spots from the products of S. cerevisiae FPS from HMBPP were invisible, although a sufficient amount of radioactivity existed before pentane extraction, which was conducted to extract the alcohols obtained from the phosphatase treatment of the products. The disappearance of the spots from the products of S. cerevisiae FPS, and also the fact that spots from the products of S. acidocaldarius GGPS were thinner than that expected from radioactivity existed before pentane extraction, was likely caused by the hydrophilic properties of the alcohols due to the additional hydroxyl groups, which prevented them from being efficiently extracted into the pentane layer. Only the products of S. acidocaldarius GGPS, in diphosphate forms, were analyzed by negative ESI-MS via the direct infusion technique. In the ion spectrum of the sample from the reaction with HMBPP, an ion peak with an m/z of 464.9, which corresponded well with the [M–H]− ion of hydroxylgeranylgeranyl diphosphate, was observed (Figure (c)). Other major ion peaks in the spectrum were also observed in the spectrum of the sample from the reaction with DMAPP, in which we could detect the ion with an m/z of 448.5 derived from geranylgeranyl diphosphate (data not shown). Moreover, the MS/MS analysis of the m/z 464.9 ion gave fragmentation ions with m/z values of 446.9 and 158.6, which corresponded with [M–H2O–H]− and [P2O6H]−, respectively (Figure (d)). These results suggest that the major product of S. acidocaldarius GGPS from HMBPP is hydroxylgeranylgeranyl diphosphate. We also tried to identify the product from HMBPP of A. pernix GFPS by ESI-MS and MS/MS analyses, but could not get reliable data due to unknown contaminants (data not shown).
Discussion
In this study, we tried to prove that HMBPP is the donor substrate of lycopene elongase from C. glutamicum. The results from the two different lines of experiments, i.e. the MEP pathway inhibition experiment and the HMBPP reductase overexpression experiment, support our hypothesis that HMBPP is used for the production of flavuxanthin. Based on these results, we reached the conclusion that the hydroxyl groups of bacterial C50 carotenoids such as flavuxanthin and decaprenoxanthin from C. glutamicum are derived from those of HMBPP. Hydroxylation of carotenoids is usually catalyzed by an hydratase, such as carotenoid 1,2-hydratases CrtC [Citation37–39] and CruF [Citation40,41], or by a monoxygenase, such as cytochrome P450-type [Citation42–44] and non-heme diiron-type carotenoid hydroxylases [Citation45]. Hydratases, however, cannot be employed for hydroxylation at the terminal methyl groups of carotenoids because hydroxylation by the enzymes occurs selectively at the tertiary carbon. Monooxygenases can catalyze the terminal methyl hydroxylation of isoprenoid, as observed with geraniol 8-hydroxylase (CYP76B6) that is involved in iridoid biosynthesis [Citation8,9], or with CYP735A1 and CYP735A2 that are involved in trans-zeatin biosynthesis by plant [Citation46], but the known carotenoid hydroxylases catalyze hydroxylation at the non-methyl positions. The use of HMBPP for the introduction of hydroxyl groups is a limited, but reasonable, path toward the biosynthesis of hydroxylated carotenoids. This approach could be applicable to the bio-production of valuable hydroxylated isoprenoids. For example, the product of S. acidocaldarius GGPS from HMBPP, hydroxygeranylgeranyl diphosphate, has an ω-hydroxyl group probably at position 16 and might be applied as the precursor for the production of important bioactive compounds such as the mating hormones of the plant pathogen Phytophthora [Citation47].
The acceptance of HMBPP by C. glutamicum lycopene elongase and also by some (all-E)-prenyl diphosphate synthases was surprising because these distinct types of prenyltransferases are thought to employ the carbocation-involving SN1-type mechanism. From the results of the kinetic study on S. acidocaldarius GGPS, the low reactivity of the enzyme toward HMBPP compared with that toward DMAPP can be attributed mainly to the difference in kcat, rather than that in KM. This suggests that the difference in the stability of carbocations derived from HMBPP and DMAPP affects the reactivity of the donor substrates, supporting the previously proposed reaction mechanism. Notably, all the (all-E)-prenyl diphosphate synthases that were shown to accept HMBPP in this study are derived from organisms possessing only the MVA pathway. In contrast, the enzymes from organisms possessing the MEP pathway, i.e. C. glutamicum and G. stearothermophilus, showed no activity toward HMBPP, probably because HMBPP is a cellular component that the enzymes would encounter. If the enzymes that naturally utilize DMAPP accept HMBPP as the donor substrate, the resultant unnatural products might disturb downstream isoprenoid biosynthetic pathways or cellular processes involving isoprenoid compounds. L. brevis FPS also do not accept HMBPP even though the organism has only the MVA pathway; however, this fact might be explained by a hypothetical evolutionary scenario where the MVA pathway, which is now known to be minor in domain Bacteria, was acquired by some bacterial lineages via relatively recent horizontal gene transfer events and then replaced the MEP pathway inherited from the common bacterial ancestor. The strict preference toward DMAPP might be an overall trend for bacterial DMAPP-utilizing prenyltransferases because of their or their ancestors’ coexistence with HMBPP. The results from the present study, however, also raise expectations for the existence of undiscovered prenyltransferases that naturally utilize HMBPP as the preferable donor substrate, because the compound is potentially acceptable by a wide variety of prenyltransferases.
Author contributions
H. H. conceived and designed the experiments. Y. H., T. I., Y. T. and H. H. performed the experiments and analyzed the data. Y. H. and H. H. wrote the paper.
Funding
This work was supported by JSPS KAKENHI [grant number 26660060], [grant number 16K14882] and [grant number 17H05437].
Disclosure statement
No potential conflict of interest was reported by the authors.
Acknowledgement
We thank Masaki Honda, Nagoya University, for his assistance in the FAB-MS analysis of flavuxanthin.
References
- Heider SAE, Peters-Wendisch P, Wendisch VF, et al. Metabolic engineering for the microbial production of carotenoids and related products with a focus on the rare C50 carotenoids. Appl Microbiol Biotechnol. 2014;98:4355–4368.10.1007/s00253-014-5693-8
- Dummer AM, Bonsall JC, Cihla JB, et al. Bacterioopsin-mediated regulation of bacterioruberin biosynthesis in Halobacterium salinarum. J Bacteriol. 2011;193:5658–5667.10.1128/JB.05376-11
- Yang Y, Yatsunami R, Ando A, et al. Complete biosynthetic pathway of the C50 carotenoid bacterioruberin from lycopene in the extremely halophilic archaeon Haloarcula japonica. J Bacteriol. 2015;197:1614–1623.10.1128/JB.02523-14
- Krubasik P, Takaichi S, Maoka T, et al. Detailed biosynthetic pathway to decaprenoxanthin diglucoside in Corynebacterium glutamicum and identification of novel intermediates. Arch Microbiol. 2001;176:217–223.10.1007/s002030100315
- Netzer R, Stafsnes MH, Andreassen T, et al. Biosynthetic pathway for γ-cyclic sarcinaxanthin in Micrococcus luteus: heterologous expression and evidence for diverse and multiple catalytic functions of C50 carotenoid cyclases. J Bacteriol. 2010;192:5688–5699.10.1128/JB.00724-10
- Tao L, Yao H, Cheng Q. Genes from a Dietzia sp for synthesis of C40 and C50 β-cyclic carotenoids. Gene. 2007;386:90–97.10.1016/j.gene.2006.08.006
- Krubasik P, Kobayashi M, Sandmann G. Expression and functional analysis of a gene cluster involved in the synthesis of decaprenoxanthin reveals the mechanisms for C50 carotenoid formation. Eur J Biochem. 2001;268:3702–3708.10.1046/j.1432-1327.2001.02275.x
- Collu G, Unver N, Peltenburg-Looman AM, et al. Geraniol 10-hydroxylase, a cytochrome P450 enzyme involved in terpenoid indole alkaloid biosynthesis. FEBS Lett. 2001;508:215–220.10.1016/S0014-5793(01)03045-9
- Höfer R, Dong L, André F, et al. Geraniol hydroxylase and hydroxygeraniol oxidase activities of the CYP76 family of cytochrome P450 enzymes and potential for engineering the early steps of the (seco)iridoid pathway. Metab Eng. 2013;20:221–232.10.1016/j.ymben.2013.08.001
- DeBarber AE, Bleyle LA, Roullet JB, et al. ω-Hydroxylation of farnesol by mammalian cytochromes P450. Biochim Biophys Acta. 2004;1682:18–27.10.1016/j.bbalip.2004.01.003
- Johnston JB, Kells PM, Podust LM, et al. Biochemical and structural characterization of CYP124: a methyl-branched lipid ω-hydroxylase from Mycobacterium tuberculosis. Proc Natl Acad Sci USA. 2009;106:20687–20692.10.1073/pnas.0907398106
- Hayakawa H, Sobue F, Motoyama K, et al. Identification of enzymes involved in the mevalonate pathway of Flavobacterium johnsoniae. Biochem Biophys Res Commun. 2017;487:702–708.10.1016/j.bbrc.2017.04.120
- Ito T, Hemmi H, Kataoka K, et al. A novel zinc-dependent D-serine dehydratase from Saccharomyces cerevisiae. Biochem J. 2008;409:399–406.10.1042/BJ20070642
- Hemmi H, Ohnuma S, Nagaoka K, et al. Identification of genes affecting lycopene formation in Escherichia coli transformed with carotenoid biosynthetic genes: candidates for early genes in isoprenoid biosynthesis. J Biochem (Tokyo). 1998;123:1088–1096.10.1093/oxfordjournals.jbchem.a022047
- Peralta-Yahya PP, Ouellet M, Chan R, et al. Identification and microbial production of a terpene-based advanced biofuel. Nat Commun. 2011;2:483.
- Heider SA, Peters-Wendisch P, Beekwilder J, et al. IdsA is the major geranylgeranyl pyrophosphate synthase involved in carotenogenesis in Corynebacterium glutamicum. FEBS J. 2014;281:4906–4920.10.1111/febs.13033
- Wallrapp FH, Pan JJ, Ramamoorthy G, et al. Prediction of function for the polyprenyl transferase subgroup in the isoprenoid synthase superfamily. Proc Natl Acad Sci USA. 2013;110:E1196–E1202.10.1073/pnas.1300632110
- Blanchard L, Karst F. Characterization of a lysine-to-glutamic acid mutation in a conservative sequence of farnesyl diphosphate synthase from Saccharomyces cerevisiae. Gene. 1993;125:185–189.10.1016/0378-1119(93)90326-X
- Hemmi H, Shibuya K, Takahashi Y, et al. (S)-2,3-Di-O-geranylgeranylglyceryl phosphate synthase from the thermoacidophilic archaeon Sulfolobus solfataricus. Molecular cloning and characterization of a membrane-intrinsic prenyltransferase involved in the biosynthesis of archaeal ether-linked membrane lipids. J Biol Chem. 2004;279:50197–50203.
- Ogawa T, Yoshimura T, Hemmi H. Geranylfarnesyl diphosphate synthase from Methanosarcina mazei: different role, different evolution. Biochem Biophys Res Commun. 2010;393:16–20.10.1016/j.bbrc.2010.01.063
- S-I Ohnuma, Nakazawa T, Hemmi H, et al. Conversion from farnesyl diphosphate synthase to geranylgeranyl diphosphate synthase by random chemical mutagenesis. J Biol Chem. 1996;271:10087–10095.
- Fujii H, Koyama T, Ogura K. Efficient enzymatic hydrolysis of polyprenyl pyrophosphates. Biochim Biophys Acta. 1982;712:716–718.
- Jomaa H, Wiesner J, Sanderbrand S, et al. Inhibitors of the nonmevalonate pathway of isoprenoid biosynthesis as antimalarial drugs. Science. 1999;285:1573–1576.10.1126/science.285.5433.1573
- Sakakibara H, Kasahara H, Ueda N, et al. Agrobacterium tumefaciens increases cytokinin production in plastids by modifying the biosynthetic pathway in the host plant. Proc Natl Acad Sci USA. 2005;102:9972–9977.10.1073/pnas.0500793102
- Sugawara H, Ueda N, Kojima M, et al. Structural insight into the reaction mechanism and evolution of cytokinin biosynthesis. Proc Natl Acad Sci USA. 2008;105:2734–2739.10.1073/pnas.0707374105
- Ueda N, Kojima M, Suzuki K, et al. Agrobacterium tumefaciens tumor morphology root plastid localization and preferential usage of hydroxylated prenyl donor is important for efficient gall formation. Plant Physiol. 2012;159:1064–1072.10.1104/pp.112.198572
- Cheng W, Li W. Structural insights into ubiquinone biosynthesis in membranes. Science. 2014;343:878–881.10.1126/science.1246774
- Huang H, Levin EJ, Liu S, et al. Structure of a membrane-embedded prenyltransferase homologous to UBIAD1. PLoS Biol. 2014;12:e1001911.10.1371/journal.pbio.1001911
- Koyama T, Obata S, Osabe M, et al. Thermostable farnesyl diphosphate synthase of Bacillus stearothermophilus: molecular cloning, sequence determination, overproduction, and purification. J Biochem. 1993;113:355–263.10.1093/oxfordjournals.jbchem.a124051
- S-I Ohnuma, Suzuki M, Nishino T. Archaebacterial ether-linked lipid biosynthetic gene. Expression cloning, sequencing, and characterization of geranylgeranyl-diphosphate synthase. J Biol Chem. 1994;269:14792–14797.
- Tachibana A, Yano Y, Otani S, et al. Novel prenyltransferase gene encoding farnesylgeranyl diphosphate synthase from a hyperthermophilic archaeon, Aeropyrum pernix. Molecularevolution with alteration in product specificity. Eur J Biochem. 2000;267:321–328.
- Lu YP, Liu HG, Liang PH. Different reaction mechanisms for cis- and trans-prenyltransferases. Biochem Biophys Res Commun. 2009;379:351–355.10.1016/j.bbrc.2008.12.061
- Poulter CD, Argyle JC, Mash EA. Letter: Prenyltransferase. New evidence for an ionization-condensation-elimination mechanism with 2-fluorogeranyl pyrophosphate. J Am Chem Soc. 1977;99:957–959.10.1021/ja00445a056
- Sanchez VM, Crespo A, Gutkind JS, et al. Investigation of the catalytic mechanism of farnesyl pyrophosphate synthase by computer simulation. J Phys Chem B. 2006;110:18052–18057.10.1021/jp063099q
- Thulasiram HV, Erickson HK, Poulter CD. A common mechanism for branching, cyclopropanation, and cyclobutanation reactions in the isoprenoid biosynthetic pathway. J Am Chem Soc. 2008;130:1966–1971.10.1021/ja0771282
- Bernardet JF, Segers P, Vancanneyt M, et al. Cutting a gordian knot: emended classification and description of the genus Flavobacterium, emended description of the family Flavobacteriaceae, and proposal of Flavobacterium hydatis nom. nov. (basonym, Cytophaga aquatilis Strohl and Tait 1978). Int J Sys Bacteriol. 1996;46:128–148.
- Hiseni A, Arends IW, Otten LG. Biochemical characterization of the carotenoid 1,2-hydratases (CrtC) from Rubrivivax gelatinosus and Thiocapsa roseopersicina. Appl Microbiol Biotechnol. 2011;91:1029–1036.10.1007/s00253-011-3324-1
- Lang HP, Cogdell RJ, Takaichi S, et al. Complete DNA sequence, specific Tn5 insertion map, and gene assignment of the carotenoid biosynthesis pathway of Rhodobacter sphaeroides. J Bacteriol. 1995;177:2064–2073.10.1128/jb.177.8.2064-2073.1995
- Steiger S, Mazet A, Sandmann G. Heterologous expression, purification, and enzymatic characterization of the acyclic carotenoid 1,2-hydratase from Rubrivivax gelatinosus. Arch Biochem Biophys. 2003;414:51–58.10.1016/S0003-9861(03)00099-7
- Graham JE, Bryant DA. The biosynthetic pathway for myxol-2′ fucoside (myxoxanthophyll) in the cyanobacterium Synechococcus sp. Strain PCC 7002. J Bacteriol. 2009;191:3292–3300.10.1128/JB.00050-09
- Sun Z, Shen S, Wang C, et al. A novel carotenoid 1,2-hydratase (CruF) from two species of the non-photosynthetic bacterium Deinococcus. Microbiology. 2009;155:2775–2783.10.1099/mic.0.027623-0
- Blasco F, Kauffmann I, Schmid RD. CYP175A1 from Thermus thermophilus HB27, the first β-carotene hydroxylase of the P450 superfamily. Appl Microbiol Biotechnol. 2004;64:671–674.10.1007/s00253-003-1529-7
- Kim J, DellaPenna D. Defining the primary route for lutein synthesis in plants: the role of Arabidopsis carotenoid β-ring hydroxylase CYP97A3. Proc Natl Acad Sci USA. 2006;103:3474–3479.10.1073/pnas.0511207103
- Tian L, Musetti V, Kim J, et al. The Arabidopsis LUT1 locus encodes a member of the cytochrome P450 family that is required for carotenoid ε-ring hydroxylation activity. Proc Natl Acad Sci USA. 2004;101:402–407.10.1073/pnas.2237237100
- Tian L, DellaPenna D. Progress in understanding the origin and functions of carotenoid hydroxylases in plants. Arch Biochem Biophys. 2004;430:22–29.10.1016/j.abb.2004.02.003
- Takei K, Yamaya T, Sakakibara H. Arabidopsis CYP735A1 and CYP735A2 encode cytokinin hydroxylases that catalyze the biosynthesis of trans-zeatin. J Biol Chem. 2004;279:41866–41872.10.1074/jbc.M406337200
- Qi J, Asano T, Jinno M, et al. Characterization of a phytophthora mating hormone. Science. 2005;309:1828.10.1126/science.1114756