Abstract
The transient receptor potential (TRP) V1 is a cation channel belonging to the TRP channel family and it has been reported to be involved in energy metabolism, especially glucose metabolism. While, we have previously shown that intragastric administration of allyl isothiocyanate (AITC) enhanced glucose metabolism via TRPV1, the underlying mechanism has not been elucidated. In this study, we examined the relationship between insulin secretion and the increase in carbohydrate oxidation due to AITC. Intragastric administration of AITC elevated blood insulin levels in mice and AITC directly enhanced insulin secretion from isolated islets. These observations were not reproduced in TRPV1 knockout mice. Furthermore, AITC did not increase carbohydrate oxidation in streptozotocin-treated mice. These results suggest that intragastric administration of AITC could induce insulin secretion from islets via TRPV1 and that enhancement of insulin secretion was related to the increased carbohydrate oxidation due to AITC.
Intragastric administration of AITC could induce insulin secretion via TRPV1 and that insulin secretion was related to the increased carbohydrate oxidation due to AITC.
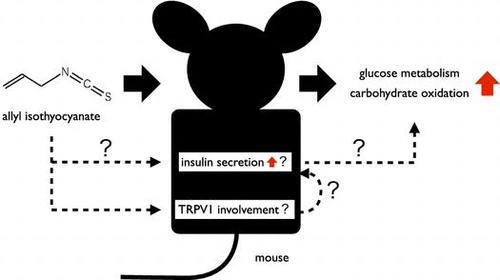
The transient receptor potential (TRP) channel family comprises a wide variety of cation-permeable channels, which have diverse mechanisms of activation. TRPV1 and TRPA1 are cation channels belonging to the TRP channel family, and are activated by high [Citation1,2] and low [Citation3] nociceptive temperatures, respectively. They are also activated by spicy or pungent compounds in foods [Citation1,4,5]. Recent studies have suggested that TRPV1 and TRPA1 are expressed in both sensory tissues and non-sensory tissues, and that their activation is involved not only in nociception and thermosensation but also in thermoregulation and energy metabolism [Citation6–10]. Therefore, detailed elucidation of the physiological relationship between these channels and energy metabolism would be useful, allowing control of metabolic disorders via intake of foods that are TRP channel agonists.
Allyl isothiocyanate (AITC) is a natural compound found in plants belonging to the family Cruciferae, and is the pungent ingredient in mustard, horseradish, and wasabi. AITC is a TRPA1 agonist [Citation11], and recent studies have suggested that AITC activates not only TRPA1 but also TRPV1 [Citation7,12]. We previously reported that intragastric administration of AITC increased carbohydrate oxidation via TRPV1 in mice [Citation7] and that it reduced elevated blood glucose levels by increasing the consumption of extra blood glucose in intraperitoneal glucose tolerance test [Citation13]. Thus, we have shown that the intragastric administration of AITC enhanced glucose metabolism via TRPV1; however, the detailed mechanism remains unclear.
Recent studies have suggested that TRPV1 is involved in the emergence of diabetes and obesity [Citation10]. Long-term treatment of ob/ob mice with TRPV1 antagonists led to a decrease in levels of fasting glucose, triglycerides, and insulin [Citation9]. Moreover, dietary capsaicin, which is a TRPV1 agonist, is expected to ameliorate not only obesity-induced inflammation but also obesity-related metabolic disorders such as insulin resistance [Citation8]. It has also been reported that TRPV1 modulated insulin secretion in rat pancreatic β-cells [Citation14]. Therefore, TRPV1 is believed to play an important role in glucose metabolism, especially in the regulation of insulin secretion or insulin sensitivity.
For these reasons, we hypothesized that insulin secretion via TRPV1 was involved in the changes observed in glucose metabolism following AITC administration. Our previous study also indicated that vagus nerves were not involved in the changes in glucose metabolism due to AITC and that AITC might act directly on the pancreas after absorption in the gastrointestinal tract [Citation13]. Therefore, we aimed to examine whether AITC could alter blood insulin levels and directly induce insulin secretion from pancreatic islets via TRPV1 and also whether the insulin secretion was involved in the increase in carbohydrate oxidation due to AITC. These experiments aimed to reveal the detailed mechanism responsible for the increase in carbohydrate oxidation via TRPV1 and to demonstrate the importance of TRPV1 in glucose metabolism.
In this study, the effects of intragastric administration of AITC on blood insulin levels in mice were examined. To elucidate the effects of AITC on pancreatic islets, insulin secretion from isolated pancreatic islets following AITC administration was measured. We also investigated the relationship between TRP channels and these effects using TRPA1 knockout (KO) mice and TRPV1 KO mice. Furthermore, to elucidate the relationship between insulin secretion and the increase in carbohydrate oxidation due to AITC, the effects of insulin administration on energy metabolism were investigated by respiratory gas analysis.
Materials and methods
Animals
Male C57BL/6 mice (SLC, Hamamatsu, Japan) were used. Mutant TRPV1-null mice and TRPA1-null mice were generously provided by Dr D. Julius (University of California, San Francisco); their production has been previously reported [Citation15,16]. Mutant mice were back-crossed into the C57BL/6 genetic background. The mice were housed in a vivarium maintained at 23 ± 2 °C under a 12:12 h light:dark cycle (lights on: 0600–1800 h) with free access to a commercial standard laboratory chow (MF; Oriental Yeast, Tokyo, Japan) and drinking water. All experimental protocols were approved by the Institutional Animal Care and Use Committee of Kyoto University and were in compliance with the National Institutes of Health Guide for the Care and Use of Laboratory Animals.
Measurements of blood insulin levels
The mice (7–9 weeks old) were deprived of food for 3 h (0900–1200 h) but had free access to water. AITC (25 mg/kg body mass) or vehicle was administered intragastrically and blood insulin levels were measured before and after administration. Blood samples were obtained by decapitation and serum insulin levels were measured with an insulin immunoassay kit (Shibayagi, Gunma, Japan).
Measurement of insulin secretion and residual insulin content of isolated islets
Detailed methodologies to assess insulin output from collagenase-isolated islets have been previously described [Citation17]. Male mice (weight: 25–30 g) were anesthetized using pentobarbital sodium (50 mg/kg body mass). Islets were isolated by collagenase digestion, handpicked under a stereomicroscope, and placed into Krebs-Ringer bicarbonate (KRB) buffer supplemented with 8 mM glucose. The islets were free of exocrine contamination.
After isolation, islets were loaded onto nylon filters (Sefar America, Kansas City, MO) and preincubated for 30 min with KRB buffer containing 3 mM glucose. Groups of five islets were transferred into each glass vial and incubated with 500 μL of KRB buffer containing 8 mM glucose or KRB buffer containing 8 mM glucose supplemented with AITC. The vial was capped with a rubber stopper and gassed for 10 s with 95% O2:5% CO2 and islets were then incubated for 2 h at 37 °C. After incubation, the supernatant of the solutions was collected and the insulin released into the solution was measured by radioimmunoassay (RIA) [Citation18] and determined to be the pancreatic insulin secretion. Hanks’ solution (500 μL) was added to the pellets (islets) in the glass vial and sonicated. The supernatant of the sonicated solutions was collected and insulin was measured by RIA and determined to be the residual insulin content in islets. Solutions were stored at −30 °C until analysis.
Synthesis of NAC-AITC
NAC-AITC was synthesized as described previously with a slight modification [Citation19]. Briefly, N-acetyl-L-cysteine (200 mg, 1.2 mmol) and AITC (100 mg, 1.0 mmol) were dissolved in methanol (10 mL). Then, potassium carbonate (200 mg) was added and the solution was stirred for 1 h under an N2 atmosphere. Acetic acid (1 mL) was added, the solution was filtered through a 0.2-μm membrane filter, and then purified by preparative HPLC performed using a Hitachi system (Hitachi, Tokyo, Japan) consisting of an L-7420 UV detector equipped with a chromatography data station software. The column was a 5-μm Capcell Pak C18 10 × 250 mm UG80 (Shiseido, Tokyo, Japan). The mobile phase was a mixture of 1:9 acetonitrile:water containing 0.2% acetic acid, delivered at a flow rate of 2 mL/min, with peak detection at a wavelength of 254 nm. Finally, NAC-AITC was purified from the collected solution by evaporation and freeze-drying, and stored at 4 °C under an N2 atmosphere.
Reagents
Allyl isothiocyanate was purchased from Nacalai Tesque (Kyoto, Japan). For intragastric administration, AITC was diluted in a saline solution containing 3% ethanol and 10% Tween 80. In vitro experiments, AITC and NAC-AITC were diluted in the incubation solution. The salt solution for incubation (KRB buffer) consisted of 115 mM NaCl, 5 mM KCl, 1 mM MgCl2, 2.2 mM CaCl2, 7 mM NaHCO3, and 0.17% bovine serum albumin. Hanks’ solution consisted of 138 mM NaCl, 5.3 mM KCl, 1.2 mM CaCl2, 0.41 mM MgSO4, 0.34 mM NaHPO4, 0.44 mM KH2PO4, and adjusted to pH 7.4 with NaHCO3. The 125I-labeled insulin for the insulin assay was purchased from PerkinElmer Life Sciences (Tokyo, Japan). Bovine serum albumin (RIA grade) was obtained from Sigma (St. Louis, MO). Glucose and other salts used in buffer preparation were purchased from Wako Chemicals (Tokyo, Japan). Collagenase (type P) was obtained from Roche (Indianapolis, IN).
Respiratory gas analysis
Prior to analysis, the mice were kept individually in chambers for a period of 3 h in order to attain a constant respiratory exchange ratio (RER). Insulin (Humulin R; Eli Lilly and Co.) or glibenclamide (Sigma, St. Louis, MO) was administered intraperitoneally or intragastrically, respectively, and the expired air was analyzed. The oxidation of total fatty acids and carbohydrates was computed using the rates of oxygen consumption () and carbon dioxide production (
). Gas analysis was performed using an open-circuit metabolic gas analysis system connected directly to a mass spectrometer (Arco2000; ArcoSystem, Chiba, Japan). The gas analysis system has been described in detail elsewhere [Citation7,13]. Briefly, each metabolic chamber had a 72-cm2 floor and was 6 cm in height. Room air was pumped through the chambers at a rate of 0.5 L/min. Expired air was dried in a cotton thin column and then directed to an O2/CO2 analyzer for mass spectrometry.
On the basis of the volume of gas production per unit of time (L/min; and
), the total glucose and lipid oxidation were calculated using the stoichiometric equations of Frayn [Citation20] as follows: total fatty acid oxidation = 1.67
−1.67
and carbohydrate oxidation = 4.55
−3.21
.
Respiratory gas analysis of streptozotocin-treated mice
To attenuate insulin secretion, mice were intraperitoneally administered with streptozotocin (STZ) (100 mg/kg body mass). Experiments were performed after a recovery period of 7 days. To confirm the effectiveness of STZ-treatment, glucose levels were measured immediately prior to respiratory gas analysis by Glucocard Diameter (Arkray, Kyoto, Japan). In STZ-treated mice, the average blood glucose level was 395 ± 42; the average for control-treated mice was 155 ± 8. In the respiratory analysis experiments, the mice were fasted for 3 h before AITC was intragastrically administered.
Data analysis
All values are presented as mean ± SEM. The effect of intragastric administration of AITC on blood insulin levels was examined by two-way repeated-measures ANOVA with Bonferroni post hoc test (Prism 5.0; GraphPad Software, San Diego, CA) (Figure (a)). In TRPA1 or TRPV1 KO mice, the effects of intragastric administration of AITC on blood insulin levels were examined by two-way repeated-measures ANOVA with Bonferroni post hoc test (Figure ). The effects of AITC or NAC-AITC treatment on insulin secretion and residual insulin content were analyzed by unpaired t test (Figures and ). The effect of administration of insulin or glibenclamide on RER, oxygen consumption, carbohydrate oxidation, and fat oxidation was examined by two-way repeated-measures ANOVA with Bonferroni post hoc test (Figures and ). The effects of intraperitoneal administration of insulin on average RER and cumulative oxygen consumption, carbohydrate oxidation, and fat oxidation for 2 h were examined by one-way ANOVA (Figure ). Tukey’s test was used as a post hoc test. The effect of intragastric administration of AITC to STZ-treated mice on RER and oxygen consumption was examined by two-way repeated-measures ANOVA with Bonferroni post hoc test (Figure ).
Figure 1. Changes in blood insulin levels of wild type mice. (a) Changes in blood insulin levels of mice administered with AITC or vehicle (control). Values are expressed as means ± SE. n = 4; *p < 0.05 (two-way repeated-measures ANOVA, followed by Bonferroni’s post hoc test). (b) Area under the curve of blood insulin levels of mice administered with AITC or vehicle. Values are expressed as means ± SE. n = 4; *p < 0.05 (unpaired t test).
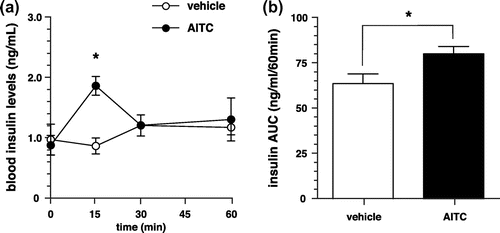
Figure 2. Changes in blood insulin levels of TRPA1 KO or TRPV1 KO mice. (a) Changes in blood insulin levels of TRPA1 KO mice administered with AITC or vehicle (control). Values are expressed as means ± SE. n = 5; *p < 0.05 (two-way repeated-measures ANOVA, followed by Bonferroni’s post hoc test). (b) Changes in blood insulin levels of TRPV1 KO mice administered with AITC or vehicle (control). Values are expressed as means ± SE. n = 7. There is no significant difference between the groups.
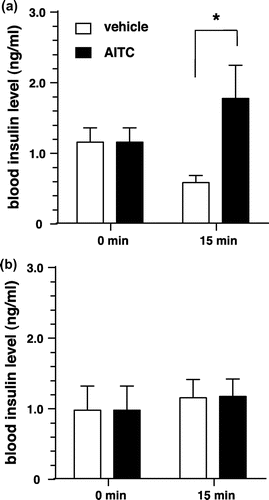
Figure 3. Insulin secretion and residual insulin content of isolated islets by AITC. (a) and (b) Effects of AITC on insulin secretion and residual insulin content of isolated islets from WT mice. Groups of islets were stimulated with 8.0 mM glucose buffer including AITC (0 or 20 nM) for 2 h. Released insulin into the buffer (a) and remained insulin in the islets (b) were measured. Values are expressed as means ± SE. n = 7–12. *p < 0.05 (unpaired t test). (c) and (d) Effects of AITC on insulin secretion and residual insulin content of isolated islets from TRPV1 KO mice. Values are expressed as means ± SE. n = 10–12. There is no significant difference between the groups.
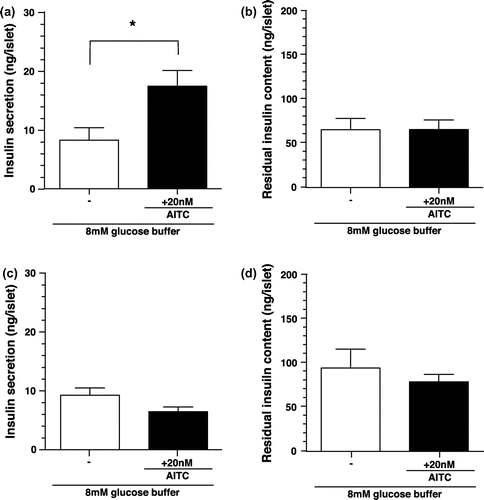
Figure 4. Effects of NAC-AITC for isolated islets on insulin secretion and residual insulin content in islets. (a) and (b) Effects of NAC-AITC on insulin secretion and residual insulin content of isolated islets from WT mice. Groups of islets were stimulated with 8.0 mM glucose buffer including NAC-AITC (0, 2 or 20 nM) for 2 h. Released insulin into the buffer (a) and remained insulin in the islets (b) were measured. Values are expressed as means ± SE. n = 18–19. *p < 0.05 (unpaired t test). (c) and (d) Effects of NAC-AITC on insulin secretion and residual insulin content of isolated islets from TRPV1 KO mice. Values are expressed as means ± SE. n = 10–11. *p < 0.05 (unpaired t test).
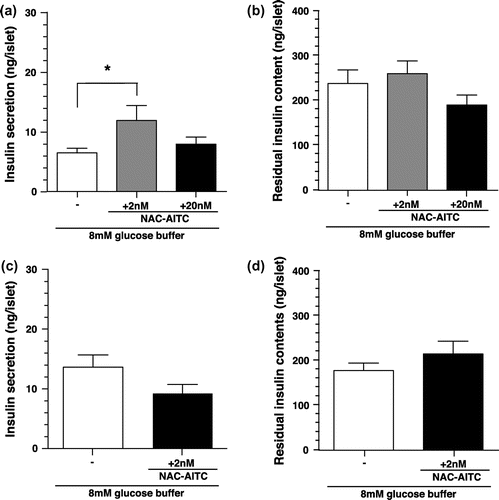
Figure 5. Respiratory gas analysis with insulin administered mice. (a) Changes in the respiratory exchange ratio of mice administered with insulin or saline (control). Values are expressed as means ± SEM. n = 12 (saline vs. insulin 0.5 IU/kg: p < 0.05 at 20–30 min; saline vs. insulin 1.0 IU/kg: p < 0.05 at 20–100 min; two-way repeated-measures ANOVA, followed by Bonferroni’s post hoc test). (b) Changes in oxygen consumption of mice administered with insulin or saline (control). Values are expressed as means ± SEM (n = 12). (c) Changes in carbohydrate oxidation of mice administered with insulin or saline (control). Values are expressed as means ± SEM. n = 12 (saline vs. insulin 0.5 IU/kg: p < 0.05 at 20–30 min; saline vs. insulin 1.0 IU/kg: p < 0.05 at 20–80 min; two-way repeated-measures ANOVA, followed by Bonferroni’s post hoc test). (d) Changes in fat oxidation of mice administered with insulin or saline (control). Values are expressed as means ± SEM. n = 12 (saline vs. insulin 0.5 IU/kg: p < 0.05 at 20–30 min; saline vs. insulin 1.0 IU/kg: p < 0.05 at 20–110 min; two-way repeated-measures ANOVA, followed by Bonferroni’s post hoc test).
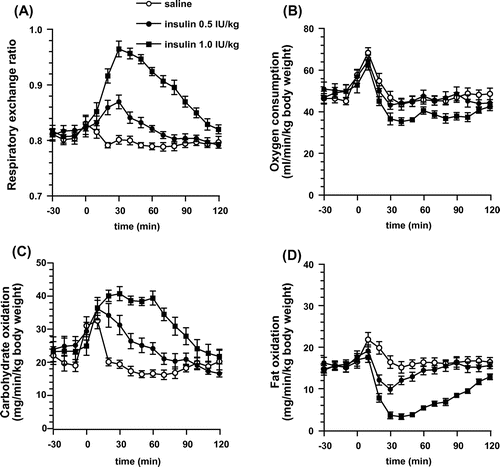
Figure 6. Changes in respiratory gas with insulin administered mice. (a) Average the respiratory exchange ratio of mice for 2 h after intraperitoneal administration of insulin or saline (control). (b)–(d) Cumulative oxygen consumption, carbohydrate oxidation, and fat oxidation of mice for 2 h after intraperitoneal administration of insulin or saline (control). Values are expressed as means ± SEM. n = 12; *p < 0.05 (Tukey’s test).
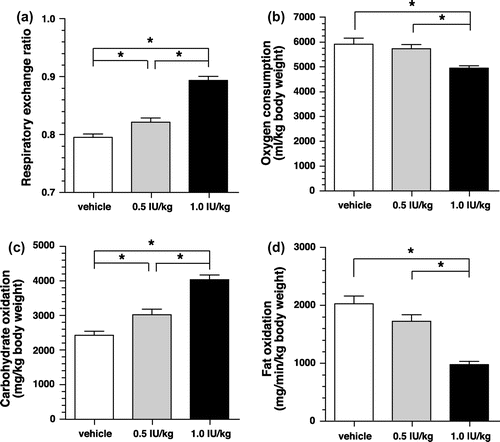
Results
Effects of intragastric administration of AITC on blood insulin levels
We measured blood insulin levels after intragastric administration of AITC. The AITC dosage was established from our previous study wherein it we determined a sufficient dose to affect energy metabolism [Citation7]. Mice were fasted for 3 h before the experiment to eliminate potential interference from dietary components on their metabolism. Intragastric administration of AITC elevated blood insulin levels for 15 min after administration to nearly twice as high as those of the control group (Figure (a)). The area under the curve (AUC) of the blood insulin levels for 60 min after administration was higher in the AITC-treated group than in the vehicle-treated group (Figure (b)).
In TRPA1 KO mice, intragastric administration of AITC elevated the blood insulin levels for 15 min after administration compared with the values reported for the control group (Figure (a)). This finding was similar to that observed in wild type (WT) mice. Conversely, in TRPV1 KO mice, intragastric administration of AITC did not elevate the blood insulin levels (Figure (b)).
AITC induces insulin secretion from isolated islets
We examined the effects of AITC on insulin secretion from isolated islets under physiological glucose conditions (8 mM; 144 mg/dL). Islets from mice were preincubated with 3 mM glucose to establish basal stable insulin secretory rates. After preincubation, islets were stimulated by 8 mM glucose, with or without AITC, for 2 h. A 20 nM dose of AITC increased the insulin secretion from isolated islets to nearly twice as high as that of the control (Figure (a)). With regard to the residual insulin content in islets over 2 h, there was no significant difference between the AITC-treated group and the vehicle-treated group (Figure (b)). At a dose of 200 nM, no increase in insulin secretion was observed and the residual insulin content in islets was lower in the AITC-treated group than in the vehicle-treated group (data not shown).
In TRPV1 KO mice, the insulin secretion from the isolated islets tended to be lower in the AITC-treated group, than in the vehicle-treated group; however, there was no significant difference between the two groups (Figure (c)). There was no significant difference in residual insulin content in islets between the AITC-treated group and the vehicle-treated group (Figure (d)). These findings differed from those reported for WT mice.
NAC-AITC induces insulin secretion from isolated islets
Previous studies reported that AITC was primarily metabolized in vivo through the mercapturic acid pathway to an N-acetylcysteine (NAC) conjugate (NAC-AITC) [Citation21–23]. Therefore, we examined whether NAC-AITC increased the insulin secretion from isolated islets, and a dose of 2 nM was sufficient to produce an increase (Figure (a)). The residual insulin content in islets over a period of 2 h showed no significant difference between the NAC-AITC-treated group and the vehicle-treated group (Figure (b)). At a dose of 20 nM, the increase in insulin secretion was not observed and the residual insulin content in islets was tended to be lower in the NAC-AITC-treated group than in the vehicle-treated group.
In TRPV1 KO mice, the insulin secretion from isolated islets tended to be lower in the NAC-AITC-treated group, than in the vehicle-treated group; however, there was no significant difference between the two groups (Figure (c)). The residual insulin content in islets showed no significant difference between the NAC-AITC-treated group and the vehicle-treated group (Figure (d)), results different from those reported for WT mice.
Relationship between insulin secretion and the increase in carbohydrate oxidation due to AITC
In previous studies, we demonstrated that intragastric administration of AITC increased carbohydrate oxidation [Citation7]. To elucidate the relationship between the insulin secretion and the increase in carbohydrate oxidation, we analyzed respiratory gases after insulin administration. Intraperitoneal administration of insulin dose-dependently elevated RER for 2 h after administration in comparison to the administration of a control saline solution (Figure ). For both dose rates tested, peak RER elevation was observed at 30 min after administration. Insulin administration dose-dependently increased carbohydrate oxidation for 2 h after administration in comparison to the control. In contrast, insulin administration dose-dependently decreased fat oxidation for 2 h after administration. Oxygen consumption decreased 30–100 min after administration in the 1.0 IU/kg insulin-treated group, but not in the other groups (Figure ).
We also used glibenclamide, a sulfonylurea, to elucidate the effects of insulin secretion on carbohydrate oxidation under physiological conditions. Intragastric administration of glibenclamide dose-dependently elevated RER for 2 h after administration compared with the administration of a control solution of DMSO (Figure ). For both dose rates tested, peak RER elevation was observed at 60 min after administration. Glibenclamide dose-dependently increased carbohydrate oxidation and decreased fat oxidation for 2 h after administration in comparison to the control. These findings were similar to those observed in insulin-treated mice. Oxygen consumption was unaffected for at least 2 h following administration.
Figure 7. Respiratory gas analysis with glibenclamide administered mice. (a) Changes in the respiratory exchange ratio of mice administered with glibenclamide or DMSO (control). Values are expressed as means ± SEM. n = 9–10 (DMSO vs. glibenclamide 10 mg/kg: p < 0.05 at 40–70 min; DMSO vs. glibenclamide 30 mg/kg: p < 0.05 at 30–80 min; two-way repeated-measures ANOVA, followed by Bonferroni’s post hoc test). (b) Changes in oxygen consumption of mice administered with glibenclamide or DMSO (control). Values are expressed as means ± SEM (n = 9–10). (c) Changes in carbohydrate oxidation of mice administered with glibenclamide or DMSO (control). Values are expressed as means ± SEM. n = 9–10 (DMSO vs. glibenclamide 10 mg/kg: p < 0.05 at 30–40, 60 min; DMSO vs. glibenclamide 30 mg/kg: p < 0.05 at 40–60 min; two-way repeated-measures ANOVA, followed by Bonferroni’s post hoc test). (d) Changes in fat oxidation of mice administered with glibenclamide or DMSO (control). Values are expressed as means ± SEM. n = 9–10 (DMSO vs. glibenclamide 10 mg/kg: p < 0.05 at 50–60 min; DMSO vs. glibenclamide 30 mg/kg: p < 0.05 at 40–80 min; two-way repeated-measures ANOVA, followed by Bonferroni’s post hoc test).
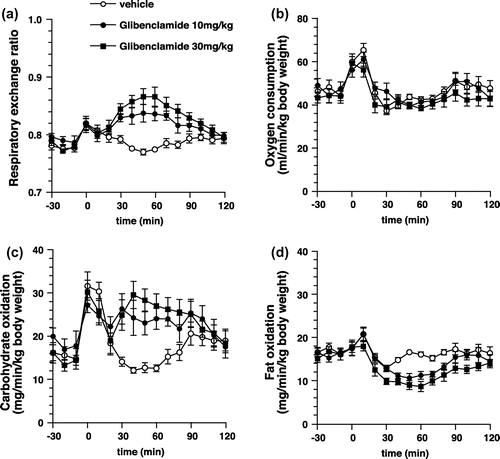
Effects of STZ-treatment on the changes in energy metabolism due to AITC
To elucidate the relationship between the insulin secretion and the increase in carbohydrate oxidation due to AITC, we used STZ-treated mice. Intragastric administration of AITC elevated RER in the vehicle-treated mice, but not in the STZ-treated mice (Figure ). Oxygen consumption was not unaffected for at least 2 h following administration in both groups.
Figure 8. Respiratory gas analysis with STZ-treated mice. (a) and (b) Changes in the respiratory exchange ratio and oxygen consumption of STZ-treated mice administered with AITC (25 mg/kg) or vehicle (control). Values are expressed as means ± SEM (n = 9–10). (c) and (d) Changes in the respiratory exchange ratio and oxygen consumption of sham-treated mice administered with AITC (25 mg/kg) or vehicle (control). Values are expressed as means ± SEM (n = 7–10). *p < 0.05 (two-way repeated-measures ANOVA, followed by Bonferroni’s post hoc test).
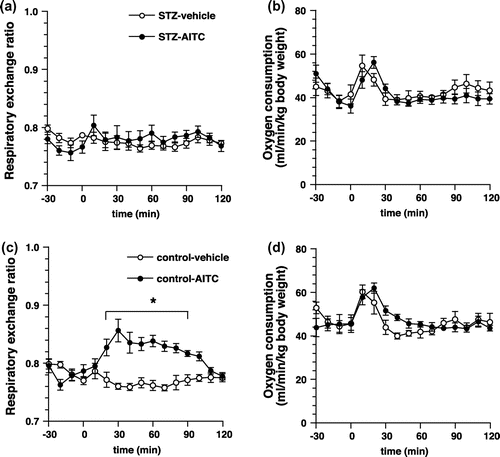
Discussion
In the present study, we observed that intragastric administration of AITC increased blood insulin levels at 15 min after administration (Figure ). AITC also increased blood insulin levels at 15 min after administration in TRPA1 KO mice, but not in TRPV1 KO mice (Figure ). These results suggest that TRPV1, but not TRPA1, is involved in the increase in blood insulin level due to AITC.
AITC increased insulin secretion, but did not affect residual insulin content in islets, in isolated islets from WT mice (Figure (a) and (b)). In islets from TRPV1 KO mice, AITC did not increase insulin secretion (Figure (c) and (d)). These results suggest that TRPV1 is involved in the AITC-induced enhancement of insulin secretion from islets. However, the existence and function of TRPV1 in pancreatic β-cells remain controversial. TRPV1 immunoreactivity has been shown in primary β-cells of Sprague-Dawley rats by Akiba et al. [Citation14], but not in those of Zucker diabetic rats [Citation24] or NOD mice [Citation25]. TRPV1 has been shown to exist in nerve fibers innervating pancreatic islets in mice [Citation25]. It has been also reported that TRPV1 is expressed in a rat pancreatic β-cell line (RIN-5F) and that capsaicin could induce insulin secretion from rat pancreatic β-cells via TRPV1 [Citation14]. However, it has been suggested that TRPV1 did not contribute to glucose-induced insulin secretion in β-cells [Citation26]. Considering this body of information, AITC may enhance insulin secretion by effects other than those on β-cells, such as paracrine action on the neighboring α-cells releasing glucagon [Citation27,28]. In addition, recent study has identified TRPV1 signaling converging onto secretagogin expression as an important regulatory axis for both β-cell secretory function and survival [Citation29]. In TRPV1 KO mice, the down regulation of secretagogin expression might affect insulin secretion from islets. Although the relationship between insulin secretion and TRPV1 activation in β-cells remains controversial, our results suggest that TRPV1 is at least involved in insulin secretion from islets.
Previous studies reported that, AITC was primarily metabolized to NAC-AITC in rats and approximately 80% of the administered AITC in the urine existed as the thiocyanate ion and 10–20% of that did as NAC-conjugate in mice [Citation22]. Therefore, it is assumed that both AITC and its metabolite, NAC-AITC, could act on pancreatic islets after absorption in the gastrointestinal tract. Indeed, our previous study showed that intraperitoneal administration of AITC could elevate RER [Citation7]. In this study, both AITC and NAC-AITC were able to induce insulin secretion from isolated islets, indicating that both could directly induce insulin secretion from the pancreas.
NAC-AITC enhanced insulin secretion from isolated islets; however, the efficient dose of NAC-AITC (2 nM) was different from that of AITC (20 nM) (Figures and ). The residual insulin content in islets tended to be lower in islets treated with 20 nM NAC-AITC than those treated with control solution in WT mice. Decreased residual insulin content in islets may represent a decrease in insulin synthesis leading to a suppression of the amount of increase in the insulin secretion from the islets. A high dose of AITC decreased the residual insulin content in islets and did not increase the insulin secretion from islets (data not shown). The conjugation with NAC may enhance the suppressive effect of AITC on insulin synthesis, and the higher dose of NAC-AITC could suppress insulin synthesis, followed by inhibition of the enhancement of insulin secretion. Furthermore, we could not examine the TRPV1 activation potential of NAC-AITC in this study. Together with these facts, there is a possibility of alternative mechanisms to enhance insulin by NAC-AITC and more detailed studies are needed to confirm these points in future.
In this study, we showed that AITC directly enhanced insulin secretion from isolated mice pancreatic islets, however, but the involvement of the AITC-induced insulin secretion and TRPV1 activation by AITC is not confirmed precisely. In addition, the effective concentrations of AITC for insulin secretion from islets were lower than those for TRPV1 activation in the pervious in vitro experiments [Citation7,12]. In our previous study, 20 μM of AITC caused a significant increase of [Ca2+]i in HEK293 cells expressing TRPV1 [Citation7]. Taken together with the concentration of capsaicin required for TRPV1 activation (μM order) [Citation1], AITC could activate TRPV1 with about 10–100 fold higher than that of capsaicin. Since the effective concentration of capsaicin for enhancement of insulin secretion in RIN-5F is 0.1–10 nM [Citation14], the effective concentration of AITC could be 1–1000 nM. Furthermore, AITC gradually increases [Ca2+]i in those cells via TRPV1 [Citation7,12], therefore, the reasonably long incubation time (2 h) might allow to activate TRPV1 by AITC at lower concentrations (2–20 nM) than those in the pervious in vitro experiments. Although we believe that AITC could activate TRPV1 at these concentrations in islets, further studies are needed to elucidate these points.
Insulin and glibenclamide administration increased carbohydrate oxidation in mice (Figures (c) and (c)). At a dose of 1.0 IU/kg, insulin decreased for 2 h after administration (Figure (b)). This decrease in
was assumed to result from the suppression of spontaneous behavior due to hypoglycemia. Glibenclamide is a sulfonylurea and it enhances insulin secretion from pancreatic β-cells, resulting in an increase in blood insulin levels under physiological conditions. Therefore, the increase in blood insulin level could increase carbohydrate oxidation. Although the detailed mechanisms explaining how and where insulin acts in the body are unclear, insulin stimulation can increase the carbohydrate oxidation throughout the body.
We demonstrated that the impairment of insulin secretion by STZ led to inhibition of the AITC-induced elevation of RER (Figure ), suggesting that the increase in insulin secretion is related to the increase in carbohydrate oxidation due to AITC. However, STZ-treatment could not only impair insulin secretion but also affect normal energy metabolism. Therefore, an abnormality in energy metabolism might be involved in the increased carbohydrate oxidation following administration of AITC. Our results suggest that insulin secretion is vital to the increase in carbohydrate oxidation due to AITC; however, further in-depth studies are required.
Intragastric administration of AITC can enhance pancreatic insulin secretion through direct or indirect pathways. In this study, we showed the direct pathway, in which AITC directly acts on pancreatic islets after absorption in the gastrointestinal tract. However, the indirect pathway occurs through neural or hormonal factors [Citation30–37], and has not been extensively studied. The islets are innervated by both the cholinergic and adrenergic limbs of the autonomic nervous system. The secretion of insulin is stimulated by the vagus nerve and inhibited by the sympathetic nerve [Citation31–33]. In a previous study, we suggested that the vagus nerve was not involved in the increase in carbohydrate oxidation due to AITC [Citation7]. The activation of the sympathetic nerve system is involved in the inhibition of insulin secretion; therefore, the increase in insulin secretion due to AITC may not be related to the autonomic nervous system but sensory nerves or hormonal actions [Citation38]. Further studies are needed to clarify this.
We showed that the enhancement of insulin secretion induced the increase in carbohydrate oxidation (Figures ). Our results suggest that only increases of the insulin secretion could enhance glucose consumption in whole body. In this study, the increase in blood insulin levels due to AITC was observed within 30 min (Figure (a)); however, the increase in carbohydrate oxidation due to AITC was maintained for 90–120 min (Figure (c)). AITC has been reported to activate TRPV1 and TRPA1 and the activation of these channels induces various responses leading to enhancement of energy metabolisms [Citation7,8,25,26,39,40]. Previous studies have also suggested TRPV1 involvement in the enhancement of glucose consumption [Citation7,40] and the development of insulin resistance or sensitivity [Citation8,25,26]. In addition to enhancing insulin secretion, AITC may enhance glucose consumption by increasing adrenalin secretion or improving insulin sensitivity via TRPV1; this may explain why AITC markedly increased carbohydrate oxidation. This implies that TRPV1 may have an important physiological role in glucose controls owing to its involvement in glucose uptake and consumption. Further studies on the close relationship between TRPV1 and glucose metabolism are warranted.
In conclusion, we demonstrated that intragastric administration of AITC increased blood insulin levels in mice and that AITC directly enhanced insulin secretion from isolated mice pancreatic islets. We also showed that these effects involved TRPV1 and suggested that the activation of TRPV1 in islets can enhance insulin secretion from the pancreas. Furthermore, we showed that enhancement of insulin secretion increased carbohydrate oxidation and suggested this enhancement was important to the AITC-mediated increase of carbohydrate oxidation.
Author contributions
N.M., M.K., and T.F. conceived and designed the experiments. N.M., M.K., and H.Y. performed the experiments. N.M. and M.K. analyzed the data. N.M., H.Y., S.M., T.H., K.K. contributed reagents/materials/analysis tools. H.Y., S.M., T.H., K.K., T.N, K.I., and T.F. supervised and provided critical comments to the manuscript. N.M. wrote the paper.
Disclosure statement
No potential conflict of interest was reported by the authors.
Funding
This work was supported by JSPS, Grant-in-Aid for Young Scientists (B) [grant number 24780131].
Acknowledgments
The authors would like to thank Dr. David Julius for Knockout mice.
References
- Caterina MJ, Schumacher MA, Tominaga M, et al. The capsaicin receptor: a heat-activated ion channel in the pain pathway. Nature. 1997;389:816–824.
- Tominaga M, Caterina MJ, Malmberg AB, et al. The cloned capsaicin receptor integrates multiple pain-producing stimuli. Neuron. 1998;21:531–543.10.1016/S0896-6273(00)80564-4
- Story GM, Peier AM, Reeve AJ, et al. ANKTM1, a TRP-like channel expressed in nociceptive neurons, is activated by cold temperatures. Cell. 2003;112:819–829.10.1016/S0092-8674(03)00158-2
- McNamara FN, Randall A, Gunthorpe MJ. Effects of piperine, the pungent component of black pepper, at the human vanilloid receptor (TRPV1). Br J Pharmacol. 2005;144:781–790.10.1038/sj.bjp.0706040
- Macpherson LJ, Geierstanger BH, Viswanath V, et al. The pungency of garlic: activation of TRPA1 and TRPV1 in response to allicin. Curr Biol. 2005;15:929–934.10.1016/j.cub.2005.04.018
- Motter AL, Ahern GP. TRPV1-null mice are protected from diet-induced obesity. FEBS Lett. 2008;582:2257–2262.10.1016/j.febslet.2008.05.021
- Mori N, Kawabata F, Matsumura S, et al. Intragastric administration of allyl isothiocyanate increases carbohydrate oxidation via TRPV1 but not TRPA1 in mice. Am J Physiol Regul Integr Comp Physiol. 2011;300:R1494–R1505.10.1152/ajpregu.00645.2009
- Kang JH, Goto T, Han IS, et al. Dietary capsaicin reduces obesity-induced insulin resistance and hepatic steatosis in obese mice fed a high-fat diet. Obesity (Silver Spring). 2010;18:780–787.10.1038/oby.2009.301
- Tanaka H, Shimaya A, Kiso T, et al. Enhanced insulin secretion and sensitization in diabetic mice on chronic treatment with a transient receptor potential vanilloid 1 antagonist. Life Sci. 2011;88:559–563.10.1016/j.lfs.2011.01.016
- Suri A, Szallasi A. The emerging role of TRPV1 in diabetes and obesity. Trends Pharmacol Sci. 2008;29:29–36.10.1016/j.tips.2007.10.016
- Jordt SE, Bautista DM, Chuang HH, et al. Mustard oils and cannabinoids excite sensory nerve fibres through the TRP channel ANKTM1. Nature. 2004;427:260–265.10.1038/nature02282
- Everaerts W, Gees M, Alpizar YA, et al. The capsaicin receptor TRPV1 is a crucial mediator of the noxious effects of mustard oil. Curr Biol. 2011;21:316–321.10.1016/j.cub.2011.01.031
- Mori N, Kurata M, Yamazaki H, et al. Intragastric administration of allyl isothiocyanate reduces hyperglycemia in intraperitoneal glucose tolerance test (IPGTT) by enhancing blood glucose consumption in mice. J Nutr Sci Vitaminol (Tokyo). 2013;59:56–63.10.3177/jnsv.59.56
- Akiba Y, Kato S, Katsube K, et al. Transient receptor potential vanilloid subfamily 1 expressed in pancreatic islet beta cells modulates insulin secretion in rats. Biochem Biophys Res Commun. 2004;321:219–225.10.1016/j.bbrc.2004.06.149
- Caterina MJ, Leffler A, Malmberg AB, et al. Impaired nociception and pain sensation in mice lacking the capsaicin receptor. Science. 2000;288:306–313.10.1126/science.288.5464.306
- Bautista DM, Jordt SE, Nikai T, et al. TRPA1 mediates the inflammatory actions of environmental irritants and proalgesic agents. Cell. 2006;124:1269–1282.10.1016/j.cell.2006.02.023
- Zawalich WS, Yamazaki H, Zawalich KC. Biphasic insulin secretion from freshly isolated or cultured, perifused rodent islets: comparative studies with rats and mice. Metabolism. 2008;57:30–39.10.1016/j.metabol.2007.07.020
- Albano JD, Ekins RP, Maritz G, et al. A sensitive, precise radioimmunoassay of serum insulin relying on charcoal separation of bound and free hormone moieties. Acta Endocrinol (Copenh). 1972;70:487–509.
- Brusewitz G, Cameron BD, Chasseaud LF, et al. The metabolism of benzyl isothiocyanate and its cysteine conjugate. Biochem J. 1977;162:99–107.10.1042/bj1620099
- Frayn KN. Calculation of substrate oxidation rates in vivo from gaseous exchange. J Appl Physiol. 1983;55:628–634.
- Borghoff SJ, Birnbaum LS. Age-related changes in the metabolism and excretion of allyl isothiocyanate. A model compound for glutathione conjugation. Drug Metab Dispos. 1986;14:417–422.
- Bollard M, Stribbling S, Mitchell S, et al. The disposition of allyl isothiocyanate in the rat and mouse. Food Chem Toxicol. 1997;35:933–943.10.1016/S0278-6915(97)00103-8
- Ioannou YM, Burka LT, Matthews HB. Allyl isothiocyanate: comparative disposition in rats and mice. Toxicol Appl Pharmacol. 1984;75:173–181.10.1016/0041-008X(84)90199-6
- Gram DX, Ahrén B, Nagy I, et al. Capsaicin-sensitive sensory fibers in the islets of Langerhans contribute to defective insulin secretion in Zucker diabetic rat, an animal model for some aspects of human type 2 diabetes. Eur J Neurosci. 2007;25:213–223.10.1111/j.1460-9568.2006.05261.x
- Razavi R, Chan Y, Afifiyan FN, et al. TRPV1+ sensory neurons control beta cell stress and islet inflammation in autoimmune diabetes. Cell. 2006;127:1123–1135.10.1016/j.cell.2006.10.038
- Diaz-Garcia CM, Morales-Lazaro SL, Sanchez-Soto C, et al. Role for the TRPV1 channel in insulin secretion from pancreatic beta cells. J Membr Biol. 2014;247:479–491.10.1007/s00232-014-9658-8
- Malaisse WJ, Malaisse-Lagae F. The role of cyclic AMP in insulin release. Experientia. 1984;40:1068–1075.10.1007/BF01971453
- Sharp GW. The adenylate cyclase-cyclic AMP system in islets of langerhans and its role in the control of insulin release. Diabetologia. 1979;16:287–296.10.1007/BF01223617
- Malenczyk K, Girach F, Szodorai E, et al. A TRPV1-to-secretagogin regulatory axis controls pancreatic beta-cell survival by modulating protein turnover. EMBO J. 2017;36:2107–2125.10.15252/embj.201695347
- Roy MW, Lee KC, Jones MS, et al. Neural control of pancreatic insulin and somatostatin secretion. Endocrinology. 1984;115:770–775.10.1210/endo-115-2-770
- Woods SC, Porte D Jr. Neural control of the endocrine pancreas. Physiol Rev. 1974;54:596–619.
- Kurose T, Seino Y, Nishi S, et al. Mechanism of sympathetic neural regulation of insulin, somatostatin, and glucagon secretion. Am J Physiol. 1990;258:E220–227.
- Nishi S, Seino Y, Ishida H, et al. Vagal regulation of insulin, glucagon, and somatostatin secretion in vitro in the rat. J Clin Invest. 1987;79:1191–1196.10.1172/JCI112936
- Andersen DK, Elahi D, Brown JC, et al. Oral glucose augmentation of insulin secretion. Interactions of gastric inhibitory polypeptide with ambient glucose and insulin levels. J Clin Invest. 1978;62:152–161.10.1172/JCI109100
- Dupre J, Ross SA, Watson D, et al. Stimulation of insulin secretion by gastric inhibitory polypeptide in man. J Clin Endocrinol Metab. 1973;37:826–828.10.1210/jcem-37-5-826
- Zawalich WS. Synergistic impact of cholecystokinin and gastric inhibitory polypeptide on the regulation of insulin secretion. Metabolism. 1988;37:778–781.10.1016/0026-0495(88)90014-5
- Zawalich WS, Diaz VA. Prior cholecystokinin exposure sensitizes islets of Langerhans to glucose stimulation. Diabetes. 1987;36:118–122.10.2337/diab.36.1.118
- Ahren B. Autonomic regulation of islet hormone secretion–implications for health and disease. Diabetologia. 2000;43:393–410.
- Riera CE, Huising MO, Follett P, et al. TRPV1 pain receptors regulate longevity and metabolism by neuropeptide signaling. Cell. 2014;157:1023–1036.10.1016/j.cell.2014.03.051
- Kyle Palmer RK, Lunn CA. TRP channels as targets for therapeutic intervention in obesity: focus on TRPV1 and TRPM5. Curr Top Med Chem. 2013;13:247–257.10.2174/15680266113139990089