Abstract
Flavonoids are distributed across the plant kingdom and have attracted substantial attention owing to their potential benefits for human health. Several studies have demonstrated that flavonoids prenylation enhances various biological activities, suggesting an attractive tool for developing functional foods. This review provides an overview of the current knowledge on how prenylation influences the biological activity and bioavailability of flavonoids. The enhancement effect of prenylation on the biological activities of dietary flavonoids in mammals was demonstrated by comparing the effect of 8-prenyl naringenin (8PN) with that of parent naringenin in the prevention of disuse muscle atrophy in mice. This enhancement results from higher muscular accumulation of 8PN than naringenin. As to bioavailability, despite the lower absorption of 8-prenyl quercetin (8PQ) compared with quercetin, higher 8PQ accumulation was found in the liver and kidney. These data imply that prenylation interferes with the elimination of flavonoids from tissues.
Graphical abstract
This review provides current knowledge of how prenylation influences the biological activity and bioavailability of dietary flavonoids.
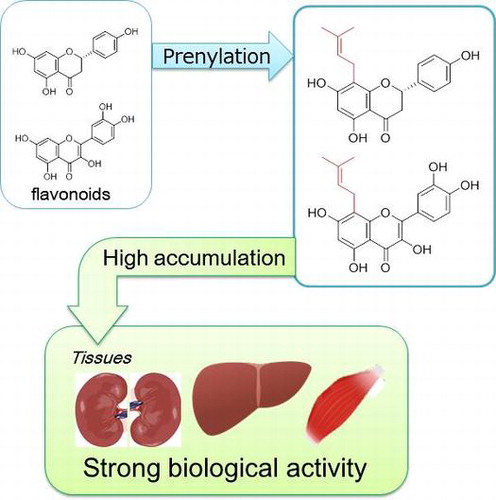
Flavonoids, which have a diphenyl propane structure, are synthesized as plant secondary metabolites. The prenyl group, C5 isoprene (dimethylallyl) unit(s), binds to different positions of the aromatic ring of flavonoids through the action of prenyl transferase [Citation1]. These flavonoids are predominantly C-prenylated, whereas a few studies have shown O-prenylated substituents [Citation2]. More than 1000 species of prenyl flavonoids were isolated and identified from plants classified as leguminous, moraceous, and others [Citation2]. Some of these plants are used as food ingredients or in traditional Oriental medicines. Prenyltransferases are cloned from edible plants, including Humulus lupulus (hops), Glycine max (soy bean), Citrus limon (lemon), and Lupinus albus (lupine) [Citation3–6]. Xanthohumol (XN; 2′,4,4′-trihydroxy-6′-methoxy-3′-prenylchalcone, Figure ), isoxanthohumol (IX, Figure ), 6-prenyl naringenin (6PN, Figure ), and 8-prenyl naringenin (8PN, Figure ) are found in hops, a key ingredient of beer [Citation7]. 8-Prenyl quercetin (8PQ, Figure ) was found in Desmodium caudatum [Citation8], which acts as a preservative for food storage. Koeduka et al. [Citation9] and Sugiyama et al. [Citation10] developed transgenic plants by metabolic engineering using prenyl transferases for the production of prenyl polyphenols in tomato fruits and legume plants, respectively.
Recent studies have demonstrated that prenyl flavonoids possess strong biological activity in vitro and in vivo; however, the bioavailability of prenyl flavonoids is not yet fully understood. This review focuses on how prenylation enhances the biological activity of flavonoids and moderates their bioavailability, including effects on the intestinal absorption, metabolism, and tissue distribution of flavonoids.
Biological activities of prenyl flavonoids
The anti-oxidant activity of prenyl flavonoids has been demonstrated. For example, Stevens et al. [Citation11] demonstrated that prenyl flavonoids extracted from hops suppressed the oxidation of low-density lipoproteins induced by peroxynitrite. Prenyl flavonoids from the roots of Sophora flavescens showed anti-oxidant activity against DPPH radicals, ABTS radical cations, and peroxy-nitrite and reactive oxygen species [Citation12]. The anti-oxidative activity of XN was also revealed in rats administered CCl4 [Citation13]. Reduction of glutathione as well as increases of thiobarbituric acid reactive substances and H2O2 generation were also observed in the rats administered CCl4. However, XN pre-treatment suppressed these enhancements of oxidative stress markers and also prevented the reductions of the activities of redox-related enzymes such as superoxide dismutase, catalase, glutathione peroxidase, glutathione reductase, and glutathione S-transferase in CCl4-administered rats.
Prenyl flavonoids act as selective estrogen receptor modulators [Citation14]. The position of the prenyl substituent to isoflavone changes the affinity to estrogen receptor α (ERα) or ERβ; e.g. double prenylation at positions 8 and 3′ of genistein showed ERβ-selective activation [Citation15]. Icariin (Figure ), a prenyl flavanone glycoside isolated from Epimedii herba, was shown to promote the osteogenic differentiation of rat bone marrow stromal cells by activating ERα [Citation16].
Prenyl flavanone isolated from Cudrania tricuspidata Bureau [Citation17] and Artocarpus communis [Citation18] showed anti-inflammatory effects. XN suppressed interleukin (IL)-6 production induced by lipopolysaccharide (LPS) in human THP-1 monocytes [Citation19]. XN also reduced the levels of Toll-like receptor 4 (TLR4) protein by interacting with the TLR4 co-receptor myeloid differentiation protein-2. Three prenyl flavonoids, cudraflavone B, pomiferin, and osajin, inhibited IκB-α degradation in the murine macrophage cell line J774.A1 treated with LPS [Citation20]. Chemically synthesized prenylated chalcone suppressed prostaglandin E(2) production induced by LPS in mouse macrophages [Citation21]. The anti-inflammatory effect of 8PQ was investigated in a mouse model of paw edema induced by LPS [Citation22]. Pretreatment with 8PQ (1 μM/kg body weight) for 4 days significantly reduced the LPS-induced paw thickness during the experiment without any side effects noted from the 8PQ treatment. The concentration of IL-6 in the serum from 8PQ-pretreated mice induced with LPS was lower than that of the mice treated with LPS alone.
Several studies have also demonstrated other biological activities of prenyl flavonoids, such as anti-cancer [Citation23,24], anti-bacterial [Citation25], anti-virus [Citation26], and anti-phospholipase phosphorylation [Citation27] activities.
Prenylation enhances the biological activities of flavonoids
Recently, there has been much research focus on the biological activities of prenyl flavonoids, which are stronger than those of non-prenyl flavonoids (Table ).
Table 1. Prenylation enhances biological activities of parent flavonoids.
The prenylation of flavonoids was shown to enhance the inhibitory effects of flavonoids on some enzymatic activities. For example, prenyl flavonoids from Sophora flavescens inhibited tyrosinase activity [Citation28,29]. The tyrosinase inhibitory activity of luteolin was increased by prenylation at the 3-position of luteolin [Citation30]. Prenylation at the 6-position of apigenin also enhanced the inhibitory effect on melanin biosynthesis in B16 melanoma cells [Citation31]. Moreover, an increase in the length of prenylation to quercetin, genistein, and chalcone resulted in enhancement of an inhibitory effect of parent polyphenols on α-glucosidase activity [Citation32]. The prenyl chain might interact with the active site of α-glucosidase to exert an inhibitory effect. Certain flavonoids act as inhibitors of mitogen-activated protein kinase (MAPK). Prenylation to quercetin at the 8-position (8PQ) enhanced the inhibitory effect of quercetin on SEK1-JNK1/2 phosphorylation and MEK1/2-ERK1/2 phosphorylation induced by LPS in mouse LAW264.7 macrophages [Citation22]. Hisanaga et al. [Citation22] further suggested that 8PQ showed a stronger interaction with SEK1 and MEK1 than with quercetin to inhibit the activities of these kinases.
Flavonoids with prenyl groups improved the antioxidant activity in HepG2 cells [Citation33]. Kazinol E, which has three prenyl groups and a catechol structure, had a greater suppressive effect on oxidative stress than other kazinols harboring only a few or no prenyl groups. By contrast, Hošek et al. [Citation20] suggested that the catechol moiety in prenyl flavonoids is crucial for the anti-oxidative activity in the murine macrophage cell line J774.A1. Taken together, these findings suggest that the prenyl group might not improve the anti-oxidative activity itself but would instead contribute to the interaction at the target site (biomembrane and/or protein) to reveal the effect due to its elevation of hydrophobicity.
8PN and 6-(1,1-dimethylallyl)naringenin showed stronger estrogenic activity than the parent compound naringenin in MCF-7 cells [Citation34]. It was further demonstrated that prenyl chains interact with a hydrophobic pocket in the estrogen receptors using an in silico modeling experiment [Citation35]. 8PN can modulate physiological responses in hormone-responsive cells [Citation36], and has a stronger effect on osteoblast differentiation and osteogenic function in cultured rat calvarial osteoblasts. In addition, induction of apoptosis of mature osteoclasts by 8PN was greater than that observed by naringenin.
8PN has also been shown to exert beneficial effects on muscle maintenance. Our study demonstrated that 8PN improved recovery from muscle atrophy induced by immobilization [Citation37]. Ankle immobilization was used to induce disuse muscle atrophy in mice, and then 8PN feeding was started. At 20 days into the recovery period, the weight of the skeletal muscle from the 8PN-fed group was higher than that from the control group. 8PN maintained the level of Akt phosphorylation during the recovery period, but did not increase the serum insulin-like growth factor 1 (IGF-1) concentration. In addition, a cultured cell study clearly indicated that 8PN activated the PI3K/Akt/P70S6K1 phosphorylation pathway, which promotes protein synthesis in the skeletal muscle. This activation was diminished by fulvestrant, an inhibitor of estrogen receptors. In addition, 8PN was shown to promote muscle recovery from disuse muscle atrophy in ovariectomized female mice, which was also observed in ovariectomized female mice with pellets releasing 17β-estradiol. These data suggest that activation of muscle recovery by 8PN is associated with its estrogenic activity. Another study demonstrated that the suppressive effect of 8PN on disuse muscle atrophy was related to the significant accumulation of 8PN in the skeletal muscle of mice [Citation38]. The sciatic nerve in the leg of each mouse was cut to induce immobilization for the development of disuse muscle atrophy in the gastrocnemius muscle (i.e. denervation). This muscle atrophy was completely suppressed by the pre-intake of 8PN or hops (an 8PN and its structure-related prenyl flavonoids supplier) for 14 days. The suppressive effect of 8PN was not accompanied by changes in the water and protein proportions in the muscle. In addition, 8PN did not affect the weight of the normal muscle. Although suppression of the IGF-1/mTOR/Akt pathway by denervation resulted in the induction of atrogin-1, a muscle atrophy-specific ubiquitin ligase, 8PN supplementation completely suppressed atrogin-1 expression in the denervated muscle. On the other hand, feeding of naringenin over the same period had no effect on the atrophy. These results clarified that prenylation provides naringenin with the ability to prevent disuse muscle atrophy. Tissue accumulation in the gastrocnemius muscle and the plasma concentration of 8PN in mice were analyzed and compared to those of naringenin. The total amounts of 8PN (including both aglycones and their conjugated metabolites) was 2.26–6.44 nmol/g tissue [Citation38] (a representative result is shown in Figure ). This level was approximately 10-fold higher than that of naringenin under the same experimental condition. 8PN aglycone was also detected in the gastrocnemius muscle, although no naringenin aglycone was detected. Higher accumulation of 8PN in the gastrocnemius muscle is likely one of the main reasons why 8PN but not naringenin exerted a preventive effect on disuse muscle atrophy. Therefore, the increment of accumulation of flavonoids in the target tissue by prenylation may be the critical factor for enhancement of the in vivo biological activity of flavonoids by prenylation.
Figure 2. High accumulation of prenyl flavonoids in the tissues. Mukai et al. [Citation38,65] demonstrated higher tissue accumulation of prenyl flavonoids by comparison with parent flavonoids. Mice were fed each flavonoid mixed in a diet for more than 2 weeks, and the concentrations were analyzed by high-performance liquid chromatography. (A) Accumulation of 8PN and naringenin in the gastronomic muscle [Citation38], (B) 8PQ and quercetin in the liver and kidney [Citation65]. Data are modified from previous reports [Citation38,65]. Data represent the mean ± S.E (n = 4). Asterisks indicate significant differences analyzed by two-sided Student’s t-test (p < 0.05).
![Figure 2. High accumulation of prenyl flavonoids in the tissues. Mukai et al. [Citation38,65] demonstrated higher tissue accumulation of prenyl flavonoids by comparison with parent flavonoids. Mice were fed each flavonoid mixed in a diet for more than 2 weeks, and the concentrations were analyzed by high-performance liquid chromatography. (A) Accumulation of 8PN and naringenin in the gastronomic muscle [Citation38], (B) 8PQ and quercetin in the liver and kidney [Citation65]. Data are modified from previous reports [Citation38,65]. Data represent the mean ± S.E (n = 4). Asterisks indicate significant differences analyzed by two-sided Student’s t-test (p < 0.05).](/cms/asset/f5a72efc-ca2f-498c-9c91-f3ecdf61b4e7/tbbb_a_1415750_f0002_b.gif)
Bioavailability: absorption, metabolism, and tissue distribution
The biological activity of dietary flavonoids varies depending on their bioavailability, including absorption, metabolism, and tissue distribution. Studies related to the bioavailability of flavonoids have mainly been conducted using non-prenylated flavonoids with a sugar moiety or its aglycone. The sugar moiety of glucosylated flavonoids is removed during absorption in enterocytes [Citation39]. Mullen et al. [Citation40] demonstrated that glucuronic acid, sulfate, acetyl, glutathione, or methyl groups are attached to flavonoids by phase-II metabolism in enterocytes to facilitate an increment in hydrophilicity for entering the blood circulation. Some of these metabolites have also been detected in the lymph circulation [Citation41]. Since the metabolites of flavonoids do not show an organ- or tissue-specific distribution in the body, they are found in various organs and tissues such as the liver, kidney, brain, lung, heart, skin, and muscle [Citation42,43]. ATP-binding cassette (ABC) transporters, including multidrug-resistant protein 2, breast cancer-resistant protein, and P-glycoprotein regulate the excretion of flavonoids from tissue-constituting cells [Citation44–46]. Here, we have attempted to provide an overview of the existing information on the bioavailability of prenyl flavonoids, including their absorption, metabolism, and tissue distribution, in mammals, including humans. This information should be useful for developing functional foods containing prenyl flavonoids.
Absorption of prenyl flavonoids
Aoki et al. [Citation47] demonstrated the pharmacokinetic properties of glabridin, an isoflavane with cyclization of a prenyl chain termed pyran (Figure ), in humans. The maximum concentration of glabridin in plasma was reached at 4 h after administration of a single dose of licorice oil containing glabridin [Citation47]. Although glabridin disappeared from the plasma within a few days after the single dose, continuous administration of the same dose of licorice oil for 2 weeks resulted in a steady-state plasma concentration of glabridin [Citation47]. In a clinical study, volunteers consumed a hop supplement containing 2.04 mg XN, 1.20 mg IX, and 0.1 mg 8PN three times per day for 5 days [Citation48]. The concentrations of XN and IX were determined to be 0.72–17.65 and 3.30–31.50 nM, respectively, in hydrolyzed serum [Citation48]. LeeCole et al. [Citation49] further demonstrated that IX, 8PN, and 6PN were detected as metabolites of XN after the oral intake of XN. The plasma concentration of these metabolites after single ingestion of XN showed two maximal peaks in the time–concentration curve [Citation50]. The second peak, which was detected at approximately 5 h after ingestion, may have been due to the enterohepatic circulation [Citation50]. 8PN showed a third peak at 24 h after ingestion, which was likely derived from further complicated metabolites generating the enterohepatic circulation.
Metabolic transformation of prenyl flavonoids via drug metabolism enzymes
The clinical studies described above demonstrated that glucuronide was the most abundant metabolite in both the plasma and urine. In addition, phase-I enzymes also contribute to the metabolism of prenyl flavonoids, accounting for approximately 10% of the total metabolites [Citation48]. These metabolic reactions occurring during absorption and circulation were also clarified in rodent or in vitro experiments. Icariin, a prenyl flavonoid glucoside in Epimedium spp., undergoes hydrolysis during enterohepatic circulation, resulting in the formation of an aglycone, icarinoside II [Citation51]. Lactase phlorizin hydrolase (LPH) and β-glucosidase would hydrolyze the Epimedium flavonoids such as icariin to aglycone. Rodent studies also demonstrated that glucuronide conjugation was identified as a major metabolic event after the oral ingestion of prenyl flavonoids derived from Epimedium, licorice, or hops [Citation52–54]. A cell culture study further investigated the hepatic and intestinal metabolism of 8PN [Citation55]. 8PN showed passive diffusion during absorption to Caco-2 cells, a model of enterocytes. 8PN underwent phase-II metabolism in Caco-2 cells, with approximately 54 and 3% of the 8PN treated to the cells ultimately being converted to glucuronides and sulfates, respectively. The incubation of 8PN with various UDP-glucuronosyl transferases indicated that the isozymes 1A1, 1A6, 1A8, and 1A9 were responsible for the glucuronidation of 8PN. Indeed, this author detected not only the 7-O-glucuronide of 8PN but also the cis- and trans-8PN alcohols as metabolites of 8PN in hepatocytes. CYP2C19 converted 8PN to both the cis- and trans-alcohols of the prenyl side chain of 8PN, and CYP2C8 was found to catalyze the formation of the trans-alcohol of the prenyl group of 8PN [Citation56].
Biotransformation of prenyl flavonoids by intestinal microflora
The intestinal microflora plays an important role in the biotransformation of prenyl flavonoids. Icariin (Figure ) was converted to icarinoside II, icaritin (Figure ), and desmethylicaritin by intestinal microflora, including Streptococcus sp., Enterococcus sp., and Blautia sp. [Citation57]. Liu et al. [Citation58] demonstrated that 8PN was generated after ingestion of XN in humans. XN was spontaneously converted to IX during heating in beer production, and then IX further underwent enzymatic O-demethylation to 8PN by CYP1A2 [Citation56] or via intestinal microflora metabolism (Figure ) [Citation59,60]. Eubacterium limosum resulted in 90% conversion of IX to 8PN in vitro with pure incubation [Citation61]. 8PN production in humans exhibited individual variation depending on the intestinal microflora composition. Possemiers et al. [Citation59] carried out the in vitro incubation of 51 fecal samples from female volunteers with IX, and classified the ability of 8PN production as poor (32 of 51), moderate (11 of 51), and strong (8 of 51) based on the level of 8PN production from IX.
Figure 3. Conversion of XN to 8PN [Citation56,59,60].
![Figure 3. Conversion of XN to 8PN [Citation56,59,60].](/cms/asset/1e2d8618-1022-4c76-8e85-f75027a68019/tbbb_a_1415750_f0003_b.gif)
Tissue distribution of prenyl flavonoids
A few studies have evaluated the tissue distribution of prenyl flavonoids in rodent animal models [Citation62–64]. The intraperitoneal injection of icariin to mice resulted in icariin distribution into the spleen, liver, lung, kidney, heart, and brain [Citation64]. The maximum concentrations of icariin in each tissue was in the order spleen > lung > liver > kidney > heart > brain. However, with oral injection of icaritin to rats, the maximum concentrations of icaritin in each tissue showed a different order: liver > spleen > muscle > lung > kidney > heart [Citation63]. The maximum concentration of icaritin aglycone in each tissue was detected within 1 h. The liver showed the highest accumulation of icaritin aglycone, whereas most of the icaritin glucuronide was detected in the kidney [Citation63]. These results imply that icaritin aglycone could undergo glucuronidation in the liver and then the glucuronides would excrete into the urine. 4-Hydroxyderricin, a prenyl chalcone from Angelica keiskei, was found to be distributed in the liver, kidney, spleen, skeletal muscle, and adipose tissue after single ingestion in mice [Citation62]. A higher amount of 4-hydroxyderricin was detected in the adipose tissue than the other tissues. Thus, the adipose tissue could be the storage site of prenyl flavonoids because prenyl flavonoids are more hydrophobic than their parent flavonoids. Only one clinical study was carried out by Bolca et al. [Citation48], who demonstrated that XN and IX were detected in the breast tissue after hops supplementation in women.
Prenylation reduces intestinal absorption and enhances tissue accumulation of dietary flavonoids
The author’s group carried out a study to clarify the effect of prenylation on the bioavailability of flavonoids, since there were few studies related to the effect of prenylation on the bioavailability of flavonoids compared with studies on their biological activities [Citation65]. 8PQ was applied for this study because the bioavailability, metabolism, and tissue distribution properties of quercetin are well known. We determined the tissue distribution of 8PQ in mice fed 8PQ for 2 weeks (Figure ; note that the data were modified from the previous report [Citation65]). The tissue accumulation of the subtotal of 8PQ and its metabolites in the liver and kidneys was at least 10 times higher than that of the parent quercetin. However, the plasma and lymph concentrations of 8PQ after single ingestion, as an index of intestinal absorption, were lower than those of quercetin. Results showing the same trend were obtained from a study using 8PN and naringenin [Citation38]. Konishi et al. [Citation66] also demonstrated that prenylation to p-coumaric acid (artepillinC) lowered the intestinal absorption compared with the parent p-coumaric acid. Moreover, an experiment on intestinal permeability using Caco-2 cells demonstrated that 8PQ permeated to the basolateral side at an extremely low level, although the cellular uptake of 8PQ in Caco-2 cells was higher than that of quercetin [Citation65]. This result is supported by a study showing that XN was hardly excreted from Caco-2 cells because of the trap created by cytosolic proteins in the cells [Citation67]. Therefore, it is likely that prenyl flavonoids accumulated at a higher level than the parent flavonoids despite their lower intestinal absorption. In order to clarify the reason for this apparent contradiction, an experiment was carried out on the uptake into murine skeletal muscle C2C12 myoblast cells as an organ model. The results showed that the cellular uptake of 8PQ and 8PN was up to 10-times greater than that of the parent flavonoids; moreover, the accumulation persisted for a longer time than that of the non-prenylated forms. In addition, 8PQ, unlike quercetin, is hardly excreted outside the cell at all via the ABC transporter. Although glucuronidation to prenyl flavonoids is necessary for their excretion from cells, an increase of the number of prenyl groups will decrease the rate of glucuronidation [Citation68]. This could be one of the reasons explaining why prenyl flavonoids do not readily excrete from tissues. Consequently, prenylation enhances the tissue accumulation of flavonoids by modulating the balance between the absorption and excretion of flavonoids in tissue-constituting cells.
Conclusion and future perspectives
Proposed scheme of the accumulation of prenyl flavonoids in the tissues to exert strong biological activity in vivo is shown in Figure . Prenylation lowers the transport of flavonoids from enterocytes to the internal circulation, elevates its incorporation from the circulation to tissue-constituting cells, and slows its efflux from tissue-constituting cells. Prenylation facilitates the accumulation in target tissues to exert strong biological activity in the case of continuous, long-term dietary intake.
Figure 4. Prenylation enhances the biological activity of dietary flavonoids by increasing their bioaccumulation.
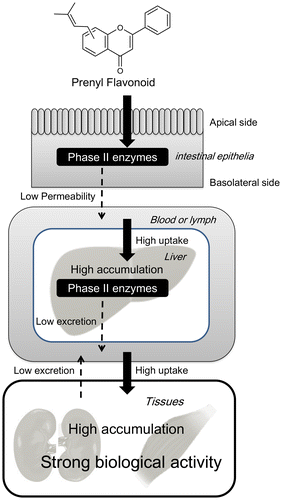
Prenyl flavonoids will be used as components of functional foods because of their potent biological activity. For this purpose, it is necessary to verify the biological activity based on the bioavailability in clinical studies. Differences in metabolism between individuals, which were notably observed in the study of microflora, may influence the biological activity. The rodent study implied that prenyl flavonoids are cleared from the tissues within a few days after a single dose, indicating that they could not be stored in vivo. However, dramatically high accumulation of prenyl flavonoids in several tissues after long-term ingestion was observed. Since this phenomenon raises concerns of the potential side effects of prenyl flavonoids, it is necessary to investigate the clearance of prenyl flavonoids from the tissues into the urine and feces after long-term ingestion. Clarification of the balance between absorption and excretion would help to determine how much and for how long prenyl flavonoids should be taken for exerting a beneficial effect for human health without any harmful side effects.
Funding
This work was supported by the Program for the Promotion of Basic and Applied Researches for Innovations in Bio-oriented Industry (Japan); and JSPS KAKENHI [grant number 26892020] to R. Mukai and [grant number 16K12721] to R. Mukai.
Disclosure statement
No potential conflict of interest was reported by the author.
Acknowledgements
This review paper is a summary of the JSBBA Award for Young Scientists (The Japan Society for Bioscience, Biotechnology, and Agrochemistry, Japan). This study was mainly carried out at Tokushima University. Sincere thanks are owed to all of the staff and students who helped with this study. Particular thanks are due to Prof Junji Terao (Konan Women’s University) who proposed the topic of this study, and provided abundant guidance and encouragement. This study was accomplished by collaboration with Prof Takeshi Nikawa (Toskuhima University), Dr Hisao Nemoto (Tokushima University), and Prof De-Xing Hou (Kagoshima University). Thanks are also owed to Prof Hitoshi Ashida (Kobe University) and emeritus Prof Naoki Higashi (Tokyo Metropolitan University) for their guidance over many years since my student days.
Notes
This review was written in response to the author’s receipt of the JSBBA Award for Young Scientists in 2017.
References
- Yazaki K, Sasaki K, Tsurumaru Y. Prenylation of aromatic compounds, a key diversification of plant secondary metabolites. Phytochemistry. 2009;70:1739–1745.10.1016/j.phytochem.2009.08.023
- Barron D, Ibrahim RK. Isoprenylated flavonoids – a survey. Phytochemistry. 1996;43:921–982.10.1016/S0031-9422(96)00344-5
- Shen G, Huhman D, Lei Z, et al. Characterization of an isoflavonoid-specific prenyltransferase from Lupinus albus. Plant Physiol. 2012;159:70–80.10.1104/pp.112.195271
- Munakata R, Inoue T, Koeduka T, et al. Molecular cloning and characterization of a geranyl diphosphate-specific aromatic prenyltransferase from lemon. Plant Physiol. 2014;166:80–90.10.1104/pp.114.246892
- Li H, Ban Z, Qin H, et al. A heteromeric membrane-bound prenyltransferase complex from hop catalyzes three sequential aromatic prenylations in the bitter acid pathway. Plant Physiol. 2015;167:650–659.10.1104/pp.114.253682
- Akashi T, Sasaki K, Aoki T, et al. Molecular cloning and characterization of a cDNA for pterocarpan 4-dimethylallyltransferase catalyzing the key prenylation step in the biosynthesis of glyceollin, a soybean phytoalexin. Plant Physiol. 2009;149:683–693.
- Stevens JF, Page JE. Xanthohumol and related prenylflavonoids from hops and beer: to your good health!. Phytochemistry. 2004;65:1317–1330.10.1016/j.phytochem.2004.04.025
- Wu Y, Luo Q, Sun C, et al. Chemical constituents contained in Desmodium caudatum. Zhongguo Zhong Yao Za Zhi. 2012;37:1788–1792.
- Koeduka T, Shitan N, Kumano T, et al. Production of prenylated flavonoids in tomato fruits expressing a prenyltransferase gene from Streptomyces coelicolor A3(2). Plant Biol (Stuttg). 2011;13:411–415.
- Sugiyama A, Linley PJ, Sasaki K, et al. Metabolic engineering for the production of prenylated polyphenols in transgenic legume plants using bacterial and plant prenyltransferases. Metab Eng. 2011;13:629–637.10.1016/j.ymben.2011.07.003
- Stevens JF, Miranda CL, Frei B, et al. Inhibition of peroxynitrite-mediated LDL oxidation by prenylated flavonoids: the alpha, beta-unsaturated keto functionality of 2′-hydroxychalcones as a novel antioxidant pharmacophore. Chem. Res. Toxicol. 2003;16:1277–1286.10.1021/tx020100d
- Jung HA, Jeong DM, Chung HY, et al. Re-evaluation of the antioxidant prenylated flavonoids from the roots of Sophora flavescens. Biol Pharm Bull. 2008;31:908–915.10.1248/bpb.31.908
- Pinto C, Duque AL, Rodríguez-Galdón B, et al. Xanthohumol prevents carbon tetrachloride-induced acute liver injury in rats. Food Chem Toxicol. 2012;50:3405–3412.10.1016/j.fct.2012.07.035
- Simons R, Gruppen H, Bovee TF, et al. Prenylated isoflavonoids from plants as selective estrogen receptor modulators (phytoSERMs). Food Funct. 2012;3:810–827.10.1039/c2fo10290 k
- Djiogue S, Njamen D, Halabalaki M, et al. Estrogenic properties of naturally occurring prenylated isoflavones in U2OS human osteosarcoma cells: structure-activity relationships. J Steroid Biochem Mol Biol. 2010;120:184–191.10.1016/j.jsbmb.2010.04.014
- Wei Q, Zhang J, Hong G, et al. Icariin promotes osteogenic differentiation of rat bone marrow stromal cells by activating the ERalpha-Wnt/beta-catenin signaling pathway. Biomed Pharmacother. 2016;84:931–939.10.1016/j.biopha.2016.09.107
- Kim DC, Yoon CS, Quang TH, et al. Prenylated flavonoids from Cudrania tricuspidata suppress lipopolysaccharide-induced neuroinflammatory activities in BV2 microglial cells. Int J Mol Sci. 2016;17:255.10.3390/ijms17020255
- Han AR, Kang YJ, Windono T, et al. Prenylated flavonoids from the heartwood of Artocarpus communis with inhibitory activity on lipopolysaccharide-induced nitric oxide production. J Nat Prod. 2006;69:719–721.10.1021/np0600346
- Peluso MR, Miranda CL, Hobbs DJ, et al. Xanthohumol and related prenylated flavonoids inhibit inflammatory cytokine production in LPS-activated THP-1 monocytes: structure-activity relationships and in silico binding to myeloid differentiation protein-2 (MD-2). Planta Med. 2010;76:1536–1543.10.1055/s-0029-1241013
- Hošek J, Toniolo A, Neuwirth O, et al. Prenylated and geranylated flavonoids increase production of reactive oxygen species in mouse macrophages but inhibit the inflammatory response. J Nat Prod. 2013;76:1586–1591.
- Rullah K, Mohd Aluwi MF, Yamin BM, et al. Inhibition of prostaglandin E(2) production by synthetic minor prenylated chalcones and flavonoids: synthesis, biological activity, crystal structure, and in silico evaluation. Bioorg Med Chem Lett. 2014;24:3826–3834.10.1016/j.bmcl.2014.06.061
- Hisanaga A, Mukai R, Sakao K, et al. Anti-inflammatory effects and molecular mechanisms of 8-prenyl quercetin. Mol Nutr Food Res. 2016;60:1020–1032.10.1002/mnfr.201500871
- Akihisa T, Motoi T, Seki A, et al. Cytotoxic activities and anti-tumor-promoting effects of microbial transformation products of prenylated chalcones from Angelica keiskei. Chem Biodivers. 2012;9:318–330.10.1002/cbdv.v9.2
- Venturelli S, Burkard M, Biendl M, et al. Prenylated chalcones and flavonoids for the prevention and treatment of cancer. Nutrition. 2016;32:1171–1178.10.1016/j.nut.2016.03.020
- Sasaki H, Kashiwada Y, Shibata H, et al. Prenylated flavonoids from Desmodium caudatum and evaluation of their anti-MRSA activity. Phytochemistry. 2012;82:136–142.10.1016/j.phytochem.2012.06.007
- Grienke U, Richter M, Walther E, et al. Discovery of prenylated flavonoids with dual activity against influenza virus and Streptococcus pneumoniae. Sci Rep. 2016;6:252.10.1038/srep27156
- Lee YM, Hsieh KH, Lu WJ, et al. Xanthohumol, a prenylated flavonoid from hops (Humulus lupulus), prevents platelet activation in human platelets. Evid Based Complement Alternat Med. 2012;2012:852362.
- Kim SJ, Son KH, Chang HW, et al. Tyrosinase inhibitory prenylated flavonoids from Sophora flavescens. Biol Pharm Bull. 2003;26:1348–1350.10.1248/bpb.26.1348
- Son JK, Park JS, Kim JA, et al. Prenylated flavonoids from the roots of Sophora flavescens with tyrosinase inhibitory activity. Planta Med. 2003;69:559–561.
- Arung ET, Shimizu K, Tanaka H, et al. 3-Prenyl luteolin, a new prenylated flavone with melanin biosynthesis inhibitory activity from wood of Artocarpus heterophyllus. Fitoterapia. 2010;81:640–643.10.1016/j.fitote.2010.03.011
- Arung ET, Shimizu K, Kondo R. Structure-activity relationship of prenyl-substituted polyphenols from Artocarpus heterophyllus as inhibitors of melanin biosynthesis in cultured melanoma cells. Chem Biodivers. 2007;4:2166–2171.10.1002/(ISSN)1612-1880
- Sun H, Li Y, Zhang X, et al. Synthesis, alpha-glucosidase inhibitory and molecular docking studies of prenylated and geranylated flavones, isoflavones and chalcones. Bioorg Med Chem Lett. 2015;25:4567–4571.10.1016/j.bmcl.2015.08.059
- Kim AY, Lee CG, Lee DY, et al. Enhanced antioxidant effect of prenylated polyphenols as Fyn inhibitor. Free Radic Biol Med. 2012;53:1198–1208.10.1016/j.freeradbiomed.2012.06.039
- Kretzschmar G, Zierau O, Wober J, et al. Prenylation has a compound specific effect on the estrogenicity of naringenin and genistein. J Steroid Biochem Mol Biol. 2010;118:1–6.10.1016/j.jsbmb.2009.08.005
- van de Schans MG, Ritschel T, Bovee TF, et al. Involvement of a hydrophobic pocket and helix 11 in determining the modes of action of prenylated flavonoids and isoflavonoids in the human estrogen receptor. ChemBioChem. 2015;16:2668–2677.10.1002/cbic.201500343
- Ming LG, Lv X, Ma XN, et al. The prenyl group contributes to activities of phytoestrogen 8-prenynaringenin in enhancing bone formation and inhibiting bone resorption in vitro. Endocrinology. 2013;154:1202–1214.10.1210/en.2012-2086
- Mukai R, Horikawa H, Lin PY, et al. 8-prenylnaringenin promotes recovery from immobilization-induced disuse muscle atrophy through activation of the Akt phosphorylation pathway in mice. Am J Physiol Regul Integr Comp Physiol. 2016;311:R1022–R1031.10.1152/ajpregu.00521.2015
- Mukai R, Horikawa H, Fujikura Y, et al. Prevention of disuse muscle atrophy by dietary ingestion of 8-prenylnaringenin in denervated mice. PLoS ONE. 2012;7:e45048.10.1371/journal.pone.0045048
- Day AJ, Gee JM, DuPont MS, et al. Absorption of quercetin-3-glucoside and quercetin-4′-glucoside in the rat small intestine: the role of lactase phlorizin hydrolase and the sodium-dependent glucose transporter. Biochem Pharmacol. 2003;65:1199–1206.10.1016/S0006-2952(03)00039-X
- Mullen W, Graf BA, Caldwell ST, et al. Determination of flavonol metabolites in plasma and tissues of rats by HPLC-radiocounting and tandem mass spectrometry following oral ingestion of [2-(14)C]quercetin-4′-glucoside. J Agric Food Chem. 2002;50:6902–6909.10.1021/jf020598p
- Murota K, Terao J. Quercetin appears in the lymph of unanesthetized rats as its phase II metabolites after administered into the stomach. FEBS Lett. 2005;579:5343–5346.10.1016/j.febslet.2005.08.060
- Takumi H, Mukai R, Ishiduka S, et al. Tissue distribution of hesperetin in rats after a dietary intake. Biosci Biotechnol Biochem. 2011;75:1608–1610.10.1271/bbb.110157
- Bieger J, Cermak R, Blank R, et al. Tissue distribution of quercetin in pigs after long-term dietary supplementation. J Nutrition. 2008;138:1417–1420.
- Mukai R, Satsu H, Shimizu M, et al. Inhibition of P-glycoprotein enhances the suppressive effect of kaempferol on transformation of the aryl hydrocarbon receptor. Biosci Biotechnol Biochem. 2009;73:1635–1639.10.1271/bbb.90145
- Vaidyanathan JB, Walle T. Cellular uptake and efflux of the tea flavonoid (-)epicatechin-3-gallate in the human intestinal cell Line Caco-2. J Pharmacol Exp Ther. 2003;307:745–752.10.1124/jpet.103.054296
- Brand W, van der Wel PA, Rein MJ, et al. Metabolism and transport of the citrus flavonoid hesperetin in Caco-2 cell monolayers. Drug Metab Dispos. 2008;36:1794–1802.10.1124/dmd.107.019943
- Aoki F, Nakagawa K, Kitano M, et al. Clinical safety of Licorice Flavonoid Oil (LFO) and pharmacokinetics of glabridin in healthy humans. J Am Coll Nutr. 2007;26:209–218.10.1080/07315724.2007.10719603
- Bolca S, Li J, Nikolic D, et al. Disposition of hop prenylflavonoids in human breast tissue. Mol Nutr Food Res. 2010;54:S284–S294.10.1002/mnfr.v54.7s
- Legette L, Karnpracha C, Reed RL, et al. Human pharmacokinetics of xanthohumol, an antihyperglycemic flavonoid from hops. Mol Nutr Food Res. 2014;58:248–255.10.1002/mnfr.v58.2
- van Breemen RB, Yuan Y, Banuvar S, et al. Pharmacokinetics of prenylated hop phenols in women following oral administration of a standardized extract of hops. Mol Nutr Food Res. 2014;58:1962–1969.10.1002/mnfr.v58.10
- Zhao H, Fan M, Fan L, et al. Liquid chromatography-tandem mass spectrometry analysis of metabolites in rats after administration of prenylflavonoids from Epimediums. J Chromatogr B. 2010;878:1113–1124.10.1016/j.jchromb.2010.03.023
- Zhou J, Ma YH, Zhou Z, et al. Intestinal absorption and metabolism of epimedium flavonoids in osteoporosis rats. Drug Metab Dispos. 2015;43:1590–1600.10.1124/dmd.115.064386
- Xiang C, Qiao X, Wang Q, et al. From single compounds to herbal extract: a strategy to systematically characterize the metabolites of licorice in rats. Drug Metab Dispos. 2011;39:1597–1608.10.1124/dmd.111.038695
- Martinez SE, Davies NM. Enantiospecific pharmacokinetics of isoxanthohumol and its metabolite 8-prenylnaringenin in the rat. Mol Nutr Food Res. 2015;59:1674–1689.10.1002/mnfr.201500118
- Nikolic D, Li Y, Chadwick LR, et al. In vitro studies of intestinal permeability and hepatic and intestinal metabolism of 8-prenylnaringenin, a potent phytoestrogen from hops (Humulus lupulus L.). Pharmaceut Res. 2006;23:864–872.10.1007/s11095-006-9902-8
- Guo J, Nikolic D, Chadwick LR, et al. Identification of human hepatic cytochrome P450 enzymes involved in the metabolism of 8-prenylnaringenin and isoxanthohumol from hops (Humulus lupulus l.). Drug Metab Dispos. 2006;34:1152–1159.10.1124/dmd.105.008250
- Wu H, Kim M, Han J. Icariin metabolism by human intestinal microflora. Molecules. 2016;21:1158.10.3390/molecules21091158
- Liu M, Hansen PE, Wang G, et al. Pharmacological profile of xanthohumol, a prenylated flavonoid from hops (Humulus lupulus). Molecules. 2015;20:754–779.10.3390/molecules20010754
- Possemiers S, Bolca S, Grootaert C, et al. The prenylflavonoid isoxanthohumol from hops (Humulus lupulus L.) is activated into the potent phytoestrogen 8-prenylnaringenin in vitro and in the human intestine. J Nutr. 2006;136:1862–1867.
- Bolca S, Possemiers S, Maervoet V, et al. Microbial and dietary factors associated with the 8-prenylnaringenin producer phenotype: a dietary intervention trial with fifty healthy post-menopausal Caucasian women. Br J Nutr. 2007;98:950–959.
- Possemiers S, Heyerick A, Robbens V, et al. Activation of proestrogens from hops (Humulus lupulus L.) by intestinal microbiota; conversion of isoxanthohumol into 8-prenylnaringenin. J Agric Food Chem. 2005;53:6281–6288.10.1021/jf0509714
- Nakamura T, Tokushima T, Kawabata K, et al. Absorption and metabolism of 4-hydroxyderricin and xanthoangelol after oral administration of Angelica keiskei (Ashitaba) extract in mice. Arch Biochem Biophys. 2012;521:71–76.10.1016/j.abb.2012.03.013
- Zhang SQ, Zhang SZ. Oral absorption, distribution, metabolism, and excretion of icaritin in rats by Q-TOF and UHPLC-MS/MS. Drug Test Anal. 2017;9:1604–1610.10.1002/dta.v9.10
- Yang W, Yu XC, Chen XY, et al. Pharmacokinetics and tissue distribution profile of icariin propylene glycol-liposome intraperitoneal injection in mice. J Pharm Pharmacol. 2012;64:190–198.10.1111/j.2042-7158.2011.01388.x
- Mukai R, Fujikura Y, Murota K, et al. Prenylation enhances quercetin uptake and reduces efflux in Caco-2 cells and enhances tissue accumulation in mice fed long-term. J Nutr. 2013;143:1558–1564.10.3945/jn.113.176818
- Konishi Y, Hitomi Y, Yoshida M, et al. Absorption and bioavailability of artepillin C in rats after oral administration. J Agric Food Chem. 2005;53:9928–9933.10.1021/jf051962y
- Pang Y, Nikolic D, Zhu D, et al. Binding of the hop (Humulus lupulus L.) chalcone xanthohumol to cytosolic proteins in Caco-2 intestinal epithelial cells. Mol Nutr Food Res. 2007;51:872–879.10.1002/(ISSN)1613-4133
- van de Schans MG, Bovee TF, Stoopen GM, et al. Prenylation and backbone structure of flavonoids and isoflavonoids from licorice and hop influence their phase I and II metabolism. J Agric Food Chem. 2015;63:10628–10640.10.1021/acs.jafc.5b04703