Abstract
Ethylene (C2H4), a phytohormone that is produced in response to both abiotic and biotic stresses, is an important factor influencing the efficiency of Agrobacterium-mediated transformation. In this study, effects of various ethylene inhibitors on the efficiency of Agrobacterium-mediated genetic transformation in drought-tolerant wild watermelon was comparatively examined. Consequently, in comparison to the application of chemical inhibitors such as AgNO3 and aminoethoxyvinylglycine (AVG), lower ethylene level was observed when the infecting Agrobacterium contained a gene for 1-aminocyclopropane-carboxylic acid (ACC) deaminase (acdS), which cleaves ethylene precursor ACC into α-ketobutyrate and ammonia. GUS histochemical and spectrophotometric enzyme assays showed that acdS was more effective in enhancing gene transfer than the chemical ethylene inhibitors. Efficiency of transgenic shoots formation was higher in acdS- and AVG-treated explants. These observations demonstrated that controlling the ethylene level during co-cultivation and shoot formation, particularly using the acdS-harboring Agrobacterium, is advantageous for enhancing the transformation efficiency in this plant.
A gene for 1-aminicyclopropane-carboxylic acid deaminase was effective for enhancing the efficiency of genetic transformation in wild watermelon.

With the threat of climate change leading to more frequent and sporadic droughts in the future, it is important to give focus on plants that show potential in tolerating severe drought stress condition. These plants adapt to the harsh water deficit environments by exhibiting excellent physiological, morphological and/or metabolic adaptations [Citation1–3], which are exerted by unique molecular mechanisms that are essentially different from the commonly known model plants like Arabidopsis, which are not by nature, tolerant to the severe drought stress. Wild watermelon (Citrullus lanatus sp), an inhabitant of the Kalahari Desert, can thrive and produce fruits in harsh environments characterized by minimal rainfall, high temperatures and strong sunlight [Citation4]. Unique responses to drought stress have been reported in this plant, such as the biosynthesis of compatible solute citrulline [Citation4], stimulation of root growth [Citation5], and dynamic proteome changes [Citation6]. These observations make this plant to be an attractive source of useful genetic traits for Agrobacterium-mediated molecular breeding [Citation7]. Examining the gene function in wild watermelon will be valuable not only for elucidating the molecular mechanisms underlying the excellent drought resistance, but also mining gene resource for conferring the stress resistance to other crop species [Citation8].
Agrobacterium transformation has become an important molecular breeding tool because of its unique ability to transfer and integrate a gene of interest into genome of a host plant [Citation9]. Its capability to transfer a particular segment of the T-DNA of the tumor-inducing (Ti) plasmid and to integrate into the host genome has then been extensively explored and manipulated [Citation10]. However, genetic transformation of the majority of plants remains technically demanding due to a number of constraints, awaiting substantial improvement of the transformation efficiency.
A phytohormone ethylene plays a major role limiting the Agrobacterium-mediated gene transfer, as the inoculation of explants with Agrobacterium induces ethylene production, which subsequently lead to the decreased T-DNA transfer via suppression of vir gene expression [Citation11]. In ethylene biosynthetic pathway, methionine is converted by S-adenosylmethionine (SAM) synthetase to generate SAM, a common intermediate metabolite not only for the ethylene biosynthesis but also for the biosynthesis of spermidine/spermine and other methylated acceptors in the cells (Figure ) [Citation12]. SAM is then metabolized to 1-aminocyclopropane-1-carboxylic acid (ACC) by ACC synthase (ACS), a committing enzyme for ethylene biosynthesis [Citation13,14]. ACC is then converted to ethylene catalyzed by ACC oxidase (ACO). Chemical inhibitors such as AgNO3, a suppressor to ethylene perception [Citation14], and aminoethoxyvinlyglycine (AVG), a specific inhibitor for ACS, have been widely used to alleviate the negative effects of ethylene and to improve transformation process in some plants including Brassicaceae [Citation15–17], bottle gourd [Citation18], and rubber tree [Citation19]. In contrast, positive effects of these chemical inhibitors were limited or marginal in the transformation of maize [Citation20] and citrus [Citation21], suggesting that the effects of these chemical ethylene inhibitors may be divergent among different plant species.
Figure 1. Schematic diagram of ethylene biosynthesis with the enzymes that synthesize or oxidase 1-aminocyclopropane-1-carboxylic acid (ACC) to produce ethylene. The chemical and biological effectors showing different points of inhibition or decomposition of the ethylene synthesis intermediate are highlighted by gray squares. Met, L-methionine; SAM, S-adenosyl-L-methionine, AVG, aminoethoxyvinylglycine; AgNO3, silver nitrate; α-KB, α-ketobutyrate.
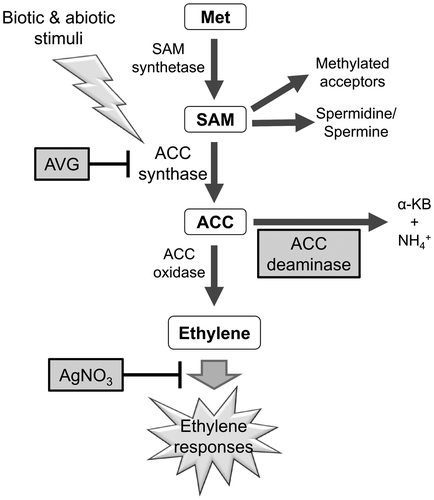
Recently, a non-chemical methodology for controlling the ethylene level has been developed using an enzyme ACC deaminase [Citation22]. ACC deaminase catalyzes decomposition of ACC into ammonia and α-ketobutyrate [Citation23], thereby reducing the formation of ethylene [Citation24]. The plant-borne ACC is exuded from plant roots, and then taken up as a carbon source by the ACC deaminase-containing microbes in the rhizosphere [Citation25]. These plant growth-promoting bacteria (PGPB) function as a sink for ACC, lowering the ACC level in the equilibrium of plant-microbe metabolic network, and are implicated in decreasing plant ethylene level [Citation26,27]. Promotion of plant growth by PGPB has been widely reported in various crop species [Citation28–31]. Agrobacteria in nature do not possess a gene for ACC deaminase (acdS) in their genomes [Citation22,27]. An engineered Agrobacterium, or super-Agrobacterium, in which acdS was incorporated on a plasmid vector, was shown to decrease plant-borne ethylene level during genetic transformation procedure, which resulted in the improvement of transformation efficiency in several plants such as melon [Citation32,33], canola [Citation34], tomato and Erianthus [Citation35]. The effectiveness of the acdS-borne Agrobacterium in diverse plant species awaits further investigation, together with the comparative evaluation with the chemical ethylene inhibitors to improve genetic transformation.
Therefore, examining the effects of different ethylene inhibitors for genetic transformation of wild watermelon should be useful for the optimization of Agrobacterium-mediated gene transfer research in this plant. In this study, we report the efficiency of different ethylene inhibitors during Agrobacterium-mediated gene transfer in wild watermelon.
Materials and methods
Chemicals
All reagents were purchased from Wako Chemicals, Osaka, Japan, otherwise described elsewhere.
Bacterial strains and culture conditions
An Agrobacterium strain EHA105 [Citation36] was used in this study. The Agrobacterium EHA105 was transformed with a binary vector pIG121-Hm, which contains uidA (GUS) gene with an intron sequence from a plant [Citation37]. The pBBRacds plasmid that carries the ACC deaminase gene was described previously [Citation11]. The pBBRcont plasmid was constructed by removing the ACC deaminase gene from the pBBRacds, via digestion of the pBBRacds by XbaI and HindIII restriction enzymes, blunting of the resultant nucleotide overhangs by Klenow fragment of E. coli DNA polymerase I (New England Biolabs, Ipswich, MA, USA) in the presence of 2.5 mM dNTPs, then self-ligation by a Ligation High Kit (Toyobo, Osaka, Japan). The pBBRacds or pBBRcont plasmids were introduced into the pIG-121-Hm-containing Agrobacterium strain EHA105 by electroporation (Biorad micropulser, Biorad, Hercules, CA, USA) according to the manufacturer’s instruction. The transformed cells were selected and cultured in LB medium supplemented with 50 mg L−1 rifampicin and 50 mg L−1 hygromycin for the Agrobacterium containing pIG121-Hm, and 50 mg L−1 rifampicin, 50 mg L−1 hygromycin, and 50 mg L−1 gentamicin for the Agrobacterium containing both pIG121-Hm and pBBR vector derivatives. Agrobacterium suspension cultures at a density of OD600 = 0.5~0.7 were used for the transformation experiments.
Plant materials and genetic transformation
Aceptic seedlings of wild watermelon, Citrullus lanatus sp. No. 101117-1 were prepared as described previously [Citation38] with following minor modifications. A scalpel and forceps were carefully used to de-coat and peel the seeds with minimum damage to the cotyledons. The de-coated seeds were sterilized in a solution containing 5% sodium hypochlorite and 0.005% Tween20 for 10 min and then rinsed five times with sterile water. The sterilized seeds were soaked in sterile water and kept in the dark overnight at 30 °C. The next day the seeds were placed on basal medium (BM) containing Murashige and Skoog [Citation39] salts, 10 mg L−1 thiamine, 100 mg L−1 myo-inositol, 30 g L−1 sucrose and 0.8% agar in the dark at 30 °C for 4 days for germination.
The expanded cotyledons were sectioned into 5 mm square explants using a sterile blade. Infection was done immediately by soaking the explants in the Agrobacterium suspension solution for 10 min and then blotted on sterile filter papers to remove excess liquid. The inoculated explants were co-cultivated for 7 days on BM agar medium supplemented with 4 mg L−1 6-benzyladenine and 10 mg L−1 acetosyringone, and grown at 28 °C under a 16 h photoperiod. In the ethylene inhibitor experiment, BM medium was supplemented with either 1 μM aminoethoxyvinlylglycine (AVG) or 40 μM silver nitrate. For shoot formation, the explants after co-cultivating for 7 days were rinsed 5 times with sterile water, 3 times with 100 mg L−1 meropenem trihydrate, and then cultured for 2 weeks on shoot-inducing media containing BM supplemented with 10 g L−1 polyvinylpyrrolidone (PVP) (Nacalai tesque, Kyoto, Japan), 1 mg L−1 4-3-indolyl butyric acid, 10 mg L−1 acetosyringone, 100 mg L−1 thidiazuron (TDZ) (Nacalai tesque, Kyoto, Japan), 100 mg L−1 meropenem trihydrate and 50 mg L−1 hygromycin. The explants were then maintained at 28 °C under a 16 h photoperiod. Infection experiments were performed using 30 explants per treatment, and the infection experiments for a given treatment was repeated 3 times.
Ethylene gas quantification
To quantify ethylene gas evolved from watermelon tissues, the Agrobacterium-inoculated explants with different ethylene inhibitors were cultivated in the BM medium in a 15 mL glass vial with a rubber cap sealed with Para-film (Bemis laboratories, Clara, Ireland), which allows needles through, and remains intact to eliminate chances of gas leakage. The explants were co-cultivated with Agrobacteria for 7 days with 16 h light per day. To measure the evolved ethylene, a 1 mL syringe was used to withdraw gas from the vial at 3, 5 and 7 days after inoculation (DAI), and directly injected onto the gas chromatography.
The amount of ethylene was measured by gas chromatography essentially as described previously [Citation40], with the following modification. A gas chromatography apparatus GC 4000 (GL Science, Tokyo, Japan) coupled with a flame-ionization detector (FID) equipped with a DB-5 column (30 m × 0.32 mm, 0.25 μm, Agilent Technologies, Santa Clara, MA, USA) was used to quantify the ethylene gas. The oven temperature was 60 °C, and the flow rate of the helium carrier gas was 30 mL min−1. Under these conditions, the retention time for ethylene was 1.9 min. Quantification of ethylene was performed with a standard curve generated from a serial dilution of the commercial ethylene gas standard (GL Science, Tokyo, Japan).
Histochemical β-glucuronidase (GUS) assay
Histochemical GUS assay was performed as described previously [Citation41] with following minor modifications. Explants were incubated overnight at 37 °C in a GUS assay solution containing 100 mM sodium phosphate buffer, pH 7.0, 10 mM EDTA, 0.1% Triton, 0.5 mM potassium ferrocyanide, 0.5 mM potassium ferricyanide and 0.5 mg mL−1 5-bromo-4-chloro-3-indoyl glucuronide (X-gluc). The explants were then soaked in 95% ethanol for several hours to remove chlorophyll and other intrinsic pigments. Quantification of the GUS-stained area on the leaf disks was performed using ImageJ [Citation42].
Enzyme assay of GUS activity
GUS enzymatic activity was measured essentially as described previously [Citation43], with following minor modifications. Approximately 1 g of the infected explants was homogenized in an extraction buffer (50 mM sodium phosphate buffer, pH 7.0, 10 mM β-mercaptoethanol, 10 mM EDTA) with a pinch of sea sand in a pre-chilled pestle and mortar on ice. The homogenate was centrifuged at 20,000 × g for 15 min at 4 °C, then the supernatant was collected into a new 1.5 mL tube. A small sample of the extract (100 μL) was assayed in 900 μL assay buffer (extraction buffer with 1 mM of p-nitrophenyl β-D-glucopyranoside) and incubated at 37 °C for 2 h. The reaction was terminated by adding 400 μL of 2.5 M 2-amino-2-methylpropandiol. The absorbance was measured at 415 nm against a blank without the extract. Blanks without substrate were also measured and the values subtracted from the assayed samples to eliminate the chlorophyll color effect.
Molecular analysis
Genomic DNA was extracted from the shoots using DNA Plant Mini Kit (QIAGEN, Valencia, CA, USA) following the manufacturer’s protocol. The endogenous actin sequence was amplified at an annealing temperature of 55 °C with a pair of primers (Act-F: 5′CATTCTCCGTTTGGACCTTGCT-3′ and Act-R: 5′TCGTAGTTTTCTCAATGGAGGAACTG-3′) to check the presence of genome DNA. The GUS gene was amplified at an annealing temperature of 52 °C with a pair of primers (GUS-F: 5′-CAACGAACTGAACTGGCAGA-3′, and GUS-R: 5′GGCACAGCACATCAAAGAGA-3′) which were designed to amplify 989 bp fragment of the GUS gene. The nptII gene was amplified at an annealing temperature of 53 °C with a pair of primers (km-F: 5′-GGCTATTCGGCTATGACTGG-3′, and km-R: 5′-AGCCAACGCTATGTCCTGAT-3′) which were designed to amplify 720 bp fragment of the nptII gene. A region of DNA fragment in the pBI121-Hm backbone, which corresponds to the outside of the T-DNA borders was amplified at an annealing temperature of 53 °C with a pair of primers (Bor-F: 5′–CCTGGCAAAGCTCGTAGAAC-3’, and Bor-R: 5′–GTATTCGTGCAGGGCAAGAT-3′) which were designed to amplify 862 bp fragment. The KAPATaq enzyme (KAPA Biosystems, Wilmington, MA, USA) was used for the amplification according to the manufacturer’s instruction. The PCR conditions were as follows: pre-denature at 95 °C for 3 min, 35 cycles of denaturing at 95 °C for 30 s, annealing at the indicated temperature as above for 30 s and extension at 72 °C for 1 min, and a final extension at 72 °C for 5 min. PCR products were run on 0.8% agarose gels and stained with GelGreen Nucleic Acid Gel Stain (Biotium, Hayward, CA, USA), and viewed under blue light using LuminoGraph I gel viewer (ATTO, Tokyo, Japan).
Results and discussion
Watermelon explants were inoculated with Agrobacterium EHA105 containing a binary vector pIG121-Hm, and effect of different ethylene inhibitors on the ethylene evolution from the inoculated explants was compared. At 3 days after inoculation (DAI), ethylene concentration in the culture vial containing Agrobacterium-inoculated explants with no inhibitor was significantly higher than the non-inoculated control explants (Figire ). The ethylene accumulation was reduced to the non-inoculated control level not only by the supplementation of AVG, an inhibitor of ethylene biosynthesis, but also by AgNO3, an inhibitor of ethylene action. Mechanisms underlying the latter inhibition is currently unclear, but it is possible that AgNO3 might suppress positive feedback regulation of ethylene biosynthesis. A previous study reported that another ethylene action inhibitor 2,5-norbornadiene suppressed the gene expression of ACO enzyme in pea [Citation44]. Both positive and negative feedback controls of ethylene biosynthesis have been reported in various plants, in which ACS and ACO gene family members are differentially regulated in a temporal and tissue-specific manner [Citation12,45]. Further research will be needed to examine the possible relationships between the AgNO3 application and ethylene biosynthesis in wild watermelon transformation.
Figure 2. Effects of different ethylene inhibitors on the ethylene evolution from cotyledon explants inoculated with an Agrobacterium strain EHA105 harboring a binary vector pIG121-Hm. Accumulation of ethylene gas in the headspace of culture vials after varying days after inoculation (DAI) was measured using a gas chromatography. Non-inoculated, explants were not inoculated with Agrobacterium; No inhibitor, no ethylene inhibitors was used for transformation, AgNO3, silver nitrate was included in the media; AVG, aminoethoxyvinlyglycine was included in the media; pBBRcont, pBBR control vector was co-integrated in the Agrobacterium; pBBRacds, pBBRacds vector that contained ACC deaminase gene was co-integrated in the Agrobacterium. Data are the averages and SD of three vials for each treatment, in which three explants were incubated in each vial. Letters on top of the bars indicate statistical significance at the 95% confidence level based on Duncan’s mean comparison.
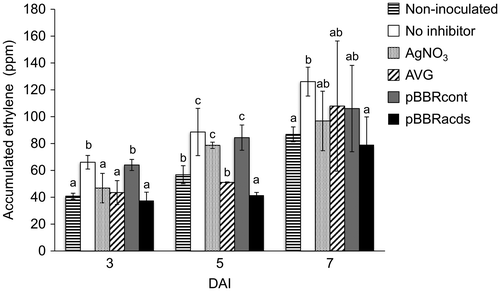
Notably, co-transformation with ACC deaminase gene-containing pBBRacds effectively reduced ethylene accumulation to the non-inoculated control level. Co-transformation of pBBRcont, a vector control in which ACC deaminase gene was eliminated from the pBBRacds, resulted in the similar level of ethylene accumulation as no ethylene inhibitor control, suggesting that the ethylene-inhibiting effect by pBBRacds is attributable to the presence of ACC deaminase gene.
At 5 and 7 DAIs, ethylene levels showed a varying gradual increase for each treatment. At 5 DAI, significant differences were observed among different inhibitors, with pBBRacds recording the lowest ethylene accumulation, followed by AVG treatment. The no inhibitor sample recorded the highest quantity of accumulated ethylene, which was not significantly different from those of AgNO3 and pBBRcont samples. At 7 DAI, ethylene levels in the AgNO3 and AVG treatments were high and statistically not different from the no-inhibitor control. The ethylene level was significantly lower in the pBBRacds treatment, which remained at the non-inoculated level. These observations suggested that pBBRacds plasmid might have a higher potential in lowering the ethylene synthesis in wild watermelon explants under Agrobacterium-mediated gene transfer, as compared to the chemical inhibitors during the early stages of co-cultivation.
To evaluate more on the different ethylene inhibitors on the Agrobacterium-mediated gene transfer into watermelon explants, the uidA reporter gene was used in histochemical GUS assay (Figure ). The GUS-expressing leaf area was quantified by the ImageJ-based analysis and expressed as the percentage of stained area over the total area of the explants (Figure ). The explants with no inhibitor showed the lowest percentages of stained areas as compared to those supplemented with ethylene inhibitors AVG and AgNO3. The effect of AVG in the present study in wild watermelon was consistent with previous observations in melon [Citation46], where this ACC synthase inhibitor significantly enhanced transgene expression in Agrobacterium-mediated transformation.
Figure 3. Histochemical GUS staining of watermelon cotyledon explants inoculated with an Agrobacterium strain EHA105 harboring pIG121-Hm binary vector. The horizontal axis represents different ethylene inhibitors as in the legend of Figure 2. Representative images of explants after varying DAI were shown.
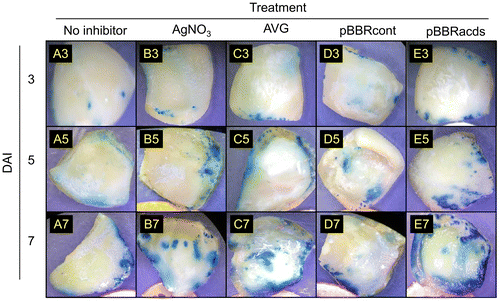
Figure 4. Quantification of leaf surface areas that showed GUS transgene expression on the cotyledonary explants of wild watermelon in the presence of different ethylene inhibitors. Values at varying DAI are shown. The ImageJ software was used to quantify the areas of blue stains on the explants. Nomenclature of the different ethylene inhibitors is the same as in Figure 2. Data are the average ± SD of 30 explants. Letters on top of the bars indicate statistical significance at the 95% confidence level based on Duncan’s mean comparison.
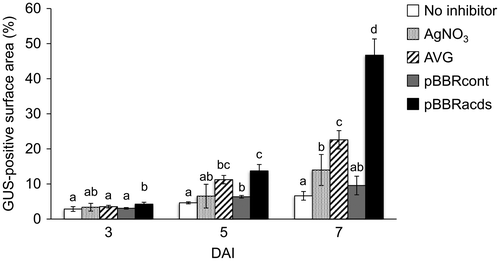
Notably, explants inoculated with pBBRacds-containing Agrobacterium achieved largest GUS-positive surface areas in the GUS histochemical assay, as the stained area occupied 45.48 ± 3.96% of the total surface area of the explant at 7 DAI (Figures and ). The stained areas on explants inoculated with pBBRcont-containing Agrobacterium were almost equivalent to that of no inhibitor control, suggesting that control plasmid used as a backbone for making pBBRacds had little or no effect in enhancing the gene transfer. The AgNO3 and AVG treatments also showed higher stained areas (13.75 ± 1.46 and 21.53 ± 0.93%, respectively) in comparison to the no-inhibitor control (6.36 ± 0.23%), but the effect of these chemical inhibitors was relatively modest. These observations also suggested that, although the chemical ethylene inhibitors enhanced the rate of gene transfer, which may not be as much as compared to the non-chemical approach using ACC deaminase. The results also demonstrated that the ACC-consumption approach by ACC deaminase was significantly effective in aiding the Agrobacterium-mediated transformation processes. Although the chemical ethylene inhibitors have been successfully used for improving tissue culture and genetic transformation in many plants [Citation15–19,47], side-effects of chemical ethylene inhibitors on plant development have been also reported, e.g. modification of sex determination in the cucumber flowers by AgNO3 [Citation48] and reduction of fruit size and weight in Cucumis species by AVG [Citation49]. The non-chemical approach for controlling ethylene using ACC deaminase may have an advantage to circumvent the potential side-effects of chemical inhibitors. Further research will be needed to evaluate this possible advantage, by monitoring the vegetative and reproductive growth characteristics of T0 plants raised by the chemical and non-chemical approaches in a comparative manner.
The results on the change of the accumulated ethylene gas during co-cultivation (Figure ) and the efficiency of gene transfer judging from the GUS-positive surface area (Figure ) could suggest important stage in the co-cultivation period. The reported co-cultivation period for Agrobacterium-mediated genetic transformation of wild watermelon was 3 days [Citation38], thus it is important to minimize the evolved ethylene from the explants during this period to improve the efficiency of gene transfer from Agrobacterium to the explants cells. The lower ethylene concentration between 3 and 5 DAI in explants inoculated with pBBRacds-harboring Agrobacterium (Figure ) could have greatly contributed to the increase in gene transfer event.
To further evaluate the effects of various ethylene effectors on the gene transfer event, GUS enzymatic activity was measured by a spectrophotometric assay. At 7 DAI, the GUS activity was highest in explants inoculated with pBBRacds-containing Agrobacterium (8.68 ± 0.27 nmol min−1 gFW−1) (Figure ), which was more than double the activity in explants without ethylene inhibitor (2.72 ± 0.25 nmol min−1 gFW−1), and even higher than those co-cultivated in medium supplemented with chemical inhibitors AgNO3 and AVG (4.54 ± 0.43 and 5.31 ± 0.43 nmol min−1 gFW−1, respectively). The results confirmed that pBBRacds-containing Agrobacterium was most effective in improving the efficiency of Agrobacterium-mediated gene transfer.
Figure 5. Spectrophotometric GUS enzyme assay using the total protein extracts from watermelon explants inoculated with Agrobacterium containing pIG121-Hm that were treated with different ethylene inhibitors. A total of 10 explants per treatment were harvested at 7 DAI, then protein extracts were independently prepared from individual explants and used for the enzyme assay. Nomenclature of the different ethylene inhibitors is the same as Figure 2. The 4-nitrophenyl β-D-glucuronide was used as a substrate. Enzyme activities were expressed as nmol of nitrophenyl produced per min per gram fresh weight of the explants. Data are average ± SD of ten explants. Letters on top of the bars indicate statistical significance at the 95% confidence level based on Duncan’s mean comparison.
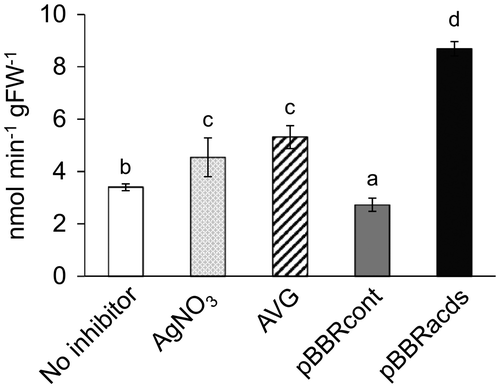
The explants were further grown in the shoot-inducing media, and the number of explants that generated shoots were counted and compared among the treatments (Table ). Consequently, the percentage of explants with shoots was not significantly different at 95% confidence level among the treatments. However, the number of shoots per explant had a significant difference, with no inhibitor and pBBRcont treatments recorded the lower number of shoots, whereas AVG and pBBRacds treatments recorded the higher number of shoots per explant. The GUS histochemical staining of these shoots showed that the transformation efficiency as calculated from the percentage of GUS-positive shoots among total shoots was higher than 69% in the three-ethylene inhibition treatments (AgNO3, AVG and pBBRacds). These observations suggested a lower frequency of escapes in the ethylene inhibition treatments in comparison to the control, and this is consistent with previous reports in which lower number of escapes was observed with ethylene inhibitor in bottle gourds [Citation18] and with acdS-harboring Agrobacterium in melon [Citation33]. The present study suggested that ethylene inhibitors not only increased the efficiency of gene transfer but also improved the regeneration of shoots from explants in wild watermelon.
Table 1. Effects of different ethylene inhibitors on transgenic shoot formation in wild watermelon.
PCR amplification of the introduced DNA from randomly selected transgenic shoots exhibited the bands with the expected size of 989 bp for the uidA (GUS) gene, and of 720 bp for the nptII (kanamycin resistant) gene for most of the selected shoots in all treatments (Figure (A) and (B)). One sample in the “no inhibitor” treatment did not give the expected bands (lane 4, Figure (A) and (B)), suggesting that this shoot might represent an escape plant, as it has been documented that selection under kanamycin usually gives a considerable number of escapes during Agrobacterium transformation [Citation50,51]. All other treatments, i.e. AgNO3, AVG and pBBRacds exhibited bands for the uidA and nptII genes, suggesting that the transgenes were present in the selected shoots.
Figure 6. PCR amplification of the transgene fragments from transformed shoots of watermelon under different ethylene inhibitors. Genome DNAs were extracted from the randomly selected shoots and used as templates for amplifying fragments of (A) uidA 989 bp, (B) the nptII 720 bp transgenes, (C) a 862 bp region of pIG121-Hm backbone outside of T-DNA borders, and (D) endogenous wild watermelon actin as reference gene to check the intactness of the DNA. The amplified fragments (A, B, C) are segments in the pIG121-Hm reporter plasmid used in the experiment for monitoring the gene transfer. Lane M, DNA size marker; 1, pIG121-Hm plasmid DNA as positive control template; 2, non-transformed wild watermelon leaf disk; 3–12, genomic DNAs extracted from regenerated shoots treated with different inhibitors (3–4, no effector; 5–6, AgNO3; 7–8, AVG; 9–10, pBBRcont; 11–12, pBBRacds).
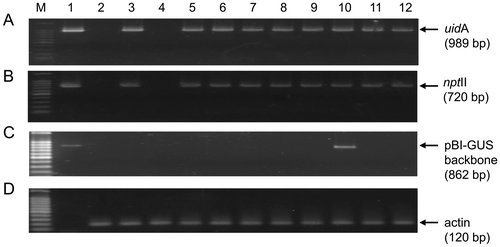
The process of Agrobacterium-mediated genetic transformation involves the transfer and integration of the T-DNA regions to the plant genome [Citation52], thus the regions of the binary vector backbone are not transferred into the plant cells. Although Agrobacterium cells are removed after co-cultivation by bacterial antibiotics and a series of washing, residual Agrobacterium cells may exist on the surface of regenerated shoots, and may give rise to the PCR bands for uidA and nptII genes. To test this possibility, a segment of pBI121-Hm vector backbone outside of the T-DNA region was amplified from the genomic DNA from the regenerated shoots by PCR (Figure (C)). Consequently, a band of expected size of 826-bp was detected from one of the regenerated shoots (Lane 10, Figure (C)), suggesting that the residual Agrobacterium cells might exist in this shoot. The 826-bp bands were not detected from all the other shoots, suggesting that these shoots might be devoid of contaminating Agrobacterium cells. A band for endogenous actin gene was detected from all the shoots, demonstrating that genome DNAs were properly prepared for the PCR experiments. Taken together, these observations suggested that the transgenes have been successfully transferred and maintained in the regenerated wild watermelon shoots.
Conclusion
In this study, effects of two chemical inhibitors for ethylene action (AgNO3), ethylene biosynthesis (AVG), and a methodology for eliminating ethylene precursor ACC using acdS-harboring Agrobacterium, on the Agrobacterium-mediated transformation of drought-tolerant wild watermelon were comparatively analyzed. Consequently, acdS-harboring Agrobacterium was most effective compared to the other commonly used chemical inhibitors, in the suppression of ethylene accumulation during the co-cultivation period, transient expression of GUS transgene in the infected explants, and generation of transgenic shoot formation in wild watermelon. The present results, therefore, showed the potential of the non-chemical approach using ACC deaminase in controlling ethylene from explants and improving the efficiency of Agrobacterium-mediated transformation.
Author contribution statement
GM, KA, SN and HS conceived and designed the study. GM and KA performed most of the experiments with the help from SN, HS, HE, HT. GM and KA wrote the manuscript while SN, HS, HE, HT read and edited the final manuscript.
Disclosure statement
No potential conflict of interest was reported by the authors
Funding
This work was supported by Grant-in-Aid for Scientific Research [grant number 17K07755].
Acknowledgement
We thank Atsushi Kato and Mako Matsuo for helping with seed preparation and various experimental procedures of wild watermelon. The authors gratefully acknowledge fundings for this co-research project from the Grant-in-Aid for Scientific Research (17K07755) from JSPS, Cooperative Research Grant of the Gene Research Center, University of Tsukuba, and from the Joint Research Program and the Project Marginal Region Agriculture, the Arid Land Research Center, Tottori University, and the IPDRE Program, Tottori University.
References
- Bray EA. Plant responses to water deficit. Trends Plant Sci. 1997;2:48–54.10.1016/S1360-1385(97)82562-9
- Bartels D, Salamini F. Desiccation tolerance in the resurrection plant craterostigma plantagineum. a contribution to the study of drought tolerance at the molecular level. Plant Physiol. 2001;127:1346–1353.10.1104/pp.010765
- Osakabe Y, Osakabe K, Shinozaki K, et al. Response of plants to water stress. Front Plant Sci. 2014;5:86.
- Kawasaki S, Miyake C, Kohchi T, et al. Responses of wild watermelon to drought stress: accumulation of an ArgE homologue and citrulline in leaves during water deficits. Plant Cell Physiol. 2000;41:864–873.10.1093/pcp/pcd005
- Yoshimura K, Masuda A, Kuwano M, et al. Programmed proteome response for drought avoidance/tolerance in the root of a C3 xerophyte (wild watermelon) under water deficits. Plant Cell Physiol. 2008;49:226–241.
- Akashi K, Yoshida K, Kuwano M, et al. Dynamic changes in the leaf proteome of a C3 xerophyte, Citrullus lanatus (wild watermelon), in response to water deficit. Planta. 2011;233:947–960.10.1007/s00425-010-1341-4
- Akashi K, Yoshimura K, Nanasato Y, et al. Wild plant resources for studying molecular mechanisms of drought/strong light stress tolerance. Plant Biotechnol. 2008;25:257–263.10.5511/plantbiotechnology.25.257
- Akashi K, Yoshimura K, Kajikawa M, et al. Potential involvement of drought-induced Ran GTPase CLRan1 in root growth enhancement in a xerophyte wild watermelon. Biosci Biotechnol Biochem. 2016;80:1907–1916.10.1080/09168451.2016.1191328
- Kakkar A, Verma VK. Agrobacterium mediated biotransformation. J Appl Pharm Sci. 2011;1:29–35.
- Komari T, Takakura Y, Ueki J, et al. Bionary vector and super-binary vectors. Methods Mol Biol. 2006;343:15–41.
- Nonaka S, Yuhashi K-I, Takada K, et al. Ethylene production in plants during transformation suppresses vir gene expression in Agrobacterium tumefaciens. New Phytol. 2008;178:647–656.10.1111/nph.2008.178.issue-3
- Wang KLC, Li H, Ecker JR. Ethylene biosynthesis and signaling networks. Plant Cell. 2002;S131–151.10.1105/tpc.001768
- Beyer EM. A potent inhibitor of ethylene action in plants. Plant Physiol. 1976;58:268–271.10.1104/pp.58.3.268
- Van de Poel B, Van der Straeten D. 1-aminocyclopropane-1-carboxylic acid (ACC) in plants: more than just the precursor of ethylene! Front Plant Sci. 2014;5:640.
- De Block M, De Brouwer D, Tenning P. Transformation of Brassica napus and Brassica oleracea using agrobacterium tumefaciens and the expression of the bar and neo genes in the transgenic plants. Plant Physiol. 1989;91:694–701.10.1104/pp.91.2.694
- Wahlroos T, Susi P, Tylkina L, et al. Agrobacterium-mediated transformation and stable expression of the green fluorescent protein in Brassica rapa. Plant Physiol Biochem. 2003;41:773–778.10.1016/S0981-9428(03)00119-0
- Takasaki T, Hatakeyama K, Hinata K. Effect of silver nitrate on shoot regeneration and agrobacterium-mediated transformation of turnip (Brassica rapa L. var. rapifera). Plant Biotechnol. 2004;21:225–228.10.5511/plantbiotechnology.21.225
- Han J-S, Kim CK, Park SH, et al. Agrobacterium-mediated transformation of bottle gourd (Lagenaria siceraria Standl.). Plant Cell Rep. 2005;23:692–698.10.1007/s00299-004-0874-z
- Kala RG, Abraham V, Sobha S, et al. Agrobacterium-mediated genetic transformation and somatic embryogenesis from leaf callus of Hevea brasiliensis: effect of silver nitrate. In: Sabu A, Augustine A, editors. Prospects in bioscience: addressing the issues. New Delhi: Springer India; 2012. p. 303–315.10.1007/978-81-322-0810-5
- Ishida Y, Saito H, Hiei Y, et al. Improved protocol for transformation of maize (Zea mays L.) Mediated by Agrobacterium tumefaciens. Plant Biotechnol. 2003;20:57–66.10.5511/plantbiotechnology.20.57
- Marutani-Hert M, Bowman KD, McCollum GT, et al. A dark incubation period is important for agrobacterium-mediated transformation of mature internode explants of sweet orange, grapefruit, citron, and a citrange rootstock. PLoS One. 2012;7:e47426.10.1371/journal.pone.0047426
- Nonaka S, Ezura H. Plant-Agrobacterium interaction mediated by ethylene and super-Agrobacterium conferring efficient gene transfer. Front Plant Sci. 2014;5:1–7.
- Honma M, Shimomura T. Metabolism of 1-aminocyclopropane-1-carboxylic acid. Agric Biol Chem. 1978;42:1825–1831.
- McDonnell L, Plett JM, Andersson-Gunneras S, et al. Ethylene levels are regulated by a plant encoded 1-aminocyclopropane-1-carboxylic acid deaminase. Physiol Plant. 2009;136:94–109.10.1111/ppl.2009.136.issue-1
- Glick BR. Modulation of plant ethylene levels by the bacterial enzyme ACC deaminase. FEMS Microbiol Lett. 2005;251:1–7.10.1016/j.femsle.2005.07.030
- Glick BR. Bacteria with ACC deaminase can promote plant growth and help to feed the world. Microbiol Res. 2014;169:30–39.10.1016/j.micres.2013.09.009
- Singh RP, Shelke GM, Kumar A, et al. Biochemistry and genetics of ACC deaminase: a weapon to “stress ethylene” produced in plants. Front Microbiol. 6;2015;937.
- Shaharoona B, Arshad M, Zahir ZA. Effect of plant growth promoting rhizobacteria-containing ACC-deaminase on maize (Zea mays L.) growth under axenic conditions and on nodulation in mung bean (Vigna radiate L.). Lett Appl Microbiol. 2004;42:155–159.
- Zahir ZA, Munir A, Asghar HN, et al. Effectiveness of rhizobacteria containing ACC deaminase for growth promotion of peas (Pisum sativum) under drought conditions. J Microbiol Biotechnol. 2008;18:958–963.
- Saleem M, Arshad M, Hussain S, et al. Perspective of plant growth promoting rhizobacterium (PGPR) containing ACC deaminase is stress agriculture. J Ind Microbiol Biotechnol. 2007;34:635–648.10.1007/s10295-007-0240-6
- Chen L, Dodd IC, Theobald JC, et al. The Rhizobacterium Variovorax paradoxus 5C-2, containing ACC deaminase, promotes growth and development of Arabidopsis thaliana via ethylene-dependent pathway. J Exp Bot. 2013;64:1565–1573.10.1093/jxb/ert031
- Nonaka S, Sugawara M, Minamisawa K, et al. 1-aminocyclopropane-1-carboxylate deaminase enhances agrobacterium tumefaciens-mediated gene transfer into plant cells. Appl Environ Microbiol. 2008;74:2526–2528.10.1128/AEM.02253-07
- Ntui VO, khan RS, Chin DP, et al. An efficient Agrobacterium tumefaciens-mediated genetic transformation of “Egusi” melon (Colocynthis citrullus L.). Plant Cell Tiss Organ Cult. 2010;103:15–22.10.1007/s11240-010-9748-y
- Hao Y, Charles TC, Glick BR. ACC deaminase increases the Agrobacterium tumefaciens-mediated transformation frequency of commercial canola cultivars. FEMS Microbiol Lett. 2010;307:185–190.10.1111/fml.2010.307.issue-2
- Someya T, Nonaka S, Nakamura K, et al. Increased 1-aminocyclopropane-1-carboxylate deaminase activity enhances Agrobacterium tumefaciens-mediated gene delivery into plant cells. Microbiology Open. 2013;2:873–880.
- Hood EE, Gelvin SB, Melchers LS, et al. New Agrobacterium helper plasmids for gene transfer to plants. Transgenic Res. 1993;2:208–218.10.1007/BF01977351
- Akama K, Shiraishi H, Ohta S, et al. Efficient transformation of Arabidopsis thaliana: comparison of the efficiencies with various organs, plant ecotypes and Agrobacterium strains. Plant Cell Rep. 1992;12:7–11.
- Akashi K, Morikawa K, Yokota A. Agrobacterium-mediated transformation system for the drought and excess light stress-tolerant wild watermelon (Citrullus lanatus). Plant Biotechnol. 2005;22:13–18.10.5511/plantbiotechnology.22.13
- Murashige T, Skoog F. A revised medium for rapid growth and bio assays with tobacco tissue cultures. Physiol Plant. 1962;15:473–497.10.1111/ppl.1962.15.issue-3
- Bassi PK, Spencer MS. Comparative evaluation of photoionization and flame ionization detectors for ethylene analysis. Plant Cell Environ. 1985;8:161–165.10.1111/pce.1985.8.issue-2
- Jefferson RA. Assaying chimeric genes in plants: the GUS gene fusion system. Plant Mol Biol Rep. 1987;5:387–405.10.1007/BF02667740
- Schneider CA, Rasband WS, Eliceiri KW. NIH Image to ImageJ: 25 years of image analysis. Nat Methods. 2012;9:671–675.10.1038/nmeth.2089
- Falciatore A, Formiggini F, Bowler C. Reporter genes and in vivo imaging. In: Gilmartin PM, Bowler C, editors. Molecular plant biology. New York: Oxford University Press; 2002, Vol. 2. p. 265–282.
- Petruzzelli L, Coraggio I, Leubner-Metzger G. Ethylene promotes ethylene biosynthesis during pea seed germination by positive feedback regulation of 1-aminocyclo-propane-1-carboxylic acid oxidase. Planta. 2000;211:144–149.10.1007/s004250000274
- Nakatsuka A, Murachi S, Okunishi H, et al. Differential expression and internal feedback regulation of 1-aminocyclopropane-1-carboxylate synthase, 1-aminocyclopropane-1-carboxylate oxidase, and ethylene receptor genes in tomato fruit during development and ripening. Plant Physiol. 1998;118:1295–1305.10.1104/pp.118.4.1295
- Ezura H, Yuhashi KI, Yasuta T, et al. Effect of ethylene on Agrobacterium tumefaciens-mediated transfer to melon. Plant Breeding. 2000;119:75–79.10.1046/j.1439-0523.2000.00438.x
- Kumar V, Parvatam G, Ravishankar GA. AgNO3 – a potential regulator of ethylene activity and plant growth modulator. Electron J Biotechnol. 2009;12:1–15.
- Yamasaki S, Manabe K. Application of silver nitrate induces functional bisexual flowers in gynoecious cucumber plants (Cucumis sativus L.). 2011;80:66–75.
- Custers JBM, Den Nijs APM. Effects of aminoethoxyvinylglycine (AVG), environment, and genotype in overcoming hybridization barriers between Cucumis species. Euphytica. 1986;35:639–647.10.1007/BF00021874
- Kuvshinov V, Koivu K, Kanerva A, et al. Agrobacterium tumefaciens-mediated transformation of green house-grown Brassica rapa ssp. oleifera. Plant Cell Rep. 1999;18:773–777.10.1007/s002990050659
- Yevtushenko DP, Misra S. Efficient Agrobacterium-mediated transformation of commercial hybrid poplar Populus nigra L. x P. maximowiczii A. Henry. Plant Cell Rep. 2010;29:211–221.10.1007/s00299-009-0806-z
- Tzfira T, Citovsky V. Agrobacterium-mediated genetic transformation of plants: biology and biotechnology. Curr Opin Biotechnol. 2006;17:147–154.10.1016/j.copbio.2006.01.009