ABSTRACT
Plant hormones are small molecules that play versatile roles in regulating plant growth, development, and responses to the environment. Classic methodologies, including genetics, analytic chemistry, biochemistry, and molecular biology, have contributed to the progress in plant hormone studies. In addition, chemical regulators of plant hormone functions have been important in such studies. Today, synthetic chemicals, including plant growth regulators, are used to study and manipulate biological systems, collectively referred to as chemical biology. Here, we summarize the available chemical regulators and their contributions to plant hormone studies. We also pose questions that remain to be addressed in plant hormone studies and that might be solved with the help of chemical regulators.
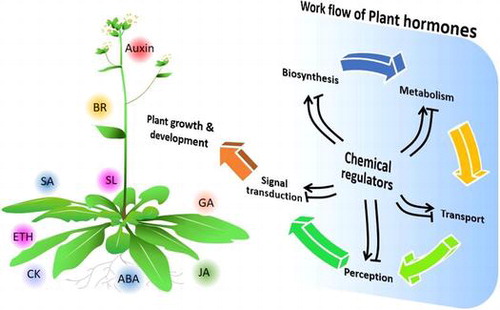
Plant hormones play versatile roles in regulating plant growth and development. Chemical regulators that perturb plant hormone biosynthesis, metabolism, transport and signal transduction are expected to contribute to deciphering the complexity of the plant hormone network and to the development of specific PGRs.
As sessile organisms, plants must sense and quickly respond to changes in the environment. Plant hormones, a series of plant endogenous small molecules, play versatile roles in regulating plant growth and development in response to ambient physical conditions. To date, auxin, gibberellin, cytokinin, abscisic acid, ethylene, brassinosteroids, jasmonic acid, salicylic acid, and strigolactones have been confirmed to function as plant hormones. The introduction of molecular biological techniques has facilitated the deciphering of plant hormone biosynthesis, metabolism, transport, and signal transduction. Recent next-generation sequencing and CRISPR-Cas9 technologies have further promoted plant hormone studies by contributing genome information of plants other than model plants (e.g. Arabidopsis and rice), as well as by allowing delicate plant genome modification. Despite these achievements, plant hormone studies continue to encounter several limitations. For example, gene redundancy often hampers studies on gene function. Although CRISPR-Cas9 can be used to delete all redundant genes, the transformed plants can be lethal or have serious developmental defects. In addition, gene-editing technologies such as CRISPR-Cas9 are still restricted to a limited number of plants.
Chemical biology, a recently emerged cross-discipline, integrates molecular biological and organic chemical methodologies to study physiological mechanisms at the molecular level. Small organic molecules with bioactivities can be applied to plants to study gene function while avoiding gene redundancy. For example, the characterization of the abscisic acid receptor is attributable to its agonist, pyrabactin, which acts selectively on a few receptors among 14 homologs [Citation1]. In addition to signal transduction regulators, inhibitors of plant hormone biosynthesis, metabolism, and transport are also useful for studying the complex signals of plant hormones. In this review, we summarize and update the progress in plant hormone studies with a focus on chemical regulators.
Auxin
In 1880, the existence of a mediator that regulates the bending of seedlings toward a light source was described by Charles Darwin and his son Francis. Salkowski identified indole-3-acetic acid (IAA, 1; (A)), a natural auxin, in fermentation media in 1885. Nearly 50 years later, IAA was isolated from plant tissues and has since become accepted as the first plant hormone. Since the 1990s, the application of molecular biology approaches in plant studies, together with genetics and biochemistry, has led to great progress in our understanding of auxin biosynthesis, metabolism, transport, and signal transduction.
Figure 1. Chemical structures of auxin-related chemicals and simplified schematic model of auxin biosynthesis, transport, and signal transduction. A detailed description of these processes is described in the section on auxin. The numbers in bold correspond with the numbers in (A) and they are unique call numbers in this paper.
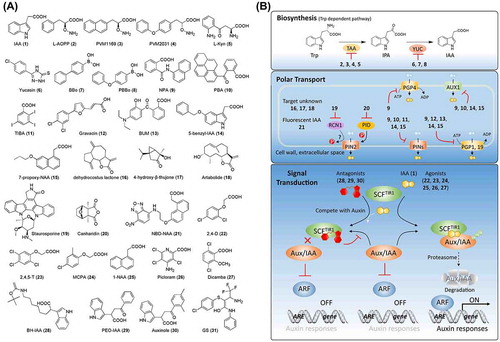
Auxin biosynthesis occurs via tryptophan-dependent and -independent pathways (reviewed by Zhao [Citation2], Korasick et al. [Citation3], and Kasahara [Citation4]). Auxin is principally synthesized in a tryptophan-dependent manner. As shown in (B), IAA is synthesized from l-tryptophan (Trp) via indole-3-pyruvate (IPA) by the tryptophan aminotransferase of Arabidopsis (TAA) and YUCCA (YUC) flavin-containing monooxygenase families [Citation4]. To date, only a few auxin biosynthesis inhibitors to target a tryptophan-dependent pathway have been reported. Soeno et al. [Citation5] reported that aminoethoxyvinylglycine (AVG, 82; (A)), an inhibitor of ACC synthase, which catalyzes a rate-limiting step in ethylene biosynthesis [Citation6], reduced the endogenous levels of IAA in an ethylene-independent manner. The authors also identified that l-aminooxyphenylpropionic acid (l-AOPP, 2) reduced the endogenous levels of IAA by targeting the Trp aminotransferase. However, these inhibitors are non-specific. Subsequently, the same group reported pyruvamine PVM1169 (also designated as KOK1169, 3), which is a more specific inhibitor than l-AOPP in Arabidopsis [Citation7]. Kakei et al. [Citation8] recently reported that PVM2031 (also designated as KOK2013, 4) outperforms PVM1169 in rice, indicating the species specificity of these inhibitors. A chemical screening aimed at identifying inhibitors of ethylene signaling identified l-kynurenine (l-Kyn, 5), which suppresses ethylene responses in Arabidopsis roots [Citation9]. Interestingly, further studies indicated that TAA1/tryptophan aminotransferase-related (TAA1/TAR), vital enzymes in auxin biosynthesis, are targets of Kyn, which acts as a competitive substrate of TAA1/TAR and thus, suppresses auxin biosynthesis [Citation9]. In addition to inhibitors of TAA1/TAR, 5-(4-chlorophenyl)-4H-1,2,4-triazole-3-thiol, referred to as yucasin (6), has been reported to be a potent inhibitor of IAA biosynthesis by inhibiting YUC1 in a competitive manner against the substrate IPA [Citation10]. Kakei et al. [Citation11] also developed a series of inhibitors that target YUC, including 4-biphenylboronic acid (BBo, 7) and 4-phenoxyphenylboronic acid (PPBo, 8). These inhibitors of TAA1/TAR and YUC are useful tools for characterizing the mechanisms of tryptophan-dependent IAA biosynthesis. In fact, in addition to the core pathway displayed in (B), other IAA biosynthetic pathways, such as the Trp-independent pathway, might exist and play important roles [Citation4]. Chemical biology might serve as a tool to investigate uncharacterized proteins involved in IAA biosynthetic pathways. For example, screening for chemicals that perturb endogenous levels of IAA or its precursors in tryptophan biosynthesis mutants might lead to the identification of chemical regulators of the Trp-independent pathway.
Polar transport of auxin is critical for its regulation of plant growth and development. To date, several classes of auxin transporters have been reported, including pin-formed proteins (PINs), auxin transporter 1 and auxin transporter-like (AUX1/LAX), and P-glycoproteins belonging to the ATP-binding cassette (ABC) family (PGP/ABCB) (reviewed in Grones and Friml [Citation12]). PINs function as auxin efflux transporters, while AUX1/LAX acts as an auxin influx carrier. PGPs are involved in both auxin efflux (PGP1/PGP19) and influx (PGP4) in an ATP-dependent manner [Citation13–Citation15]. Multiple auxin signal inhibitors, such as 1-N-naphthylphthalamic acid (NPA, 9), 1-pyrenoylbenzoic acid (PBA, 10), and 2,3,5-triiodobenzoic acid (TIBA, 11), have been confirmed as polar auxin transport inhibitors ((A) and (B)) [Citation16]. NPA and PBA target PINs and PGPs [Citation17,18], while TIBA might target AGR1/PIN2 [Citation19]. It is noteworthy that NPA was applied to a screening for NPA-resistant mutants, which identified seven TIR genes (TIR1 through TIR7) [Citation20,21]. Among them, TIR1 was finally confirmed to encode an auxin receptor [Citation22,23]. The search for auxin transport inhibitors has led to the identification of several PGP transporter inhibitors, including gravacin (12) that targets PGP19 and also affects PGP trafficking [Citation24,25], and 2-(4-diethylamino-2-hydroxybenzoyl)benzoic acid (BUM, 13) that might target PGP1 and PGP19 [Citation26]. To address the non-specificity of the above inhibitors, Tsuda et al. (2011) developed alkoxy-auxin analogs, such as 5-benzyl-IAA (14) and 7-propoxy-NAA (15), which block the auxin transport activity of PIN, PGP, and AUX1 without interfering with PIN trafficking or disturbing (Skp-Cullin-F-box) SCFTIR1 auxin signaling [Citation27]. By using the radish hypocotyl assay, dehydrocostus lactone (16), 4-hydroxy-β-thujone (17), and artabolide (18) were identified as polar auxin transport inhibitors, although their targets remain unknown [Citation28,29]. Besides direct inhibitors of auxin transporters, protein kinase inhibitors, such as staurosporine (19), and specific inhibitors of protein phosphatases 1 and 2A, such as cantharidin (20), have been reported to be involved in regulating auxin transport in a PIN2-dependent manner via PID kinase and RCN1-PP2A complex, respectively [Citation19,30]. For a better understanding of endogenous auxin distribution profiles, Hayashi et al. (2014) coupled auxin to 7-nitro-2,1,3-benzoxadiazole (NBD) to generate fluorescent auxin analogs, such as NBD-IAA and NBD-NAA (21), which remain active for auxin transport but are inactive for auxin signaling and metabolism [Citation31]. These fluorescent auxin analogs provide a new strategy for imaging the distribution of auxin in planta.
Owing to the identification of transport inhibitor response 1 (TIR1) as an auxin receptor, the auxin-signaling pathway was established [Citation22,23]. As shown in (B), auxin binds to the TIR1 receptor and promotes the interaction between TIR1 and Aux/IAA, a transcriptional repressor in auxin signaling, triggering the degradation of Aux/IAA by the proteasome. Subsequently, auxin response factor, which is repressed by Aux/IAA, is released and binds to auxin response elements to induce the transcription of auxin-responsive genes. As part of investigations addressing auxin, various synthetic chemicals have been developed and have contributed to basic studies of auxin signaling and its application in agriculture. In 1940, William Tempelman reported that a high concentration of IAA could suppress the growth of broadleaf plants, while having limited adverse effects on cereals, suggesting that IAA could be used as an herbicide. A search for auxin mimics with longer half-lives led to the identification of 2,4-dichlorophenoxyacetic acid (2,4-D, 22) and 2,4,5-trichlorophenoxyacetic acid (2,4,5-T, 23) ((A)). 2-Methyl-4-chlorophenoxyacetic acid (MCPA, 24), 1-naphthaleneacetic acid (1-NAA, 25), 4-amino-3,5,6-trichloro-2-pyridinecarboxylic acid (picloram, 26), and 3,6-dichloro-2-methoxybenzoic acid (dicamba, 27) were also developed as auxin-like plant growth regulators (PGRs) and herbicides. Recent studies have confirmed that some of these chemicals, despite having different structures, act as agonists of TIR1, [Citation23,32–34] ((A) and (B)). Calderon et al. (2012) [Citation35] reported that 1-NAA and 2,4-D display stronger bioactivity than IAA, although they have lower affinity for TIR1-Aux/IAA, which indicates that synthetic auxin transport, metabolism, and accumulation should be taken into consideration together with receptor affinity. From another point of view, these differences between natural and synthetic auxins can be exploited to study the native mode of action of natural auxin in planta. Hayashi et al. [Citation36] designed and synthesized a series of agonists and antagonists based on X-ray crystallographic analyses. The results showed that auxin derivatives with a substitution of methyl, ethyl, and n-propyl groups at the α position of the carboxylic acid retained auxin activity. Introducing n-butyl or a longer alkyl chain at this position led to chemicals with auxin-antagonistic activity. α-Butoxycarbonyl aminohexyl-IAA (BH-IAA, 28; (A)) displayed an especially strong antagonistic effect against auxin. Based on the co-crystal of TIR1 and IAA, it was suggested that IAA derivatives with n-butyl or longer alkyl chains introduce steric effects that prevent the interaction of TIR1 with Aux/IAA. Further structure–activity relationship (SAR) studies led to α-(phenylethyl-2-oxo)-IAA (PEO-IAA, 29) and α-(2,4-dimethylphenylethyl-2-oxo)-IAA (auxinole, 30), with stronger antagonistic activity owing to their interaction with the phenyl group Phe82 of TIR1, which increases their affinity for TIR1 [Citation37]. Germostatin (GS, 31) was identified in a chemical screening for inhibitors of seed germination [Citation38,39]. Further screening for GS-resistant mutants led to the identification of GSR1, encoding a tandem plant homeodomain finger protein. GSR1 was characterized as an auxin-mediated seed germination factor by forming a co-repressor with ARF16. GS, as a small non-auxin molecule, can be applied to investigate auxin-mediated seed germination [Citation38,39].
Gibberellins
Gibberellins (GAs) play important roles in plant development, including seed germination, vegetative growth, and reproduction. GAs are a group of diterpenoids that share the ent-gibberellane carbon skeleton. GAs were first isolated by Hori in 1926 from the fungal plant pathogen Gibberella fujikuroi (G. fujikuroi) that causes bakanae (“silly seedling” in Japanese) disease, which is characterized by excessive seedling elongation, causing lodging and infertility in rice. The studies described below revealed that the toxin secreted from G. fujikuroi affects plant growth and identify it as GA [Citation40].
The GA biosynthetic and catabolic pathways in higher plants have been well studied. GAs are synthesized from geranylgeranyl diphosphate (GGDP) by a series of enzymes, including ent-copalyl diphosphate synthase (CPS) and ent-kaurene synthase (that convert GGDP to ent-kaurene), ent-kaurene oxidase and ent-kaurenoic acid oxidase (that oxidize ent-kaurene to GA12), and GA20-oxidase (GA20ox) and GA3-oxidase (GA3ox) (that catalyze the conversion of GA12 to bioactive GAs, such as GA1 or GA4) (32, 33; (A) and (B)). Bioactive GAs undergo hydroxylation at the 2β position by a 2-oxoglutarate-dependent dioxygenase, GA2-oxidase (GA2ox), which leads to their deactivation ((B)) [Citation41]. Several types of plant growth retardants have been developed and confirmed to act as GA biosynthesis inhibitors [Citation42,43]. The triazole paclobutrazol (PBZ, 34) and uniconazole-P (UNI, 35) inhibit the oxidation of ent-kaurene to ent-kaurenoic acid to suppress GA production ((A) and (B)). These inhibitors are used to suppress plant height, thus increasing plant compactness and preventing lodging. Prohexadione-Ca (PHX, 36), a non-specific GA biosynthesis inhibitor, not only inhibits GA biosynthesis in rice at the 3β hydroxylation step of GA but also inhibits GA catabolism at 2β hydroxylation ((A) and (B)) [Citation44]. To stabilize bioactive GAs in plants, Otani et al. [Citation45] conducted a chemical screening to identify inhibitors of GA2ox, a deactivating enzyme of bioactive GAs. Methyl 6-chloro-3H-1,2,3-benzodithiazole-4-carboxylate 2-oxide (CBTC, 37; (A)) was identified as an inhibitor of GA2ox with high specificity in Arabidopsis. In addition to biosynthesis and catabolism, transport represents a level of regulation of plant hormones. To elucidate the spatial distribution of GA, Shani et al. [Citation46] modified GA3/GA4 by introducing a fluorescent moiety into its C6 position conjugated by amide linkage (GA4-Fl, 38; (A)). Application of GA-Fl facilitates the visualization of GA dynamic distribution, such as its preferential localization to the root endodermis, ATP-dependent uptake, and accumulation regulated by ethylene (81, (A)) through changes in GA transport [Citation46,47]. Later research identified NPF3 to be a GA transporter by using GA-Fl [Citation48].
Figure 2. Chemical structures of gibberellin-related chemicals and simplified schematic model of gibberellin biosynthesis, catabolism, transport, and signal transduction. A detailed description of these processes is described in the section on gibberellins. The numbers in bold correspond with the numbers in (A) and they are unique call numbers in this paper.
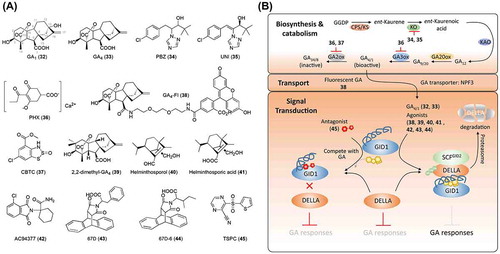
In 2005, gibberellin-insensitive dwarf 1 (GID1) was identified in rice as a GA receptor. Together with former discoveries, the GA signaling pathway has now been established. Upon binding of GA to the GID1 receptor, conformational changes in the lid of GID1 facilitate its interaction with SLR1, a rice DELLA protein. Then, SCFGID2 ubiquitinates SLR1, leading to the degradation of SLR1 [Citation49] ((B)). GID1 receptors have also been identified in Arabidopsis [Citation50]. Further analysis of the crystallography of GID1-GA3/GA4 provided insight into the SAR between GA and GID1, which partially explained why, among over 136 GAs identified, only a few (including GA1, GA3, and GA4), display bioactivities [Citation49]. In higher plants, hydroxylation of the 3β position is necessary for the bioactivities of GAs (e.g. GA1 and GA4), while 2β position hydroxylation plays a role in the deactivation of bioactive GAs. The introduction of two methyl groups at the 2β position of GA4, which prevents 2β hydroxylation, contributed to stronger and more durable activities of the product, 2,2,-dimethyl-GA4 (39; (A)) [Citation51,52]. Half a century ago, helminthoporol (H-ol, 40), which is secreted by Helminthosporium sativum, and helminthoporic acid (H-acid, 41) were reported to display GA-like activities in rice, although they do not possess an ent-gibberellane carbon skeleton ((A)) [Citation53–Citation55]. However, their mode of action remained elusive because of a lack of information on the GA signaling pathway at that time. A recent study demonstrated that H-acid promotes the interaction between GID1 and DELLA, triggers the degradation of RGA (a DELLA protein in Arabidopsis), and regulates the transcription of GA-responsive genes [Citation56]. A docking simulation of H-acid with OsGID1 (PDB: 3EBL) suggested that the carboxylate group of H-acid can be superimposed onto GA4, implying that H-acid can introduce hydrogen bonds into the side chains of GID1. Several hydrophobic interactions are also induced between H-acid and the lid of GID1, which facilitates the closure of the lid. These results, together with leaf growth-promoting effects of H-acid in rice, suggest that H-acid is an agonist of the GID1 receptor.
Various synthetic N-substituted phthalimides have been developed as alternatives for natural GAs [Citation57–Citation59]. For example, the phthalimide 1-(3-chlorophthalimido)-cyclohexanecarboxamide, also referred to as AC94377 (42), has GA-like effects on the germination of weed seeds and seedling growth in some crops [Citation57,59,60], despite the structural difference between AC94377 and GAs ((A)). A recent study demonstrated that AC94377 is an agonist of the GID1 receptor, which employs the same signaling pathway as GA in regulating seed germination and seedling establishment in Arabidopsis [Citation61]. Further studies addressing the physiological and molecular roles of AC94377 have indicated that AC94377 has selectivity for specific GID1s because it recognizes Ile-317 in the GID1 pocket. Interestingly, this amino acid is often replaced by valine in higher plant species or by histidine in Physcomitrella patens, which suggests why the biological activities of AC94377 differ from that of GA in many plants [Citation62]. A chemical screening was conducted to identify GA mimics that can promote seed germination [Citation63]. 67D (43) and one of its derivatives, 67D-6 (44), were shown to have GA-like activity in vivo. Further investigation revealed that these two chemicals can bind to the GID1 receptor and trigger the degradation of RGA, identifying them as agonists of GID1 ((A) and (B)). Except for GA3, which can be produced by fermentation with fungi, all bioactive GAs have been isolated from plants, which limits their application in agriculture because of the high production cost. Synthetic AC94377 and 67D, which can be simply synthesized at low cost, are good alternatives for GA. Further SAR studies based on the structures of AC94377 and 67D are expected to lead to the development of new agonists or even antagonists with selectivity for GID1. Recently, TSPC (45; (A)) was identified to be an antagonist of GID1 based on a chemical library screening [Citation64]. Together with the inhibitors of GA biosynthesis and catabolism, these agonists and antagonists of GID1 are expected to contribute to studies on GA signaling and delicate regulation of GA signaling in agriculture.
Cytokinins
Cytokinins (CKs) are a family of plant hormones that regulate multiple developmental and environmental responses of plants, including cell division, the formation of shoots from callus, and nutrient transport [Citation65,66]. A non-plant-derived CK was first isolated from decomposition products of DNA in a search for factors that promote cell division in plant tissue culture [Citation67]. Since then, screenings for natural CKs in plants have led to the identification of trans-zeatin (tZ, 46), isopentenyl adenine (iP, 47), and dihydrozeatin (48) ((A)), among others [Citation68–Citation71]. These CKs are adenine derivatives with small substituents at the N6 position.
Figure 3. Chemical structures of cytokinin-related chemicals and simplified schematic model of cytokinin biosynthesis, transport, and signal transduction. A detailed description of these processes is described in the section on cytokinins. The numbers in bold correspond with the numbers in (A) and they are unique call numbers in this paper.
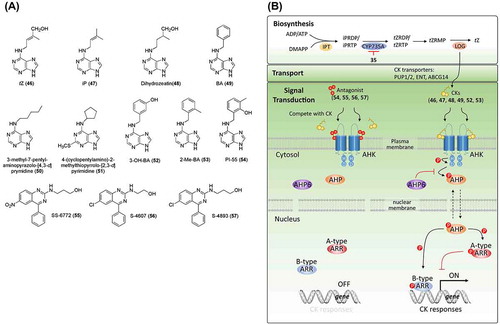
The biosynthesis of CK has been widely studied and has been reviewed by Sakakibara [Citation65], Frebort et al. [Citation72], and Zürcher [Citation73]. tZ, a major CK, is synthesized from dimethylallyl diphosphate and ADP/ATP by isopentenyltransferase (IPT) that produces isopentenyladenine (iP) nucleotide (iPRDP/iPRTP), CYP735A that catalyzes iP nucleotides to tZ nucleotides, and the phosphoribohydrolase lonely guy that converts inactive tZ nucleotides to the active tZ (B). An in silico screening of candidate CK biosynthesis inhibitors was carried out utilizing genome-wide gene expression profiling and prediction of target sites using global CK accumulation profile analysis [Citation74]. UNI (35; (A)), a cytochrome P450 monooxygenase inhibitor, was found to suppress tZ biosynthesis by targeting CYP735As in Arabidopsis. However, UNI has also been reported to inhibit the biosynthesis of GA, and no specific inhibitor of CK biosynthesis has yet been reported, possibly because of the importance of the biosynthesis and catabolism of adenine and its derivatives in basic life activities. CK conjugates, such as ribosyl CK derivatives (zeatin riboside and zeatin ribotide), are present in plants as intermediates in CK biosynthesis and are inactive as a result of scavenging excess active CKs [Citation75]. Compared to biosynthesis and signal transduction studies, studies addressing CK transport lag behind. Recently, three types of CK transporters have been reported, including purine permease 1/2 (PUP1/2), equilibrative nucleoside transporter (ENT), and G subfamily ATP-binding cassette 14 (ABCG14) (reviewed in Duran-Medina [Citation76]). PUP and ENT have been proposed to be involved in CK uptake, while ABCG14 functions as a CK exporter. PUP and ENT reportedly also transport other molecules. The functions of these CK transporters in planta remain elusive. Thus, the design of chemicals targeting these processes is expected to facilitate future studies addressing CK transport, as well as the development of novel CK regulators.
Recently, great progress has been made in the determination of the molecular mechanism of CK. By analyzing CK-insensitive mutants, cytokinin response 1 (CRE1)/Arabidopsis histidine kinase 4 (AHK4) were identified as CK receptors in Arabidopsis [Citation77]. AHK2 and AHK3 in Arabidopsis, as well as ZmHK1, ZmHK2, and ZmHK3 in Zea mays, are homologs of the CRE1/AHK4 receptors [Citation78–Citation81]. The CK receptor is an integral transmembrane protein with a cyclase/histidine kinase-associated sensory extracellular (CHASE) domain, histidine kinase domain, and receiver domain ((B)). The CK signal is transduced through two-component signal transduction systems that contain a histidine kinase as a sensor, and a response regulator. Ligand perception by CRE1/AHK4 in the CHASE domain activates autophosphorylation of the histidine kinase, in which the high-energy phosphate is transferred from a histidine to an aspartate residue (the receiver domain) in a process referred to as “phosphorelay.” The high-energy phosphate is further transferred to histidine-containing phosphotransfer protein (AHP) by phosphorylation of the conserved histidine residue in AHP. Phosphorylated AHP enters the nucleus and transfers high-energy phosphate to B-type Arabidopsis response regulator (ARR) by phosphorylation, which leads to the activation of primary responsive gene transcription. The CK signal transduction is complex because it involves multistep (His-Asp-His-Asp) phosphorelay. AHP6, as a pseudo-AHP without conserved histidine for phosphorelay, acts as a negative regulator of CK signaling. Type-A ARR possesses a small c-terminal that cannot trigger transactivation and is also a negative regulator, despite its conserved histidine for receiving high-energy phosphate [Citation82,83] ((B)).
Inspired by the discovery of kinetin, multiple studies have aimed to develop CK mimics through chemical modification of the kinetin molecule. N6-benzyladenine (BA, 49; (A)), which has an aromatic substituent at the N6 position, is a representative synthetic CK PGR. In-vitro ligand-binding tests have confirmed that BA shows high affinity for the CK receptor, which indicates that BA is an agonist of the receptor [Citation82]. Several chemicals, including pyrrolo[2,3-d]pyrimidines, pyrazolo[4,3-d]pyrimidines, s-triazines, N-benzyl-N 1-phenylureas, and N-arylcarbamates have been confirmed to have anti-cytokinin activity [Citation83]. However, studies on 3-methyl-7-pentylaminopyrazolo[4,3-d]pyrimidine (50) and 4-(cyclopentylamino)-2-methylthiopyrrolo[2,3-d]pyrimidine (51), which possess the strongest anti-cytokinin activity, have indicated that they show a poor affinity for CRE1/AHK4 and AHK3 in vitro and do not suppress ARR5 transcription [Citation84]. Further investigation of their mode of action indicated that, rather than acting as CK-receptor antagonists, these chemicals are cell-division inhibitors that cease the cell cycle by inhibiting cyclin-dependent kinase. Spichal et al. [Citation85] conducted SAR studies on BA by introducing substituents to its phenyl group. Assays of the in vitro binding affinity for CRE1/AHK4/WOL and in vivo tests of CK-induced ARR5 transcript levels have shown that 3-hydroxy-BA (3-OH-BA, 52) and 2-methyl-BA (2-Me-BA, 53) display CK activity at similar levels as BA, while 2-hydroxy-3-methyl-BA (PI-55, 54) displays strong antagonistic activities ((A)). These results indicate that, although the perception of CK by receptors is structurally strict, new agonists and antagonists could be developed by further SAR studies on BA. Arata et al. [Citation86] constructed a yeast strain that survives in a cytokinin-dependent manner by transforming cytokinin receptor CRE1 into yeast sln1 with histidine-kinase loss of function. In a high-throughput chemical screening (80,000 chemicals) for antagonists of CRE1/AHK4/WOL, two 4-phenylquinazoline derivatives, SS-6772 (55) and S-4607 (56), with similar structures ((A)), were identified. S-4983 (57), which was designed based on SS-6772 and S-4607, more strongly suppressed ARR5 transcription and promoted root growth. Further testing indicated that S-4983 allosterically inhibits the binding of [3H]iP to CRE1.
Recently, crystal structures of the CRE1/AHK4 CHASE sensor domain (residues 126–395) in complex with natural and synthetic CKs have provided further insight into the mechanism of ligand recognition [Citation87]. A complex structure with BA suggested that both the aromatic tail group of BA and the isoprenoid tail group of iP can fit the hormone-binding site without inducing major structural changes. Further comparison with the BA- and iP-bound structures revealed that Thr294 functions as a hydrogen-bond acceptor in the tail-binding pocket and introduces an additional hydrogen bond with the hydroxylated isopentenyl side chain of tZ, which explains why AHK4 perceives trans rather than cis zeatin-type CKs. Novel synthetic CKs are expected to be designed based on the above information.
Abscisic acid
Abscisic acid (ABA, 58; (A)) was first isolated and characterized from cotton during studies on the compounds responsible for fruit abscission [Citation88], which accounts for its name. Some studies have indicated that ABA, as a plant hormone, plays various physiological roles in plant growth and development, including seed dormancy and germination, cell division and elongation, floral transition, and responses to abiotic stresses, such as drought, salinity, cold, and UV radiation [Citation89]. Recent studies indicate that ABA also plays vital roles in plant responses to biotic stresses by regulating stomatal movement and crosstalk with other hormones, including jasmonic acid and salicylic acid [Citation90–Citation93].
Figure 4. Chemical structures of abscisic acid-related chemicals and simplified schematic model of abscisic acid biosynthesis, catabolism, transport, and signal transduction. A detailed description of these processes is described in the section on abscisic acid. The numbers in bold correspond with the numbers in (A) and they are unique call numbers in this paper.
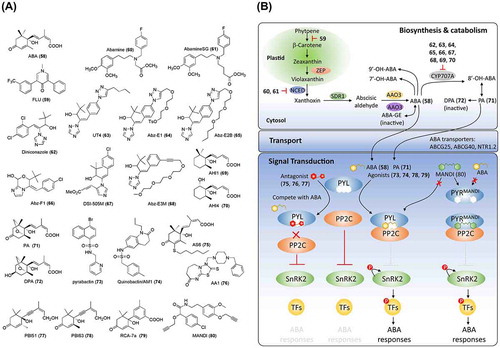
ABA biosynthesis and catabolism have been well studied (reviewed in Xiong and Zhu [Citation94], Nambara and Marion-Poll [Citation95], Finkelstein [Citation89], Endo et al. [Citation96], and Dong et al. [Citation97]). Multiple genes encode the enzymes for ABA biosynthesis from carotenoids. First, in the chloroplast, zeaxanthin epoxidase (ZEP), which is encoded by ABA1 in Arabidopsis, converts zeaxanthin to violaxanthin. Then, nine-cis-epoxycarotenoid dioxygenases (NCEDs) cleave the cis-isomers of violaxanthin and neoxanthin to xanthoxin. Finally, cis-xanthoxin is converted to active ABA by sequential reactions catalyzed by SDR1, which is encoded by ABA2, and AAO3 in the cytoplasm. This process requires the sulfation of AAO3 molybdenum cofactor by ABA3 ((B)). Fluridone (FLU, 59), an aquatic herbicide, inhibits carotenoid accumulation and ABA biosynthesis [Citation98]. A recently identified plant hormone, strigolactone (SL) (discussed later), also is derived from carotenoids [Citation99,100]. Thus, FLU is not an ideal candidate for studying ABA signals because it also inhibits SL biosynthesis [Citation101,102]. To regulate the endogenous ABA level, Han et al. [Citation103] developed a new inhibitor, abamine (60; (A)), which targets the ABA biosynthetic rate-limiting enzyme NCED [Citation104–Citation106]. SAR studies on abamine led to the identification of a much stronger and more specific inhibitor of NCED, named abamine-SG (61, (A)) [Citation107]. A series of sulfur- and nitrogen-containing compounds were developed as potential ABA biosynthesis inhibitors of AtNCED3 based on a modification of the sesquiterpenoid segment of the 9-cis-xanthophyll substrates and product [Citation108].
ABA homeostasis in plants also involves ABA catabolism. The first step in the main ABA-catabolic pathway is the hydroxylation of the 8′ position of ABA by a cytochrome P450 mono-oxygenase encoded by CYP707A family genes in Arabidopsis ((B)) [Citation109–Citation111]. UNI (35, (A)), which inhibits the GA biosynthesis enzyme (ent-kaurene oxidase), was reported to inhibit ABA catabolism in cultured tobacco Bright Yellow-2 cells [Citation112,113]. SAR studies of UNI led to the identification of diniconazole (62, (A)), which has inhibitory activity against CYP707A3, an ABA 8′-hydroxylase [Citation112]. To develop specific CYP707A inhibitors, Todoroki and colleagues focused on modifying the structure of UNI, which led to UT4 (63), abscinazole (Abz)-E1 (64) and AbzE2B (65) by enlarging UNI with C4 alkyltriazole [Citation114–Citation116], Abz-F1 (66) by restricting conformation of UNI [Citation117], and DSI-505 M (67) by modifying the azole ring [Citation118] ((A)). These results indicate that SAR studies on UNI could lead to specific inhibitors of CYP707A. Recently, by replacing the 1,2,3-triazolyl ring of Abz-E2B with a triple bond, Takeuchi et al. [Citation119] developed a better candidate, Abz-E3 M (68), with shorter synthesis steps and higher yields. In addition to the azole inhibitors, AHI1 (69) and AHI3 (70), with similar structures to ABA, were reported to be inhibitors of CYP707A3, which assists in the design of non-azole inhibitors [Citation120] ((A) and (B)). The unstable 8′-OH ABA is cyclized to form phaseic acid (PA, 71) and is subsequently reduced to dihydrophaseic acid (DPA, 72) and epi-DPA ((A) and (B)). Hydroxylation at the 7′ or 9′ position of ABA, which produces 7′-OH ABA and 9′-OH ABA, respectively, has also been reported. Except for DPA, all above-mentioned catabolites remain active, although their activities are weaker than that of ABA. In fact, a recent study has shown that PA, as a catabolite of ABA, can be perceived by a subset of ABA receptors and functions as a hormone in seed plants [Citation121]. The identification of an ABA metabolite as a hormone introduces a new perspective for the design of functional analogs of ABA.
In addition, although there is an ongoing debate, ABA glycosylation by glucosyltransferase, which produces ABA glucosyl ester [Citation122,123], as well as ABA hydrolysis by β-glucosidases, have been proposed to affect ABA homeostasis and transport [Citation124–Citation126]. Recently, several ABA transporters have been identified, including the ABC transporters AtPDR12/ABCG40 [Citation127] and ABCG25 [Citation128], and the NRT1/PTR protein, AIT1/NRT1.2 [Citation129]. These transporters play significant roles in the spatiotemporal functions of ABA. Chemicals targeting ABA glycosylation enzymes and transporters are expected to be useful in regulating local and distant levels of bioactive ABA.
The ABA signaling pathway was initially established by the identification of PYR/PYL/RCAR (hereafter referred to as PYL), a group of soluble ABA receptors, by two independent groups [Citation1,130]. ABA binds to PYL and promotes conformational changes in the gate and latch loops in PYL, which facilitates its interaction with PP2C, a phosphatase that interacts with and inactivates the kinase SnRK2 through dephosphorylation. It is proposed that the catalytic site of PP2C is sealed by the interaction with the ABA-PYL complex and that PP2C subsequently loses its SnRK2-inhibitory effect. When released from the inhibition by PP2C, SnRK2s phosphorylate themselves and their targets (such as transcription factors and ion-channel proteins) to induce ABA responses [Citation131]. Chemical regulators have contributed to ABA signaling studies. Pyrabactin (73, (A)), an ABA mimic, was used to screen for resistant mutants, which led to the identification of a pyrabactin resistant (PYR1) loss-of-function mutant. PYR1 and 14 homologs were subsequently identified as ABA receptors. Pyrabactin, as a selective agonist for PYL, overcomes the redundancy of the 14 ABA receptor homologs. Recently, based on chemical screening, two research groups simultaneously reported that sulfonamide (a dihydroquinolinone, initially named quinabactin and AM1 by the respective groups) (74; (A)) is an agonist of PYL [Citation132,133]. Quinabactin/AM1 promotes the interaction between PYL and PP2C, inhibits seed germination, and prevents leaf water loss. Based on the crystal structure of the PYL-ABA-PP2C complex [Citation134–Citation137], Takeuchi et al. [Citation138] noticed that there is a tunnel above the ABA 3′ ring CH, which opens at the PP2C-binding interface. A series of 3′-alkylsulfanyl ABAs were synthesized with different alkyl chain lengths and were tested both in vivo and in vitro. This approach revealed that AS6 (75, (A)) (generated by the addition of a six-carbon alkyl to ABA) functions as a PYL antagonist by interrupting the interaction between PYL and PP2C. Recently, a broad-spectrum ABA antagonist 1 (AA1, 76; (A)) of PYL was identified by chemical genetic screening [Citation139]. Two decades ago, Wilen [Citation140] developed two enantiomers of acetylenic ABA, PBI-51 (77) (with antagonistic activity) and PBI-63 (78) (with agonistic activity) ((A)). ABA analogs with a phenyl group substitution in the ABA side chain were reported by Asami et al. [Citation141]. Further studies identified RCA-7a (79; (A)) as a strong ABA analog that can be synthesized by a facile and inexpensive one-tube reaction [Citation142,143]. The chemicals reported by Wilen et al. and Asami et al. provide valuable information for ABA analog development. Interestingly, by engineering the ABA receptor PYR1, Park et al. [Citation144] generated the PYR1MANDI receptor for mandipropamid (MANDI, 80), a commercially available agrochemical, enabling the control of ABA responses and drought tolerance in transgenic plants ((A) and (B)). This work demonstrates the power of chemical genetics and paves the way for designing and utilizing the chemicals in other plant hormone signals. PP2C and SnRK2 play important roles in transducing ABA signals through dephosphorylation and phosphorylation [Citation145–Citation150]. Chemicals selectively targeting these two protein families are expected to be alternative regulators of ABA signals.
The above results suggest that further rational modification of ABA based on SAR studies and structural biology will lead to better ABA analogs and inhibitors for ABA biosynthesis or catabolism. In addition to the scaffold of ABA, chemical screening is expected to provide novel chemical scaffold for developing ABA mimics or inhibitors of enzymes involved in ABA biosynthesis, catabolism, and signal transduction. These chemicals are expected to be useful for manipulating the endogenous ABA level to regulate plant growth, development, and abiotic and biotic stress tolerance.
Ethylene
In the 19th century, streetlights used coal gas as a fuel. It was observed that trees around the gas lamps defoliated more extensively than others. Ethylene was identified as the active compound in coal gas that affects plant growth [Citation151]. Ethylene (ET, 81; (A)) is a gaseous plant hormone that regulates seed germination, seedling triple responses, abscission, fruit ripening, and leaf and flower senescence [Citation152]. Because of the multiple roles of ET in plant growth and development, there is ample opportunity for the development of novel methodologies for regulating ET biosynthesis and signaling with application potential in agriculture.
Figure 5. Chemical structures of ethylene-related chemicals and simplified schematic model of ethylene biosynthesis and signal transduction. A detailed description of the processes is described in the section on ethylene. The numbers in bold correspond with the numbers in (A) and they are unique call numbers in this paper.
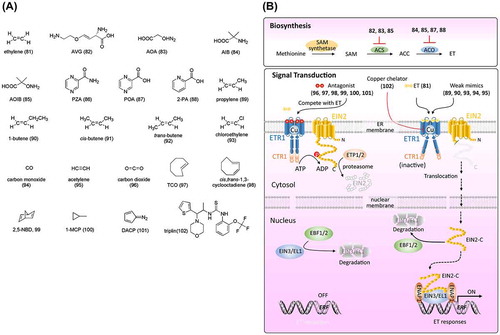
Among the plant hormones identified, ET has the simplest structure and is synthesized by a simple two-step conversion of S-adenosylmethionine (SAM), which originates from the processing of methionine by SAM synthetase [Citation152,153]. Generally, SAM is first converted into 1-aminocyclopropane-1-carboxylic acid (ACC) by ACC synthase (ACS). ACC is subsequently oxidized to ET by ACC oxidase (ACO) [Citation153] ((B)). Rhizobitoxine, a phytotoxin produced by bacteria, was reported to inhibit ET biosynthesis in sorghum seedlings and senescent apple tissues [Citation154]. Boller et al. [Citation155] and Yu et al. [Citation156] reported that aminoethoxyvinylglycine (AVG, 82; (A)), a structural analog of rhizobitoxine, blocks the conversion of SAM to ACC by a pyridoxal enzyme, which was identified as ACS. AVG is not a specific inhibitor of ET biosynthesis (it also suppresses auxin biosynthesis) [Citation5]. Aminooxyacetic acid (AOA, 83; (A)), a general inhibitor of pyridoxal phosphate-dependent enzymes, also inhibits ET biosynthesis by targeting ACS [Citation157]. α-Aminoisobutyric acid (AIB, 84; (A)), a structural analog of ACC, acts as a competitive inhibitor of ACO [Citation158]. 2-Aminooxyisobutyric acid (AOIB, 85; (A)), an analog of both AOA and AIB, inhibits ACS and ACO [Citation159]. The cobalt ion was identified to be a non-competitive inhibitor of ET [Citation160]. Despite their non-specific effects and low efficacy, the above-mentioned regulators have contributed to the study of ET biosynthesis. Recently, by chemical genetics screening in Arabidopsis, pyrazinamide (PZA, 86) was identified as a specific ET biosynthesis inhibitor that targets the final step mediated by ACO [Citation161]. Further studies confirmed that PZA is not active, but its metabolite pyrazinecarboxylic acid (POA, 87) and its structure-related chemical 2-picolinic acid (2-PA, 88) function as direct inhibitors of ACO ((A) and (B)). A SAR study, together with X-ray crystallography of the POA-ACO complex, revealed the importance of the carboxylic group and the nitrogen for POA to be an effective inhibitor of ACO, and 2-PA was identified as a stronger inhibitor, which highlights the prospects for further SAR studies based on POA and 2-PA [Citation161].
Genetic studies of Arabidopsis mutants that respond differently to ET than do wild-type plants, together with chemical biology studies using the above-mentioned ET functional regulators, have established most of the ET signaling pathway. ET receptors have been shown to localize to the endoplasmic reticulum (ER) system, including ethylene response 1 (ETR1) and ethylene-response sensor 1 (ERS1), which belong to subfamily I, and ETR2, ethylene-insensitive 4 (EIN4), and ERS2, which belong to subfamily II (ETR1 as a representative is exemplified here). As shown in (B), in the absence of ET, ETR1 binds to and activates CTR1, which phosphorylates the C-terminal domain of EIN2. The phosphorylation of EIN2 triggers its degradation via ETP1/2 and the proteasome. Simultaneously, EIN3/EIL in the nucleus are targeted for proteasome degradation via the F-box protein EBF1/2, which results in the absence of ET-related gene expression and, thus, an absence of ET responses. Upon ET binding, ETR1 undergoes structural changes and loses its kinase activity for activating CTR1. Without phosphorylation by CTR1, the C-terminal domain of EIN2 (EIN2-C) is cleaved by an unknown protease and translocates to the nucleus, where EIN2-C triggers EBF1/2 degradation by the proteasome and promotes the accumulation of EIN3/EIL1. EIN3/EIL1 activates transcriptional cascades (such as ERF1) and induces ET responses [Citation152,162,163]. Recent studies have demonstrated that EIN2-C interacts with EIN2 nuclear-associated protein 1 (ENAP), which promotes EIN3 binding to the target motif via histone acylation of H3K14 and H3K23 [Citation164,165] ((B)). Although the mode of action of ET on its receptor remains unclear, a series of chemicals related to ET perception have been reported. By observing the effects of propylene on hypocotyl growth in pea, Burg and Burg [Citation166] showed that propylene (89) has one-hundredth the biopotency of ET. Although 1-butene (90) showed weak activity in inhibiting hypocotyl growth, neither cis- (91) nor trans-2-butene (92) displayed bioactivity ((A) and (B)). Other modifications, including the introduction of chloride on the end carbon of ET (chloroethylene, 93; (A)), replacement of carbon with oxygen (CO, 94; (A)), and replacement of the double bond with a triple bond (95, acetylene; (A)) led to dramatic loss of bioactivity (below one thousandth). These results indicate that the structural perception of ET is strictly confined to its receptors. Despite their low bioactivity, these chemicals are assumed to be agonists of ET receptors. A study by Burg and Burg [Citation166] indicated that CO2 (96, (A)), as a competitive inhibitor, has antagonistic activity against ET. Interestingly, chemicals with cyclic structures, such as trans-cyclooctene (TCO, 97), cis,trans-1,3-cyclooctadiene (98), and 2,5-norbornadiene (2,5-NBD, 99), were reported to be ET receptor antagonists ((A)). The antagonistic activities of TCO and 2,5-NBD were confirmed in planta [Citation167] as well as in vitro by a competitive binding test for ETR1 [Citation168]. Because of its irreversible binding activity to ET receptor [Citation169], 1-methylcyclopropene (1-MCP, 100; (A)) has strong and long-lasting antagonistic activity against ET and thus, has been developed into products for preventing senescence and abscission of plant organs in gardening (e.g. EthylBlocTM and SmartFreshTM). Another chemical, diazocyclopentadiene (DACP, 101; (A)), with a diazo group, was confirmed to be an effective ET receptor inhibitor by blocking ET binding [Citation170]. The photolytic product of DACP, despite failed attempts at its identification, was shown to be effective at counteracting ET [Citation171]. However, the explosiveness of DACP limits its application. Neatly all chemicals with antagonistic activities against ET are volatile, which limits their application. Although the formulation of gaseous 1-MCP as a powder in complex with cyclodextrin (which releases 1-MCP when dissolved in water) permits its application, there is an urgent need for non-volatile chemicals. Chemical screening for non-volatile compounds that inhibit the binding activity of ET for its receptor is expected to identify allosteric antagonists.
The above-mentioned chemical regulators can serve as effective tools for studying ET biosynthesis and perception. For example, Hirayama et al. [Citation172] conducted a mutant screening aiming to uncover novel factors in ET signaling by using TCO (97). An Arabidopsis mutant, responsive-to-antagonist 1 (ran1), was found to display resistance to TCO. Further genetic studies demonstrated that RAN1 encodes a protein that functions as a copper transporter to create a functional ET receptor. Surprisingly, TCO was perceived by the ET receptor as an agonist, rather than an antagonist, in ran1. Hirayama et al. [Citation172] assumed that reduced delivery of copper ions to the ET receptors in ran1 might alter the receptor conformation, resulting in reduced ligand specificity. In summary, TCO as a chemical regulator contributed to clarifying the role of copper in ET perception. In fact, the existence of metal in association with the ET receptor was assumed by Burg and Burg [Citation166] and Thompson et al. [Citation173]. A quite recent chemical genetic screening identified triplin (102), a novel specific copper chelator that causes a triple response phenotype through the ET signaling pathway [Citation174] ((A) and (B)). By applying triplin to manipulate the concentration of copper ions, the interaction between RAN1 and ATX1 was discovered and confirmed to contribute to copper ion transport to the ET receptor.
Brassinosteroids
Brassinosteroids (BRs) are a family of plant steroid hormones that includes brassinolide (BL, 103; (A)) as the first identified BR. BRs regulate multiple aspects of plant growth and development, including seed germination, seedling photomorphogenesis, timing of flowering, male fertility, and responses to environmental stresses [Citation175,176]. Mutants with defects in BRs display a dwarf phenotype, dark green and rounded leaves, prolonged life-span, poor fertility, and altered vascular development [Citation175].
Figure 6. Chemical structures of brassinosteroid-related chemicals and simplified schematic model of brassinosteroid biosynthesis and signal transduction. A detailed description of the processes is described in the section on brassinosteroids. The numbers in bold correspond with the numbers in (A) and they are unique call numbers in this paper.
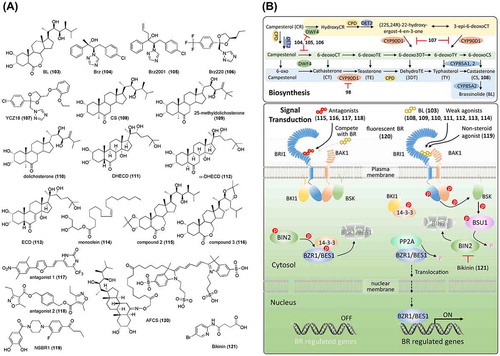
By characterizing a series of Arabidopsis dwarf mutants (DWF), several genes involved in BR biosynthesis were identified, including deetiolated 2 (DET2)/DWF6 encoding a 5α-reductase [Citation177], constitutive photomorphogenesis dwarfism (CPD)/DWF3 encoding CYP90, a cytochrome P450 [Citation178], DWF4 encoding a 22-alpha-hydroxylase, a cytochrome P450 monooxygenase [Citation179], DWF1 encoding an enzyme for the conversion of 24-methylenecholesterol to campesterol [Citation180], and DWF7/STEROL1(STE1) encoding delta 7-sterol-C-5-desaturase [Citation181,182]. These findings have contributed to our understanding of the BR biosynthetic pathways, including, as shown in (B), early C6 oxidation (blue background), late C6 oxidation (green background), and early C22 hydroxylation (yellow background) [Citation183,184]. Mutation analysis in Arabidopsis also identified a BR-insensitive mutant (bri1) [Citation185]. BRI1, as a serine/threonine kinase, was later confirmed to be a membrane-localized receptor of BR [Citation186–Citation189].
To chemically control the BR level at different developmental stages in the plant to enable the study of BR functions and signaling factors, a chemical screening was conducted aimed at identifying BR biosynthesis inhibitors [Citation190]. A triazole chemical, named brassinazole (Brz, 104), was identified as a potent chemical that induced dwarfism with the typical BR-deficient mutant phenotype, which could be rescued by BR (but not GA) treatment, indicating that Brz is a specific inhibitor of BR biosynthesis. Further studies have shown that Brz inhibits the hydroxylation of the C-22 position of the side chain in BRs by direct binding to DWF4, a cytochrome P450 monooxygenase of the BR biosynthetic pathway ((A) and (B)) [Citation191]. As part of a SAR study of Brz, a more specific BR biosynthesis inhibitor was developed; Brz2001 (105, (A)) [Citation192]. Another chemical screening for azole derivatives that inhibit BR biosynthesis identified Brz220 (106, (A)) as a potent inhibitor with stronger activity and fewer side effects than Brz and Brz2001 [Citation193]. A series of triazole derivatives, based on the ketoconazole scaffold, and YCZ18 (107, (A)), a triazole, were designed and confirmed to be potent BR biosynthesis inhibitors targeting CYP90 [Citation194–Citation198]. Brz, as the first BR biosynthesis inhibitor identified, has been widely used in BR studies and facilitated the identification of several vital factors in BR signaling. Arabidopsis plants treated with Brz display photomorphogenesis in the dark, with a shorter hypocotyl and open cotyledons. A mutant screening to identify mutants with a longer hypocotyl and cotyledon closure in the presence of Brz led to the identification of the Brz-resistant (bzr1) and Brz-insensitive-long hypocotyl (bil1) mutants, which each carry a mutation in the same gene. bzr1/bil1 was characterized as a dominant mutant and thus named bzr1-1D/bil1D. Later studies indicated that BZR1/BIL1 encodes a transcription factor that positively regulates BR signaling [Citation199,200]. Since then, several BR-related genes have been identified based on screenings using Brz, including BIL2, which encodes mitochondrial-localized DnaJ/heat shock protein 40 (DnaJ/Hsp40) family and promotes ATP synthesis in mitochondria [Citation201], BIL4, which encodes a seven-transmembrane-domain protein and regulates the localization of BRI1 [Citation202,203], and BSS1 (BRZ-sensitive-short hypocotyl 1), which encodes the BTB-POZ domain protein and inhibits transport of BZR1/BIL1 by forming a complex [Citation204].
The BR signaling pathway has been well studied using genetic and chemical mutant screenings, as shown in B (reviewed in Wang et al. [Citation205]; Belkhadir, Jaillais [Citation206]; Nolan et al. [Citation207]). BR functions by interacting with the amino acids of the leucine-rich repeat (LRR) domain of BRI1 [Citation208]. Seventy analogs of BR, including BL, the most potent natural BR, and castasterone (108, CS; (A)), a precursor of BL, have been reported [Citation209]. In fact, CS also displays BR-like activity, although it is weaker than that of BL, which is attributed to the weaker affinity of CS to BRI1 than that of BL. To obtain agonists with stronger BR activity or antagonists against BR signaling, several synthetic BRs have been tested in biological assays, including lamina inclination in rice, epicotyl elongation in Azuki bean, and leaf unrolling in wheat [Citation210–Citation213]. Kim et al. found that 25-methyldolichosterone (109) shows one order of magnitude higher BR activity than dolichosterone (110, (A)) [Citation214]. Based on this result, BL was modified by introducing a methyl C-25, which improved its activity [Citation215]. Further SAR studies on the modification of side chains in BRs suggested that hormonal activity in plants is governed by the structure of the side chain, rather than by the nature of the steroidal core skeleton [Citation209,216]. Recently, Thussagunpanit et al. [Citation217] reported that 7,8-dihydro-8α-20-hydroxyecdysone (DHECD, 111) and 7,8-dihydro-5α,8α-20-hydroxyecdysone (α-DHECD, 112), which are structurally similar to BL ((A)), have functional activities similar to those of BR. DHECD and α-DHECD can be produced with phytoecdysteroid 20-hydroxyecdysone (ECD, 113; (A)), which is abundant in Vitex glabrata, a common plant in Thailand. These synthetic ecdysteroid analogs provide reasonable-cost alternatives for the application of BR during plant growth. In addition to steroids, chemicals without steroidal structures have been reported. Two auxin derivatives, methyl 4-chloroindole-3-acetate (4-Cl-IAA-Me) [Citation218] and 2,4-dichlorophenoxyacetate (2,4-D-Me) [Citation219], were found to influence lamina inclination. However, their activities disappeared when these compounds were converted to free acids. The modes of action of 4-Cl-IAA-Me and 2,4-D-Me as BR-like chemicals remain unclear. Park et al. [Citation220,221] isolated a monoglyceride, monoolein (114, (A)), and confirmed that it displays BR-like activities, although the activity is weaker than that of BL. Although the mode of action of monoolein has yet to be determined, its structural resemblance to one of the BLs suggests that it binds to the BRI1 receptor. Recently, following technological advances and improved understanding of BR signaling, several chemicals were rationally developed by targeting BRI1. Muto and Todoroki [Citation222] designed multiple BL acetonides based on the 3-D structure of BRI1-bound BL and demonstrated that BL could be converted to an antagonist through acetonide functionalization of the 2,3-dihydroxyl group (compound 2, 115; (A)) and the 22,23-dihydroxyl group (compound 3, 116; (A)). A pharmacophore-based in silico screening of 5 million chemicals using the crystal structure of the BRI1-BL-SERK1 complex identified 22 non-steroidal candidates, of which several antagonists, such as antagonist 1 (117) and antagonist 2 (118) ((A)), were confirmed to inhibit lamina inclination in rice, which could be recovered by BL treatment [Citation223]. However, no agonists were identified, indicating the strict selectivity of BRI1 for its ligands. Sugiura et al. [Citation224] conducted an in silico analysis, including docking simulation and molecular dynamics, based on the antagonists reported by Takimoto et al. [Citation223]. The designed chemicals were synthesized and their activities were tested under co-treatment with Brz in Arabidopsis. As a result, a non-steroidal BL-like chemical, NSBR1 (119, (A)), was developed by considering the ligand–receptor binding energy based on the molecular dynamics simulation [Citation224]. To understand the BR spatial and temporal distribution, a bioactive fluorescent BR analog, Alexa Fluor 647-castasterone (AFCS, 120; (A)), was synthesized and aided in revealing BRI1 signaling from the plasma membrane in living Arabidopsis cells [Citation225].
Except for the BRI1 receptor, other factors in BR signaling are alternative regulatory points. A chemical screening for agents inducing constant BR-responses identified a GSK3 BIN2 inhibitor, named bikinin (121; (A)), that directly binds to GSK3 BIN2 and functions as an ATP competitor [Citation226]. The SAR studies on bikinin by Rohzon et al. [Citation227] provided a better understanding of the relationship between structure and inhibitory effects.
Jasmonic acid
Jasmonic acid (JA) is an important plant hormone that regulates plant responses to biotic and abiotic stresses, including herbivory and wounding caused by insects and pathogens, plant elongation growth, plant inflorescence, and senescence [Citation228–Citation232]. Methyl jasmonate (MeJA, 122; (A)) was first isolated from the jasmine flower as one of the main odor components. In the early 1980s, the existence of JA as a free acid was reported [Citation233–Citation235]. Following analyses of JA biosynthesis mutants and JA insensitive mutants in 1990, JA was recognized as a plant hormone. JA displays cis and trans isomeric forms (123 and 124 respectively; (A)), depending on the steric structure of the C5 side chain and acetate moiety. It is thought that cis-JA (with higher bioactivity and more fragrance than its trans isomer) is biosynthesized first and then converted to the trans isomer, which is much more stable.
Figure 7. Chemical structures of jasmonic acid-related chemicals and simplified schematic model of jasmonic acid biosynthesis and signal transduction. A detailed description of the processes is described in the section on jasmonic acid. The numbers in bold correspond with the numbers in (A) and they are unique call numbers in this paper.
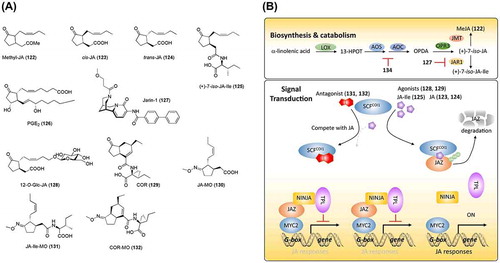
The JA biosynthesis pathway has been extensively studied. As shown in (B), the original substrate of JA biosynthesis is α-linolenic acid, which is a polyunsaturated fatty acid released from the galactolipids of the chloroplast membrane. An oxygen is inserted into the C-13 position of α-linolenic acid by lipoxygenase (LOX), which produces 13-hydroperoxyoctadecatrienoic acid (13-HPOT). The fatty acid hydroperoxides are cyclized by allene oxide synthase (AOS) and allene oxide cyclase (AOC) to produce 12-oxophytodienoic acid (OPDA), which is oxidized by OPDA reductases (OPRs) to produce the cyclopentanone ring, a characteristic structure of jasmonates. The last step to form bioactive jasmonate, (+)-7-iso-JA-Ile (125), is catalyzed by JAR1, a JA-amino acid synthetase ((A) and (B)) [Citation228,236–240]. Although regulation of JA biosynthesis is expected to facilitate the study of JA biosynthesis and downstream signals, few regulators of JA biosynthesis have been reported. Because JA and mammalian eicosanoid prostaglandin E2 (PGE2, 126; (A)) have similar chemical structures and biosynthetic pathways, aspirin (134; (A)), a well-known medicine, was applied to investigate its effects on JA biosynthesis [Citation241]. Aspirin was found to inhibit the hydroperoxide-dehydrase that converts 13-HPLA/13-HPOT to OPDA. Following this finding, Meesters et al. [Citation242] conducted a chemical screening using a transgenic line with a luciferase reporter driven by the promoter of the JA marker gene LOX2. A chemical, jasmonate response inhibitor-1 (jarin-1, 127; (A)), was found to inhibit the JA signals induced by MeJA. Further studies suggested that jarin-1 prevents the biosynthesis of JA-Ile by targeting JAR1. Similar to other plant hormones, JA metabolism is involved in JA signaling by regulating the homeostasis of bioactive jasmonates. JA metabolism includes conjugation with isoleucine catalyzed by JAR1 [Citation243], methylation by a methyltransferase [Citation244], hydroxylation and carboxylation by CYP94 enzymes [Citation245–Citation247], O-glucosylation [Citation248,249], and sulfation by sulfotransferases [Citation250]. In fact, in addition to JA-Ile, other JA metabolites have been reported to have bioactivities. For example, 12-O-β-D-glucopyranosyl JA (12-O-Glc-JA, 128; (A)) was reported to be a leaf-closing factor that induces nyctinastic leaf closure in Samanea saman by targeting a membrane protein, membrane target protein of jasmonate glucoside [Citation251–Citation253]. Further studies demonstrated that 12-O-Glc-JA, rather than other jasmonate derivatives, displays leaf-closing activity and indicated a novel mode of action for jasmonates (other than the well-established COI1-JA-Ile-JAZ route) [Citation254]. Thus, the development of chemicals that target various known and unknown factors is expected to create opportunities for controlling JA homeostasis and to provide tools for JA studies.
Figure 8. Chemical structures of salicylic acid-related chemicals and simplified schematic model of salicylic acid biosynthesis, catabolism, transport, and signal transduction. A detailed description of these processes is described in the section on salicylic acid. The numbers in bold correspond with the numbers in (A) and they are unique call numbers in this manuscript.
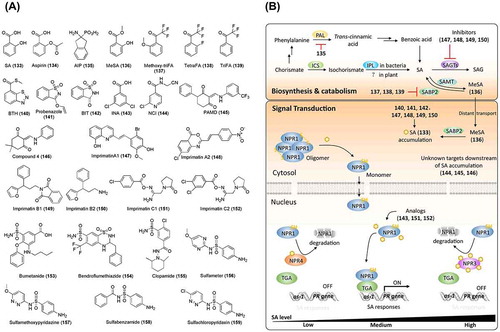
Chemical genetic studies contributed to the identification of the JA receptor. Coronatine (COR, 129; (A)), known as a phytotoxin, and which has physiological roles highly similar to those of JA, was isolated from the pathogen Pseudomonas syringae pv. atropurpurea [Citation255]. The stereo-structure of COR is similar to that of JA-Ile. Turner et al. isolated an Arabidopsis mutant that is insensitive to COR. Xie et al. [Citation256] characterized this loss-of-function mutant and identified its causal gene, which encodes an F-box protein belonging to E3 ubiquitin ligase complex, named coronatine insensitive 1 (COI1). Although COI1 was considered a JA receptor, its mechanism remained elusive at that time. Subsequent studies identified MYC2 as a transcription factor that regulates JA-responsive genes [Citation257,258], and jasmonate ZIM domain (JAZ) that represses the transcription of jasmonate-responsive genes through its interaction with MYC2 [Citation259,260]. Thus, the JA signaling pathway was established, as shown in (B). Briefly, JA-Ile as bioactive JA binds to the COI1 receptor and promotes the interaction of COI1 with JAZ, leading to the polyubiquitination and subsequent degradation of JAZ by the 26S proteasome [Citation228,261]. Yamane et al. [Citation233] conducted SAR studies on JA derivatives and assumed that some of the derivatives might function as an antagonist against JA-Ile in plants. The crystallization of the COI1-JAZ complex provided a mechanistic view on JA-Ile perception, which further serves for the development of chemical regulators, such as novel agonists and antagonists [Citation262]. Crystallization indicated that the keto-group of JA in JA-Ile and the COOH-group of Ile interact with the JAZ domain, which explains why (+)-7-iso-JA-Ile (125; (A)) is the most bioactive ligand for COI1 [Citation263]. On the basis of the crystallographic structure, O-methyloxime derivatives, including JA-MO (130), JA-Ile-MO (131), and COR-MO (132), were rationally designed ((A)) [Citation264]. COR-MO was confirmed to be an antagonist of COI1 that impedes the interaction between COI1 and JAZ, JAZ degradation, and the physiological roles of JA-Ile and COR in Arabidopsis. Although JA signaling is important for plant defense against insects, certain pathogens can hijack the COI1 receptor, increasing plant susceptibility. To prevent this pathogen hijacking, Zhang et al. [Citation265] genetically modified the COI1 receptor for it to specifically perceive JA-Ile but not COR. Structural modeling based on the crystallographic structure of COI1-JAZ1 was used to direct the modification. Finally, substitution of Ala-384 with valine in COI1 was shown to result in low affinity for COR (one hundredth) without changing its affinity for JA-Ile in in vitro experiments. COI1A384 V transgenic plants acquired resistance to the COR-secreting pathogen, Pseudomonas syringae pv. tomato strain DC3000, and maintained a high-level defense against insects. This achievement exemplifies the power of combining chemical biology and genetic approaches.
Salicylic acid
Salicylic acid (SA, 133; (A)) is a phenolic compound in plants that exists in various conjugate forms that are generated by glycosylation, methylation, and hydroxylation. Salicin, a glucoside of an SA derivative, was first isolated from Salix (willow) bark extract in 1828 and has been used for centuries as a pain reliever. In 1890, acetylsalicylic acid, named as aspirin (134), was introduced by Bayer as a commercial drug for the treatment of pain, fever, and inflammation [Citation266]. Numerous studies have shown that SA is a plant hormone that plays important roles in plant defenses, including local resistance to pathogens and systemic acquired resistance (herein referred to as “SAR response” to differentiate it from structure-activity relationship, which is also abbreviated as SAR) [Citation267–Citation272]. SA is also reported to regulate plant growth and development [Citation273], plant responses to abiotic stresses [Citation274–Citation277], and autophagy, which controls programmed cell death [Citation278–Citation280].
In plants, SA is proposed to be biosynthesized by two pathways that utilize chorismate as a substrate [Citation281,282]. In the isochorismate synthase (ICS)-dependent pathway, chorismate is converted into isochorismate by ICS [Citation283] and isochorismate is subsequently converted to SA by isochorismate pyruvate lyase (IPL) (reported in bacteria, but has not yet been identified in plants) or by isochorismate mutase [Citation284]. In the alternative pathway, phenylalanine ammonia lyase (PAL) converts the chorismate-derived phenylalanine to trans-cinnamic acid, which is then decarboxylated to benzoic acid ((B)). Benzoic acid is subsequently hydroxylated to SA by benzoic acid 2-hydroxylase (BA2H) [Citation285–Citation288]. However, several gaps in the SA biosynthesis pathway have yet to be determined. Although the ICS pathway is confirmed to account for over 95% of SA production [Citation289], many studies support the importance of PAL in pathogen-induced SA accumulation [Citation290,291]. Meuwly et al. [Citation290,292] and Pallas et al. [Citation290] demonstrated that treatment with 2-aminoindan-2-phosphonic acid (AIP, 135; (A)), a PAL inhibitor, suppressed pathogen-induced SA accumulation in tobacco, cucumber, and Arabidopsis. Further experiments supported the notion that PAL is a key factor in SA biosynthesis, which is in contrast to findings of Garcion et al. [Citation289]. Although it is well known that the conversion of isochorismate to SA in bacteria is catalyzed by ICS and IPL, the details of this process in plants remain unclear; no IPL-like protein has been identified in plants. Chemicals that specifically target biosynthetic and metabolic enzymes could be useful for addressing these unknowns. To date, only a few regulators in SA biosynthesis have been identified and utilized, such as AIP that targets PAL (as mentioned above).
SA can be further modified by methylation, glucosylation, sulfonation, hydroxylation or conjugation to l-aspartic acid (reviewed in Dempsey et al. [Citation284]). These processes are important for SA bioactivity and homeostasis and could also be used as targets for developing chemical regulators. For example, methyl salicylate (MeSA, 136; (A)) is a volatile SA that is involved in long-distance plant defense, also known as the SAR response. SA can be converted to biologically inactive MeSA by SA methyltransferase [Citation293]. MeSA can be converted back to biologically active SA by SA-binding protein 2 (SABP2), a methylesterase [Citation294]. These conversions of SA between its free and methylated forms control the local and distant SA levels. In fact, SA was demonstrated to be an effective inhibitor of SABP2 [Citation295], which is considered to control SA homeostasis in a feedback manner. Antagonistic activity of SA on SABP2 in planta cannot be studied because SA triggers bioactivity downstream of SABP2. 2′-Methoxy-2,2,2-triFA (methoxy-triFA, 137), 2,2,2,2′-tetraFA (tetraFA, 138), and 2,2,2-triFA (triFA, 139), which have structures similar to that of SA, were shown to compete with MeSA for binding to SABP2 ((A) and (B)), despite their activity being weaker than that of SA [Citation295]. TetraFA was determined to be a good candidate that specifically inactivates the esterase activity of SABP2 in vitro and blocks SAR responses in TMV-infected tobacco in vivo.
The SA signaling pathway has been widely studied. Non-expresser of pathogenesis-related genes 1 (NPR1) is a master regulator and resides in the cytoplasm in an oligomeric form. Pathogen invasion induces SA accumulation and changes the redox environment, which triggers depolymerization of polymerized NPR1 to the monomer. Then, NPR1 monomer translocates to the nucleus and interacts with the TGA transcription factors, which induce the transcription of pathogenesis-related (PR) genes [Citation296,297] ((B)). A recent report has shown that the NPR1 paralogs NPR3 and NPR4 are SA receptors that target NPR1 for degradation in an SA concentration-dependent manner [Citation298]. Simultaneously, Wu et al. [Citation299] demonstrated that NPR1 could bind to SA and function as an SA receptor ((B)). Synthetic ligands (agonist or antagonist) with selectivity for specific receptors can be expected to provide further insight on this debated pathway.
Numerous chemicals that induce SAR responses through SA signaling have been identified, although for some, the modes of action and targets have not been well defined. Benzo(1,2,3)-thiadiazole-7-carbothioic acid S-methyl ester (BTH, 140) is a synthetic chemical that induces disease resistance without causing SA accumulation [Citation300–Citation302]. 3-Allyloxy-1,2-benzisothiazole 1,1-dioxide (probenazole, 141) and its derivative 1,2-benzisothiazol-3 (2H)-one 1,1-dioxide (BIT, 142) were reported to be defense activators upstream of SA accumulation [Citation303,304]. BTH and 2,6-dichloroisonicotinic acid (INA, 143) are used as SA analogs to induce SAR responses in plants [Citation305–Citation308]. Yasuda et al. [Citation309] reported that N-cyanomethyl-2-chloroisonicotinamide (NCI, 144) induces disease resistance in an NPR1-dependent manner, without SA accumulation, indicating that NCI functions between SA and NPR1 in an SA signal transduction pathway. Further investigation of their targets and the functional mechanism is expected to unravel SA- and SA-induced SAR responses. To identify chemical regulators downstream of SA accumulation, Seo et al. [Citation310] designed a chemical screening by using a transgenic Arabidopsis line with the β-glucuronidase (GUS) gene as reporter driven by the PR1 promoter (PR1:GUS). Several chemicals that suppressed exogenous SA-induced GUS expression were identified, and PAMD (145) was found to display the strongest inhibitory activity, despite having adverse effects on plant growth. A follow-up study addressing the structure-activity relationship of PAMD derivatives developed a better SA signaling inhibitor, compound 4 (146), which has fewer side effects on plant growth [Citation311]. To date, the target of PAMD and its derivative remain elusive. However, PAMD and its derivative did not inhibit the interaction between NPR1 and TGA in a yeast-two-hybrid assay (Jiang et al. unpublished). Investigation of the chemical targets could broaden our knowledge of the SA signaling pathway. Using the screening system established by Seo et al. [Citation310], another chemical (with a structure different from that of PAMD) that has a stronger inhibitory activity on the expression of PR genes and no obvious side effects on plant growth was identified (Jiang et al. unpublished). These SA signal inhibitors can now be used in the study and control of SA signaling.
Priming plants with activators can protect them from pathogens by inducing the intrinsic immunity of plants. Some SA regulators have been identified from screenings for priming chemicals. Nouthoshi et al. conducted a chemical screening for plant immune activators and identified several series of chemicals with different modes of action, including imprimatin A and B (147, 148, 149 and 150), which increase the endogenous SA level and reduce its metabolite SA-O-β-D-glucoside by inhibiting SA glucosyltransferases [Citation312,313], imprimatin C (151 and 152), which acts as a weak SA analog [Citation314], diuretics (bumetanide 153, bendroflumethiazide 154, and clopamide 155), and sulfonamides (sulfameter 156, sulfamethoxypyridazine 157, sulfabenzamide 158, and sulfachloropyridazine 159), which function in a SA-independent pathway as they do not induce defense-related genes [Citation315,316] ((A) and (B)). With the completion of more screenings and the characterization of chemicals related to SA, our knowledge about SA biosynthesis, metabolism, and signaling pathway is expected to broaden.
Strigolactones
Strigolactones (SLs) are a family of sesquiterpene lactones derived from carotenoid. Due to their low abundance in plants and unstable characteristics, SLs were not identified as a plant hormone until the introduction of highly sensitive mass spectrometry. SL was first isolated from the root exudates of cotton in 1966 and was shown to act as a germination stimulant for the root parasite, witchweed (Striga lutea Lour.) [Citation317]. During the following decades, SLs were merely considered detrimental signal molecules that are secreted by autotrophic plants, leading to their hijack by parasitic plants. Akiyama et al. [Citation318] broadened our view on the functions of SL by demonstrating that 5-deoxystrigol (5DS, 160; (A)), a natural stereoisomer of SLs (another is 4-deoxyorobanchol, 4DO, 161; (A)), acts as a branching factor to promote the hyphal branching of arbuscular mycorrhizal (AM) fungi, which in turn help plants to capture nutrients. SLs were finally recognized as plant hormones following the finding that SL inhibits shoot branching in rice and Arabidopsis [Citation319,320]. In addition, studies have indicated that SLs also play roles in enhancing lateral root formation and root hair elongation [Citation321], inhibiting adventitious root formation [Citation322], stimulating cambial activity [Citation323], accelerating leaf senescence [Citation324], counteracting temperature-induced seed dormancy [Citation325], and playing a synergistic role in sugar-induced suppression of seedling establishment [Citation326]. Application of SLs can be expected to contribute to agriculture. For example, in Africa, the Mediterranean area, and the Middle East, crops are often severely damaged by Striga and Orobanche, parasitic weeds that deprive their hosts of nutrition. These weeds are difficult to control because of the large quantity and small size of their seeds. SL- or chemical-induced germination of Striga and Orobanche in the absence of hosts will lead to their death due to the absence of a nutrient provider. This approach, which is also referred to as suicide germination, could be an effective approach for controlling [Citation327] damage by these parasitic weeds. SLs are chemical communicators for AM fungi and signaling molecules for plants. Moreover, SLs can be used to improve nutrient absorption and to regulate plant stature [Citation328,329].
Figure 9. Chemical structures of strigolactone-related chemicals and simplified schematic model of strigolactone biosynthesis and signal transduction. A detailed description of the processes is described in the section on strigolactone. The numbers in bold correspond with the numbers in (A) and they are unique call numbers in this paper.
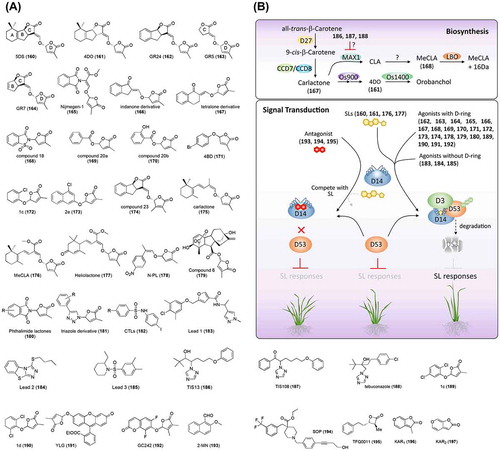
Following the initial isolation of SL from cotton, many natural SLs were identified from various plants [Citation330]. Although the structures of these natural SLs display diversity in their tricyclic lactone (ABC-ring), they all possess a butenolide ring (D-ring) linked by an enol ether bridge [Citation99], which is used as a criterion when designing SL analogs (as discussed later). The complicated structures and easy decomposition of natural SLs limit their application. Johnson et al. [Citation331] developed a series of synthetic analogs of strigol, including GR5 (162) with a single ring, GR7 (163) with a bicyclic ring, and GR24 (164) with a tricyclic ring connected with a butenolide ring by enol ether linkage ((A)). All of these analogs promote the germination of Striga and Orobanche spp. seeds, although GR5 and GR7 show relatively lower activity. GR24 has a structure similar to that of natural SL and shows strong bioactivity, and has therefore been used as an effective alternative to natural SL. These results further indicated that the CD ring, but not the AB-ring, is essential for SL-like activity. Interestingly, a later study confirmed that two GR24 diastereomers display a different level of bioactivity, indicating that the stereochemistry also affects the bioactivity of SL analogs [Citation332,333]. Based on this concept, Zwanenburg and colleagues developed several series of SL analogs, including Nijmegen-1 (165), a phthaloylglycine-derived strigol analog [Citation334] and its derivatives [Citation335], as well as indanone- (166) and teralone (167)-derived analogs ((A)) [Citation336]. It is noteworthy that compound 18 (168), derived from saccharin by direct coupling with the D-ring, and the compounds 20a (169) and 20b (170), derived from benzoic acid and SA connected to the D-ring by an ester linkage ((A)), display an unexpectedly high activity for Orobanche cernua seeds, although they do not possess an enol ether bridge [Citation337], which hints at a new approach for designing germination stimulants. Simultaneously, to develop novel SL mimics with simple structures, Fukui et al. [Citation338] designed a series of phenoxy furanone derivatives lacking the enol ether moiety and confirmed that they show inhibitory effects on tiller bud outgrowth in rice at levels similar to or stronger than that of GR24, and named these as debranones (derives from debranching furanone). SAR studies characterized 4-Br debranone (4BD, 171) as the most potent SL mimic. 4BD was confirmed to show SL-like activities, including inhibition of shoot branching in Arabidopsis, promotion of root hair elongation, and suppression of lateral root formation, albeit with weaker effectivity than GR24 [Citation339]. Unexpectedly, 4BD displayed no SL-like activity in inducing seed germination of Striga at a normal range of chemical treatment (10−9–10−6 M), despite its potent activity (stronger than that of GR24) in suppressing tiller outgrowth in rice [Citation338,339], indicating a possibility that synthetic chemicals such as debranones could be developed with selectivity for specific species, according to the purpose of application. Thus, the authors proposed a strategy to protect crops from the threats of Striga and Orobanche by a combination of SL mutant plants with 4BD [Citation339]. A further SAR study on debranones (2nd generation debranone) by altering the substituent position on the phenyl ring or replacing the phenyl ring with polycyclic moieties enabled changes in selectivity of debranone derivatives for plant species (at least between rice and Striga) [Citation340]. For example, 1c (172) (with an altered substituent position) and 2e (173, with a bicyclic moiety introduced) ((A)) have SL-like activities in both rice and Striga [Citation340]. Upon the SAR studies on GR24, Boyer et al. [Citation341] found that the presence of a Michael acceptor and a methylbutenolide or dimethylbutenolide motif in the same molecule is necessary for SL perception by D14. It is noteworthy that compound 23 (174), with a 3,4-dimethylbutenolide D-ring ((A)), is the most active SL analog for bud outgrowth repression, although 23 and GR24 differ only in the presence of a methyl group at the 4-position of the D-ring. These data suggest that artificial chemicals not only mimic the activities of natural SLs but also display some unique characteristics, such as plant species selectivity, which could be exploited for both agricultural and research applications. Recently, Alder et al. [Citation342] identified carlactone (CL, 175; (A)) as a precursor of bioactive SL. CL treatment suppressed tiller outgrowth in rice and induced Striga seed germination even though CL lacks the enone moiety of the C-ring that is present in both 5DS and 4DO. Later studies confirmed that methyl carlactonate (MeCLA, 176; (A)) (a product of CL) and its metabolite (MeCLA + 16 Da, which is catalyzed by lateral branching oxidoreductase [LBO]) show SL activity, inhibiting shoot branching in Arabidopsis [Citation343,344]. A recent study identified heliolactone (177) from sunflower as a non-sesquiterpene lactone with a CL scaffold that induces seed germination in Striga and Orobanche [Citation345]. Thus, CL-type chemicals lacking the enone moiety of the C-ring have been proposed as a subgroup of SLs [Citation100]. Based on these findings, Jia et al. [Citation346] designed a new chemical, nitro-phenlactone (N-PL, 178; (A)), and found that N-PL has SL activities, including promotion of the germination of Striga seeds and suppression of tiller outgrowth in rice and rosette branching in Arabidopsis, but that it does not induce hyphal branching in AM fungi. It is worth noting that more axillary growth1 (MAX1) has additive effects on N-PL activity in Arabidopsis, which indicates that N-PL might be subjected to further oxidation by MAX1, leading to a more active product. Various other scaffolds, such as acyclic carbon chain [Citation347], GA3 (compound 6, 179) [Citation348], phthalimides (180) [Citation349], and triazole (181) [Citation350], have been recruited and coupled with a butenolide ring for the development of SL mimics ((A)). Chemical genetic screening using yeast and Arabidopsis led to the identification of SL-related cotylimides (CTLs, 182; (A)) that can induce suicidal germination [Citation351,352]. CTLs have been confirmed to perturb SL levels, and thus, are assumed to target the SL biosynthesis pathway [Citation351]. A later study showed that CTLs promote the interaction of HTL/KAI2, a homolog of SL receptor D14, with MAX2, a key regulator of SL signaling [Citation352], indicating that CTLs might also function during SL signaling (in an unknown manner). Interestingly, similar to GR24, the lead chemicals (183, 184, and 185; (A)) identified by Toh et al. [Citation352] inhibit hypocotyl growth in Arabidopsis and induce seed germination in Striga, despite being structurally different from the other identified SL analogs. This was the first report of SL mimics lacking the normally conserved D-ring, suggesting that that chemicals inducing suicidal germination might be developed beyond the typical D-ring-containing SL analogs.
The SL biosynthesis pathway has been well studied, including the characterization of several mutants (dwarf mutants in rice and max mutants in Arabidopsis) combined with the synthetic SL analog GR24 (reviewed in Xie et al. [Citation99] and Al-Babili and Bouwmeester [Citation100]). Briefly, SL is derived from β-carotenoid, which undergoes a sequential catalysis by several enzymes to produce the final active SLs, including D27 that catalyzes isomerization of 9-cis/all-trans-β-carotene to 9-cis-β-carotene [Citation342,353,354], carotenoid cleavage dioxygenase 7 (CCD7) and CCD8 that sequentially cleavage 9-cis-β-carotene into CL [Citation342], and CYP711s that oxidize CL to CLA (converted by MAX1) in Arabidopsis [Citation343], or to 4-deoxyorobanchol (converted by Os01g0700900) and orobanchol (converted by Os01g0701400) in rice [Citation355] ((B)). To date, chemical inhibition of endogenous SL by targeting these biosynthesis enzymes has been useful for investigating SL biosynthesis and extending our knowledge of the biological roles of SLs in plants [Citation356]. Several chemicals have been reported to inhibit SL biosynthesis, including abamine (60, (A)) targeting CCD [Citation357], TIS13 (186, (A)) and its derivative TIS108 (187, (A)), which might target P450 [Citation358–Citation360], and tebuconazole (188, (A)) targeting P450 [Citation361], although not all of these are specific inhibitors of SL biosynthesis. Sergeant et al. [Citation362] developed a series of new inhibitors of CCD based on abamine and found that several of these could inhibit CCDs and promotion of branching, suggesting that these chemicals could be SL biosynthesis inhibitors. All of these chemicals are potential leads for developing SL biosynthesis inhibitors (discussed in detail in Nakamura and Asami [Citation356]). Recently, GA was identified as a novel SL biosynthesis inhibitor as it suppresses the expression of SL biosynthesis genes (D27, D17, D10, Os01g0700900, and Os01g0701400) in rice, although the mechanism remains unknown [Citation363]. In accordance herewith, AC94377 (42, (A)), as a GA mimic, also displays inhibitory effects on SL biosynthesis in the same manner as GA does (Ito et al. unpublished). These results not only suggest a crosstalk between SL and GA but also provide a novel way to regulate SL levels in plants.
Studies investigating the SL-signaling pathway have made fast progress. DWARF 14 (D14), a member of the α/β hydrolase superfamily, was first identified as a tiller regulator in rice [Citation364–Citation366]. Computational homology modeling suggested that D14 might be an SL receptor because of its similarity to several α/β hydrolase-like proteins, such as the GA receptor GID1, although it conserves the catalytic triad [Citation367]. This hypothesis was later confirmed by the crystal structure and biochemical studies of DAD2, an ortholog of rice D14 in petunia [Citation368], and by subsequent reports of rice D14 [Citation369–Citation371] and AtD14 [Citation371]. It is demonstrated that GR24 binds to DAD2/D14/AtD14, followed by its hydrolysis. Zhao et al. [Citation371] and Nakamura et al. [Citation369] attempted to co-crystallize a complex of GR24 and D14 and obtained an intermediate of the GR24 degradation product and a final degradation product butenolide D-ring at the entrance of the pocket, which supports the notion that the D-ring is hydrolyzed by nucleophile attack, as proposed by Zwanenburg et al. [Citation336]. In accordance herewith, a mutation in the catalytic triad impaired hydrolysis of GR24 by DAD2 or D14 [Citation368,369]. Crystallization of the D14-GR24 complex showed that the D-ring is close to the catalytic triad and is ready to be attacked by the serine residue [Citation372]. These results, together with SAR studies on GR24 and other SL mimics mentioned above, suggest that SL perception is accompanied by an enzymatic hydrolysis process, which is different from the perception mechanisms of other plant hormones. Two groups simultaneously reported that DWARF 53 (D53) could be a downstream repressor of SL signaling in regulating branching in rice [Citation373,374]. Genetic and biochemical studies have indicated that D53 can interact with the SL receptor D14 and DWARF 3 (D3) (an F-box protein of the SCF E3 ubiquitin ligase complex) in a GR24-dependent manner, which leads to the ubiquitination and degradation of D53. Based on these findings, a model of the SL signaling pathway was established, as shown in (B). SL perception by D14 leads to the ubiquitination and degradation of D53 by the proteasome, which triggers the SL signal; however, the details remain unclear [Citation373,374]. A similar SL signaling pathway perceived and transduced by AtD14, MAX2, and SMAX1/SMXL, sequentially, has been confirmed in Arabidopsis [Citation375,376]. ShMAX2 and SL receptors (ShHTLs/ShKAIs) have also been identified in Striga, despite the fact that no homolog of D53/SMAX1/SMXL has been found in Striga [Citation352,377,378]. Up to 2015, although the key factors in SL signaling have been identified and many crystallographic structures of SL receptors have been obtained, there was continued debate about how SL is perceived by D14 and induces signaling. Recent success in solving the crystal structure of the SL-induced AtD14-D3-ASK1 complex demonstrated that D14 binds to SL and hydrolyzes SL into a covalently linked intermediate molecule, which initiates the conformational change of AtD14 and facilitates its interaction with D3 [Citation379].
These findings regarding the SL signaling pathway help with the design of novel PGRs and inducers of suicidal germination. Thus far, SL receptors are the main targets for developing SL signal regulators. Fukui et al. [Citation380] did a docking simulation of 4BD to design agonists of OsD14 [Citation372] and ShHTL5 [Citation352]. A series of chemicals (3rd generation debranone) with two substituents on the phenyl ring, such as 1c and 1d (189 and 190, (A)), have been synthesized and confirmed to more strongly promote Striga seed germination than 4BD [Citation339]. Tsuchiya et al. [Citation381] designed a fluorescence turn-on probe, Yoshimulactone Green (YLG, 191; (A)), which conserves the butenolide D-ring. YLG displays SL activity and releases a fluorescent molecule after hydrolysis by SL receptors. Dynamic SL perception by Striga seeds has been confirmed by using this tool. Germain et al. [Citation382] synthesized another series of profluorescent molecular probes by coupling the butenolide D-ring to a 4-methylcoumarin (DiFMU) fluorophore (GC242, 192; (A)), whose hydrolysis can be quantified by observing the fluorescence emitted from free DiFMU. These fluorescent reporters are expected to extend existing methodologies in SL signal studies, including evaluation of the enzyme kinetics of SL receptors and chemical affinity for receptors [Citation381–Citation384].
Recently, several SL antagonists have been identified by chemical screening. In an attempt to identify its antagonist, Mashita et al. [Citation385] conducted an in silico screening based on the D14 structure. Eventually, 2-MN (193, (A)) was identified and demonstrated to be an effective antagonist that inhibits GR24-induced interactions of D14 with D53 and SLR1 and recovers tiller growth inhibited by GR24. GR24-induced germination of Striga seeds can be suppressed by co-treatment with 2-MN, suggesting that 2-MN is a broad-spectrum antagonist. A chemical screening in Arabidopsis identified soporidine (SOP, 194; (A)), which inhibits the activity of ShHTL7, an SL receptor in Striga [Citation386], and suppresses Striga germination without inducing obvious phenotypical changes in rice [Citation383]. Intriguingly, Xiang et al. [Citation384] developed multiple β-lactones, such as TFQ0011 (195, (A)), which introduces a covalent modification of SL receptors and displays an irreversible antagonistic activity against GR24 for AtD14 and ShHTL7. This irreversible inhibition of the SL receptor by β-lactone suggests that, similar to 4BD derivatives, inhibitors with selectivity for specific targets can be developed through SAR studies of the phenyl moiety. In fact, the chemical covalent modification of proteins has been widely used for the development of irreversible inhibitors and activity-based protein profiling. This modification targets the potential nucleophilic residues, such as serine, cysteine, lysine, tyrosine, threonine, aspartate, and glutamate [Citation387]. Several series of chemotypes have been developed to target serine nucleophiles, to which SL receptors belong. In addition to the β-lactone, fluorophosphates, diphenyl phosphonates, coumarins, carbamates, and heterocyclic ureas also covalently modify serine and thus, are potential moieties for developing SL receptor inhibitors.
Following the identification of SL as a plant hormone, during the last decade, great progress has been made in identifying natural SLs, investigating SL biosynthesis and signal transduction, and developing SL analogs and mimics. However, many questions remain to be answered, including: How is CL converted to 5-deoxystrigolactone? In addition to MeCLA and MeCLA + 16, are there any unknown SLs with a tricyclic ABC-ring in Arabidopsis? Are other factors involved in SL biosynthesis in rice, Arabidopsis, and other plants? How are non-canonical SL mimics (that do not have the D-ring) recognized by SL receptors? How does D14 integrate D3/MAX2 with D53/ SMXLs? In addition to D53/ SMXLs and D3/MAX2, D14 can also interact with SLR1; what is the physiological importance of this? What are the mechanisms of SL perception and transduction in AM fungi? To answer these questions, SL biosynthesis inhibitors and specific agonists and antagonists are expected to play critical roles together with genetic and biochemical analyses. For example, for assessing the physiological importance of the D14–SLR1 interaction, chemicals that can discriminately promote the interaction of D14 with D53 or SLR1 will provide further insight.
Last but not least, karrikins (KARs, 196 and 197; (A)), a family of butenolides derived from smoke of burning plant materials, play important roles in seed germination and plant growth (reviewed by Waters et al. [Citation388]; Smith and Li [Citation389]; De Cuyper et al. [Citation390]). KARs are perceived by karrikin insensitive 2 (KAI2), a paralog of D14. KARs and SLs display complicated crosstalk by sharing MAX2 but having different targets (SMAX1 for KAI2 and SMXL6/7/8 for D14) [Citation376,391,392]. Although KARs have been confirmed as ligands of KAI2, the natural KAI2 ligand has not yet been identified [Citation393]. Thus, information about the biosynthesis this ligand in plants and its inducing signal is scarce. Progress in these issues can be expected by integrating traditional genetic and biochemical studies with chemical genetics.
Achievements and prospects of chemical genetics
Chemical genetics is a powerful tool in biology studies that utilizes small molecules screened from chemical libraries to decipher biological processes. Compared to conventional genetics approaches, chemical genetics has several important advantages, including that it can overcome the lethality of certain mutations and gene redundancy [Citation394–Citation396]. In the above sections, we summarized numerous chemical regulators that were screened from chemical libraries or were rationally designed (Figures , and ). These chemicals perturb biosynthesis, transport, perception, and signal transduction of the major plant hormones and have greatly contributed to plant hormone studies. These chemicals are useful tools and might also be lead chemicals for the development of additional PGRs. Further screening for novel chemicals will be needed to broaden our understanding of plant hormones and to develop desirable PGRs.
Figure 10. Schematic representation of the workflow of chemical screening (adapted from Dejonghe and Russinova396)) and its applicable fields.
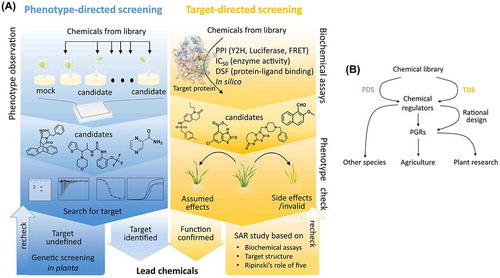
Table 1. Chemicals and their call numbers in this manuscript.
There are two common strategies for chemical screening in plants: phenotype-directed screening (PDS) and target-directed screening (TDS) ((A)). By observing phenotype changes in plants, PDS can identify novel chemicals with different structural scaffolds and diverse modes of action. For example, seed germination-based screening identified pyrabactin (73; (A)), an ABA agonist [Citation1], and 67D (43; (A)), a GA mimic [Citation63]. However, the modes of action of candidate chemicals are always as expected. For example, screening based on seed germination identified CTLs (182; (A)), which are related to SL signaling [Citation351], and germostatin (31; (A)), which targets a factor (GSR1) in auxin signaling [Citation38], rather than chemicals related to ABA or GA signals, which are considered the most closely related to seed germination. Thus, the design for PDS approaches should be as specific as possible and should be reevaluated by multiple systems. Nevertheless, PDS approaches can be effective at identifying novel candidates with novel action mechanisms and structures.
TDS is a classic reverse screening approach for drug discovery that focuses on a specific target, such as a receptor or enzyme. This methodology is based on a good understanding of the modes of action of the targets. Thus, TDS can serve as a high-throughput tool to identify regulators for specific targets. TDS approaches have been successfully applied in plants. For example, by targeting ABA receptors and their interactions with PP2C, Okamoto et al. [Citation132] and Cao et al. [Citation133] identified the same chemical, quinabactin/AM1 (74; (A)), as an agonist of PYLs. Similarly, Yoon et al. [Citation64] identified TSPC (45; (A)) as an antagonist of GID1 by targeting the GID1 receptor and its interaction with DELLA. Because of advances in X-ray crystallography and computer science, 3-dimensional (3D) structures of various receptors and enzymes have been experimentally determined or predicted. In-silico screening has been used to identify several chemicals with important roles in plants, such as an antagonist of the SL receptor (2-MN, 193; (A)) [Citation385], non-steroid BRI1 antagonists (117, 118; (A)) [Citation223], a steroid BRI1 agonist (NSBR1, 119; (A)) [Citation224], and UNI (35; (A)), a known P450 inhibitor rediscovered as a CK biosynthesis inhibitor [Citation74]. TDS approaches also have some disadvantages. For example, bioavailability is not guaranteed, and off-target effects can occur when these chemicals are applied to plants. The use of a stricter assay system can help to address these issues. A further rational modification of lead chemicals can also be expected to improve candidate bioavailability and specificity.
In addition to general library screening, the rational design of chemicals based on the 3D structure of targets has been used to developed chemicals with target specificity, such as BH-IAA, PEO-IAA, and auxinole (28, 29, and 30, respectively; (A)) as auxin receptor antagonists [Citation36,37], AS6 (75; (A)) as an ABA receptor antagonist [Citation138], jarin-1 (127; (A)) as a JA biosynthesis inhibitor, and 4BD derivatives (189 and 190; (A)) with species selectivity [Citation380]. Recently, the development of cryo-electron microscopy (cryo-EM) has provided an additional technique (in addition to X-ray and NMR) for resolving macromolecular structures. Because of advances in instrument development and the contributions made by Richard Henderson, Joachim Frank, and Jacques Dubochet (who shared the Nobel Prize in 2017) [Citation397], cryo-EM has allowed resolving a great number of protein structures, including those of membrane proteins and protein–protein and protein–drug complexes (reviewed in Wang et al. [Citation398], Jonic [Citation399], and Merino and Raunser [Citation400]). Because there is no need for crystallization, cryo-EM overcomes several of the limitations associated with X-ray and NMR analyses, including limitations in molecular size, stability, and yield [Citation400]. Thus, more protein structures, including membrane proteins (e.g. the full-length ET receptors, CK receptors, and enzymes belonging to CYP family) and high-molecular-weight complexes, are expected to be dissolved by cryo-EM, thus providing more information for the rational design of chemical regulators.
A recent report by Park et al. [Citation144] presents an intriguing application of chemical regulators. Based on an orthogonal ligand-receptor strategy (reviewed by Chockalingam, Zhao [Citation401]; Kavita [Citation402]; Assaf et al. [Citation403]), mandipropamid (80; (A)), an agrochemical, was found to trigger ABA-mediated drought stress responses in plants via an engineered ABA receptor, PYR1 (PYR1MANDI), which carries amino-acid substitutions in key residues of the pocket of PYR1MANDI, which results in its selective binding to mandipropamid but not the natural ligand ABA [Citation144]. Thus, when treated with mandipropamid, PYR1MANDI-expressing transgenic plants show increased drought resistance, without affecting the endogenous ABA. Because of the recent progress in deciphering the 3D structures of plant-hormone receptors (reviewed by Miyakawa and Tanokura [Citation404]), this orthogonal ligand–receptor strategy can now be applied to other plant hormones. For example, a recent report found that a phthalimide derivative, AC94377, acts as an agonist of the GA receptor [Citation61]. Based on docking simulation results, rational modification on AC94377 yielded novel derivatives (ACDs) that lose their GA-like activities in vivo and in vitro (Jiang et al. unpublished). Further engineering of GID1 by modifying key amino-acid residues decreased its affinity for GA and increased its responses to ACDs that are inactive for wild-type GID1 (Jiang et al. unpublished). These findings indicate that the combination of engineered GID1 and ACDs could be used to manipulate plant growth and development. Moreover, such manipulation could be spatiotemporal if engineered GID1 is driven by tissue-specific or chemical-inducible promoters.
Lastly, several plant hormones have been shown to have bioactivity in other species (e.g. auxin has been implicated in cancer [Citation405–Citation407], SA and its derivatives have an anti-inflammatory effects in humans [Citation408], ABA is included in nutraceuticals for glycemic control [Citation409,410], JA and its derivatives promote apoptosis of human cancer cells [Citation411–Citation413], and SL analogs have anti-cancer activity [Citation414,415] and induce hyphal branching in AM fungi [Citation318]). It is reasonable that those PGRs targeting proteins with functional and structural conservation across species might also act as regulators in multiple species.
Conclusion
In this review, we summarized chemical regulators of plant hormone signaling and their roles in deciphering the plant hormone network, which suggests the importance and prospects of these regulators in plant hormone studies and PGR development. The biosynthesis, metabolism, transport, and signal perception and transduction of plant hormones involve a diverse range of receptors and enzymes, providing an extensive number of regulatory points for chemical control. In combination with traditional genetics, structural biology, and bioinformatics, PDS and TDS—together with rational design—are expected to contribute to deciphering the complexity of the plant hormone network and the development of specific PGRs ((A) and (B)).
Funding
This work was supported by a grant from the Core Research for Evolutional Science and Technology (CREST) Program of Japan Science and Technology Agency (JST), and by a grant from the Deanship of Scientific Research (DSR) at King Abdulaziz University, Jeddah, Saudi Arabia, [grant number 1-130-36-HiCi]. The authors thank the DSR for technical and financial support.
Disclosure statement
No potential conflict of interest was reported by the authors.
Acknowledgements
We apologize that, because of space limitations and time lag during the submission process, we were unable to cite many other excellent and recent works. This work was supported by a grant from the Core Research for Evolutional Science and Technology (CREST) Program of Japan Science and Technology Agency (JST), and by a grant from the Deanship of Scientific Research (DSR) at King Abdulaziz University, Jeddah, Saudi Arabia, under grant number 1-130-36-HiCi. The authors thank the DSR for technical and financial support.
Notes
* This review was written in response to the author’s receipt of the JSBBA Award in 2017.
References
- Park S-Y, Fung P, Nishimura N, et al. Abscisic acid inhibits type 2C protein phosphatases via the PYR/PYL family of START proteins. Science. 2009;324:1068–1071.
- Zhao Y. Auxin biosynthesis and its role in plant development. Annu Rev Plant Biol. 2010;61:49–64.10.1146/annurev-arplant-042809-112308
- Korasick DA, Enders TA, Strader LC. Auxin biosynthesis and storage forms. J Exp Bot. 2013;64:2541–2555.10.1093/jxb/ert080
- Kasahara H. Current aspects of auxin biosynthesis in plants. Biosci Biotechnol Biochem. 2015;80:1–9.10.1080/09168451.2015.1086259
- Soeno K, Goda H, Ishii T, et al. Auxin biosynthesis inhibitors, identified by a genomics-based approach, provide insights into auxin biosynthesis. Plant Cell Physiol. 2010;51:524–536.10.1093/pcp/pcq032
- Adams DO, Yang SF. Ethylene biosynthesis: identification of 1-aminocyclopropane-1-carboxylic acid as an intermediate in the conversion of methionine to ethylene. Proc Natl Acad Sci U S A. 1979;76:170–174.10.1073/pnas.76.1.170
- Narukawa-Nara M, Nakamura A, Kikuzato K, et al. Aminooxy-naphthylpropionic acid and its derivatives are inhibitors of auxin biosynthesis targeting l-tryptophan aminotransferase: structure-activity relationships. Plant J. 2016;87:245–257.10.1111/tpj.2016.87.issue-3
- Kakei Y, Nakamura A, Yamamoto M, et al. Biochemical and chemical biology study of rice OsTAR1 revealed that tryptophan aminotransferase is involved in auxin biosynthesis; identification of a potent OsTAR1 inhibitor, pyruvamine2031. Plant Cell Physiol. 2017;58:598–606.
- He W, Brumos J, Li H, et al. A small-molecule screen identifies l-kynurenine as a competitive inhibitor of TAA1/TAR activity in ethylene-directed auxin biosynthesis and root growth in arabidopsis. Plant Cell. 2011;23:3944–3960.10.1105/tpc.111.089029
- Nishimura T, Hayashi K, Suzuki H, et al. Yucasin is a potent inhibitor of YUCCA, a key enzyme in auxin biosynthesis. Plant J. 2014;77:352–366.10.1111/tpj.2014.77.issue-3
- Kakei Y, Yamazaki C, Suzuki M, et al. Small-molecule auxin inhibitors that target YUCCA are powerful tools for studying auxin function. Plant J. 2015;84:827–837.10.1111/tpj.13032
- Grones P, Friml J. Auxin transporters and binding proteins at a glance. J Cell Sci. 2015;128:1–7.10.1242/jcs.159418
- Petrásek J, Friml J. Auxin transport routes in plant development. Development. 2009;136:2675–2688.10.1242/dev.030353
- Zazimalova E, Murphy AS, Yang H, et al. Auxin transporters–why wo many? Cold Spring Harb Perspect Biol. 2010;2:a001552.
- Terasaka K. PGP4, an ATP binding cassette P-glycoprotein, catalyzes auxin transport in arabidopsis thaliana roots. Plant cell. 2005;17:2922–2939.10.1105/tpc.105.035816
- Geldner N, Friml J, Stierhof Y-D, et al. Auxin transport inhibitors block PIN1 cycling and vesicle trafficking. Nature. 2001;413:425–428.10.1038/35096571
- Noh B, Murphy AS, Spalding EP. Multidrug resistance-like genes of arabidopsis required for auxin transport and auxin-mediated development. Plant Cell. 2001;13:2441–2454.10.1105/tpc.13.11.2441
- Geisler M, Blakeslee JJ, Bouchard R, et al. Cellular efflux of auxin catalyzed by the arabidopsis MDR/PGP transporter AtPGP1. Plant J. 2005;44:179–194.10.1111/j.1365-313X.2005.02519.x
- Shin H, Shin H-S, Guo Z, et al. Complex regulation of Arabidopsis AGR1/PIN2-mediated root gravitropic response and basipetal auxin transport by cantharidin-sensitive protein phosphatases. Plant J. 2005;42:188–200.10.1111/tpj.2005.42.issue-2
- Ruegger M, Dewey E, Hobbie L, et al. Reduced naphthylphthalamic acid binding in the tir3 mutant of Arabidopsis is associated with a reduction in polar auxin transport and diverse morphological defects. Plant Cell. 1997;9:745–757.10.1105/tpc.9.5.745
- Ruegger M, Dewey E, Gray WM, et al. The TIR1 protein of Arabidopsis functions in auxin response and is related to human SKP2 and yeast Grr1p. Genes Dev. 1998;12:198–207.10.1101/gad.12.2.198
- Dharmasiri N, Dharmasiri S, Estelle M. The F-box protein TIR1 is an auxin receptor. Nature. 2005;435:441–445.10.1038/nature03543
- Kepinski S, Leyser O. The Arabidopsis F-box protein TIR1 is an auxin receptor. Nature. 2005;435:446–451.10.1038/nature03542
- Surpin M, Rojas-Pierce M, Carter C, et al. The power of chemical genomics to study the link between endomembrane system components and the gravitropic response. Proc Natl Acad Sci U S A. 2005;102:4902–4907.10.1073/pnas.0500222102
- Rojas-Pierce M, Titapiwatanakun B, Sohn EJ, et al. Arabidopsis P-glycoprotein19 participates in the inhibition of gravitropism by gravacin. Chem Biol. 2007;14:1366–1376.10.1016/j.chembiol.2007.10.014
- Kim J-Y, Henrichs S, Bailly A, et al. Identification of an ABCB/P-glycoprotein-specific inhibitor of auxin transport by chemical genomics. J Biol Chem. 2010;285:23309–23317.10.1074/jbc.M110.105981
- Tsuda E, Yang H, Nishimura T, et al. Alkoxy-auxins Are selective inhibitors of auxin transport mediated by PIN, ABCB, and AUX1 transporters. J Biol Chem. 2011;286:2354–2364.10.1074/jbc.M110.171165
- Ueda J, Toda Y, Kato K, et al. Identification of dehydrocostus lactone and 4-hydroxy-β-thujone as auxin polar transport inhibitors. Acta Physiol Plant. 2013;35:2251–2258.10.1007/s11738-013-1261-6
- Arai T, Toda Y, Kato K, et al. Artabolide, a novel polar auxin transport inhibitor isolated from Artemisia absinthium. Tetrahedron. 2013;69:7001–7005.10.1016/j.tet.2013.06.052
- Sukumar P, Edwards KS, Rahman A, et al. PINOID kinase regulates root gravitropism through modulation of PIN2-dependent basipetal auxin transport in arabidopsis. Plant Physiol. 2009;150:722–735.10.1104/pp.108.131607
- Hayashi K, Nakamura S, Fukunaga S, et al. Auxin transport sites are visualized in planta using fluorescent auxin analogs. Proc Natl Acad Sci U S A. 2014;111:11557–11562.10.1073/pnas.1408960111
- Gleason C, Foley RC, Singh KB. Mutant analysis in arabidopsis provides insight into the molecular mode of action of the auxinic herbicide dicamba. PLoS One. 2011;6:e17245.10.1371/journal.pone.0017245
- Simon S, Kubeš M, Baster P, et al. Defining the selectivity of processes along the auxin response chain: a study using auxin analogues. New Phytol. 2013;200:1034–1048.10.1111/nph.12437
- Prigge M, Greenham K, Zhang Y, et al. The arabidopsis auxin receptor F-Box Proteins AFB4 and AFB5 are required for response to the synthetic auxin picloram. G3 (Bethesda). 2016;6:1383–1390.10.1534/g3.115.025585
- Calderón Villalobos LIA, Lee S, De Oliveira C, et al. A combinatorial TIR1/AFB–Aux/IAA co-receptor system for differential sensing of auxin. Nat Chem Biol. 2012;8:477–485.10.1038/nchembio.926
- Hayashi K, Tan X, Zheng N, et al. Small-molecule agonists and antagonists of F-box protein-substrate interactions in auxin perception and signaling. Proc Natl Acad Sci U S A. 2008;105:5632–5637.10.1073/pnas.0711146105
- Hayashi K, Neve J, Hirose M, et al. Rational design of an auxin antagonist of the SCF (TIR1) auxin receptor complex. ACS Chem. Biol. 2012;7:590–598.10.1021/cb200404c
- Ye Y, Gong Z, Lu X, et al. Germostatin resistance locus 1 encodes a PHD finger protein involved in auxin-mediated seed dormancy and germination. Plant J. 2016;85:3–15.10.1111/tpj.13086
- Ye Y, Zhao Y. The pleiotropic effects of the seed germination inhibitor germostatin. Plant Signal Behav. 2016;11:e1144000.10.1080/15592324.2016.1144000
- Hedden P, Sponsel V. A century of gibberellin research. J Plant Growth Regul. 2015;34:740–760.10.1007/s00344-015-9546-1
- Yamaguchi S. Gibberellin metabolism and its regulation. Annu Rev Plant Biol. 2008;59:225–251.10.1146/annurev.arplant.59.032607.092804
- Rademacher W. Growth retardants : effects on gibberellin. Annu Rev Plant Physiol Plant Mol Biol. 2000;51:501–531.10.1146/annurev.arplant.51.1.501
- Rademacher W. Plant growth regulators: backgrounds and uses in plant production. J Plant Growth Regul. 2015;34:845–872.10.1007/s00344-015-9541-6
- Nakayama I, Miyazawa T, Kobayashi M, et al. Effects of a new plant growth regulator prohexadione calcium (BX-112) on shoot elongation caused by exogenously applied gibberellins in rice (Oryza sativa L.) Seedlings. Plant Cell Physiol. 1990;31:195–200.
- Otani M, Yoon J-M, Park S-H, et al. Screening and characterization of an inhibitory chemical specific to Arabidopsis gibberellin 2-oxidases. Bioorg Med. Chem Lett. 2010;20:4259–4262.10.1016/j.bmcl.2010.05.015
- Shani E, Weinstain R, Zhang Y, et al. Gibberellins accumulate in the elongating endodermal cells of Arabidopsis root. Proc Natl Acad Sci U S A. 2013;110:4834–4839.10.1073/pnas.1300436110
- Schayek H, Shani E, Weinstain R. Highlighting gibberellins accumulation sites in Arabidopsis thaliana root using fluorescently labeled gibberellins. Methods Mol Biol. 2017;1497:91–97.10.1007/978-1-4939-6469-7
- Tal I, Zhang Y, Jørgensen ME, et al. The Arabidopsis NPF3 protein is a GA transporter. Nat Commun. 2016;7:11486.10.1038/ncomms11486
- Ueguchi-Tanaka M, Ashikari M, Nakajima M, et al. GIBBERELLIN INSENSITIVE DWARF1 encodes a soluble receptor for gibberellin. Nature. 2005;437:693–698.10.1038/nature04028
- Nakajima M, Shimada A, Takashi Y, et al. Identification and characterization of Arabidopsis gibberellin receptors. Plant J. 2006;46:880–889.10.1111/tpj.2006.46.issue-5
- Hoad GV, Phinney BO, Sponsel VM, et al. The biological activity of sixteen gibberellin A4 and gibberellin A9 derivatives using seven bioassays. Phytochemistry. 1981;20:703–713.10.1016/0031-9422(81)85159-X
- Yamauchi Y, Takeda-Kamiya N, Hanada A, et al. Contribution of gibberellin deactivation by AtGA2ox2 to the suppression of germination of dark-imbibed arabidopsis thaliana seeds. Plant Cell Physiol. 2007;48:555–561.10.1093/pcp/pcm023
- Tamura S, Sakurai A, Kainuma K, et al. Isolation of helminthosporol as a natural plant growth regulator and its chemical structure. Agric Biol Chem. 1963;27:738–739.10.1271/bbb1961.27.738
- Sakurai A, Tamura S. Agricultural and biological chemistry syntheses of several compounds related to helminthosporol and their plant-growth regulating activities syntheses of several compounds related to helminthosporol and their plant-growth regulating activities. Agric Biol Chem. 1965;29:407–411.10.1080/00021369.1965.10858404
- Briggs DE. Gibberellin-like activity of helminthosporol and helminthosporic acid. Nature. 1966;210:418–419.10.1038/210418b0
- Miyazaki S, Jiang K, Kobayashi M, et al. Helminthosporic acid functions as an agonist for gibberellin receptor. Biosci Biotechnol Biochem. 2017;81:2152–2159.10.1080/09168451.2017.1381018
- Metzger JD. Promotion of germination of dormant weed seeds by substituted phthalimides and gibberellic acid. Weed Sci. 1983;31:285–289.
- Donald WW. AC-94,377 for breaking dormancy of wild mustard seed in soil. Farm res. 1985;43:28–31.
- Yalpani N, Suttle JC, Hultstrand JF, et al. Competition for in vitro [3H]gibberellin A(4) binding in cucumber by substituted phthalimides: comparison with in vivo gibberellin-like activity. Plant Physiol. 1989;91:823–828.10.1104/pp.91.3.823
- Rodaway SJ, Gates DW, Brindle C. Control of early seedling growth in varietal lines of hexaploid wheat (Triticum aestivum), durum wheat (Triticum durum), and barley (Hordeum vulgare) in response to the phthalimide growth regulant, AC 94,377. Plant Growth Regul. 1991;10:243–259.10.1007/BF00024415
- Jiang K, Otani M, Shimotakahara H, et al. Substituted phthalimide AC94377 is a selective agonist of the gibberellin receptor GID1. Plant Physiol. 2017;173:825–835.10.1104/pp.16.00937
- Gott KA, Thomas TH. Comparative effects of gibberellins and an N-substituted phthalimide on seed germination and extension growth of celery (Apium graveolens L.). Plant Growth Regul. 1986;4:273–279.10.1007/BF00028170
- Jiang K, Shimotakahara H, Luo M, et al. Chemical screening and development of novel gibberellin mimics. Bioorg Med Chem Lett. 2017;27:3678–3682.10.1016/j.bmcl.2017.07.012
- Yoon JM, Nakajima M, Mashiguchi K, et al. Chemical screening of an inhibitor for gibberellin receptors based on a yeast two-hybrid system. Bioorganic Med Chem Lett. 2013;23:1096–1098.10.1016/j.bmcl.2012.12.007
- Sakakibara H. Cytokinins: activity, biosynthesis, and translocation. Annu Rev Plant Biol. 2006;57:431–449.10.1146/annurev.arplant.57.032905.105231
- Werner T, Schmülling T. Cytokinin action in plant development. Curr Opin Plant Biol. 2009;12:527–538.10.1016/j.pbi.2009.07.002
- Miller CO, Skoog F, Okomura FS, et al. Isolation, structure and synthesis of kinetin, a substrance promoting cell division. J Am Chem Soc. 1956;78:1375–1380.10.1021/ja01588a032
- Koshimizu K, Matsubara S, Kusaki T, et al. Isolation of a new cytokinin from immature yellow lupin seeds. Agric Biol Chem. 1967;31:795–801.10.1080/00021369.1967.10858881
- Koshimizu. Isolation of a cytokinin, (-)-dihydrozeatin, from immature seeds of Lupinus luteus. Tetrahedron Lett. 1967;8:1317–1320.
- Letham DS. Regulators of cell division in plant tissues—II. A cytokinin in plant extracts: Isolation and interaction with other growth regulators. Phytochemistry. 1966;5:269–286.10.1016/S0031-9422(00)82141-X
- Letham DS, Palni LMS. The biosynthesis and metabolism of cytokinins. Ann Rev Plant Physiol. 1983;34:163–197.10.1146/annurev.pp.34.060183.001115
- Frebort I, Kowalska M, Hluska T, et al. Evolution of cytokinin biosynthesis and degradation. J Exp Bot. 2011;62:2431–2452.10.1093/jxb/err004
- Zürcher E, Müller B. Cytokinin synthesis, signaling, and function-advances and new insights. Int Rev Cell Mol Biol. 2016;324:1–38.
- Sasaki E, Ogura T, Takei K, et al. Uniconazole, a cytochrome P450 inhibitor, inhibits trans-zeatin biosynthesis in Arabidopsis. Phytochemistry. 2013;87:30–38.10.1016/j.phytochem.2012.11.023
- Sakakibara H. Cytokinin biosynthesis and regulation. Vitam Horm. 2005;72:271–287.10.1016/S0083-6729(05)72008-2
- Durán-Medina Y, Díaz-Ramírez D, Marsch-Martínez N. Cytokinins on the move. Front Plant Sci. 2017;8:146.
- Inoue T, Higuchi M, Hashimoto Y, et al. Identification of CRE1 as a cytokinin receptor from Arabidopsis. Nature. 2001;409:1060–1063.10.1038/35059117
- Suzuki T, Miwa K, Ishikawa K, et al. The arabidopsis sensor his-kinase, AHK4, can respond to cytokinins. Plant Cell Physiol. 2001;42:107–113.10.1093/pcp/pce037
- Ueguchi C, Sato S, Kato T, et al. The AHK4 gene involved in the cytokinin-signaling pathway as a direct receptor molecule in arabidopsis thaliana. Plant Cell Physiol. 2001;42:751–755.10.1093/pcp/pce094
- Yamada H, Suzuki T, Terada K, et al. The arabidopsis AHK4 histidine kinase is a cytokinin-binding receptor that transduces cytokinin signals across the membrane. Plant Cell Physiol. 2001;42:1017–1023.10.1093/pcp/pce127
- Yonekura-Sakakibara K, Kojima M, Yamaya T, et al. Molecular characterization of cytokinin-responsive histidine kinases in maize. Differential ligand preferences and response to cis-zeatin. Plant Physiol. 2004;134:1654–1661.10.1104/pp.103.037176
- Lomin SN, Krivosheev DM, Steklov MY, et al. Receptor properties and features of cytokinin signaling. Acta Naturae. 2012;4:31–45.
- Kieber JJ, Schaller GE. Cytokinins. Arab B. 2014;12:e0168.10.1199/Tab.0168
- Spíchal L, Krystof V, Paprskárová M, et al. Classical anticytokinins do not interact with cytokinin receptors but inhibit cyclin-dependent kinases. J Biol Chem. 2007;282:14356–14363.10.1074/jbc.M609750200
- Spíchal L, Werner T, Popa I, et al. The purine derivative PI-55 blocks cytokinin action via receptor inhibition. FEBS J. 2009;276:244–253.10.1111/j.1742-4658.2008.06777.x
- Arata Y, Nagasawa-Iida A, Uneme H, et al. The phenylquinazoline compound S-4893 is a Non-competitive cytokinin antagonist that targets arabidopsis cytokinin receptor CRE1 and promotes root growth in arabidopsis and rice. Plant Cell Physiol. 2010;51:2047–2059.10.1093/pcp/pcq163
- Hothorn M, Dabi T, Chory J. Structural basis for cytokinin recognition by Arabidopsis thaliana histidine kinase 4. Nat Chem Biol. 2011;7:766–768.10.1038/nchembio.667
- Ohkuma K, Lyon JL, Addicott FT, et al. Abscisin II, an abscission-accelerating substance from young cotton fruit. Science. 1963;142:1592–1593.10.1126/science.142.3599.1592
- Finkelstein R. Abscisic acid synthesis and response. Arab. B. 2013;11:e0166.10.1199/Tab.0166
- Lim CW, Baek W, Jung J, et al. Function of ABA in stomatal defense against biotic and drought stresses. Int J Mol Sci. 2015;16:15251–15270.10.3390/ijms160715251
- Aleman F, Yazaki J, Lee M, et al. An ABA-increased interaction of the PYL6 ABA receptor with MYC2 transcription factor: A putative link of ABA and JA signaling. Sci Rep. 2016;6:28941.10.1038/srep28941
- Xu J, Audenaert K, Hofte M, et al. Abscisic acid promotes susceptibility to the rice leaf blight pathogen xanthomonas oryzae pv oryzae by suppressing salicylic acid-mediated defenses. PLoS One. 2013;8:e67413.10.1371/journal.pone.0067413
- Meguro A, Sato Y. Salicylic acid antagonizes abscisic acid inhibition of shoot growth and cell cycle progression in rice. Sci Rep. 2014;4:4555.
- Xiong L. Regulation of abscisic acid biosynthesis. Plant Physiol. 2003;133:29–36.10.1104/pp.103.025395
- Nambara E, Marion-Poll A. Abscisic acid biosynthesis and catabolism. Annu Rev Plant Biol. 2005;56:165–185.10.1146/annurev.arplant.56.032604.144046
- Endo A, Okamoto M, Koshiba T. ABA biosynthetic and catabolic pathways. Abscisic acid: Metab Transp Signal. 2014:21–45.
- Dong T, Park Y, Hwang I. Abscisic acid: biosynthesis, inactivation, homoeostasis and signalling. Essays Biochem. 2015;58:29–48.10.1042/bse0580029
- Gamble PE, Mullet JE. Inhibition of carotenoid accumulation and abscisic acid biosynthesis in fluridone-treated dark-grown barley. Eur J Biochem. 1986;160:117–121.10.1111/ejb.1986.160.issue-1
- Xie X, Yoneyama K, Yoneyama K. The strigolactone story. Annu Rev Phytopathol. 2010;48:93–117.10.1146/annurev-phyto-073009-114453
- Al-Babili S, Bouwmeester HJ. Strigolactones, a novel carotenoid-derived plant hormone. Annu Rev Plant Biol. 2015;66:161–186.10.1146/annurev-arplant-043014-114759
- Jamil M, Charnikhova T, Verstappen F, et al. Carotenoid inhibitors reduce strigolactone production and Striga hermonthica infection in rice. Arch Biochem Biophys. 2010;504:123–131.10.1016/j.abb.2010.08.005
- Rasmussen A, Beveridge CA, Geelen D. Inhibition of strigolactones promotes adventitious root formation. Plant Signal Behav. 2012;7:694–697.10.4161/psb.20224
- Han S-Y, Kitahata N, Sekimata K, et al. A novel inhibitor of 9-cis-epoxycarotenoid dioxygenase in abscisic acid biosynthesis in higher plants. Plant Physiol. 2004;135:1574–1582.10.1104/pp.104.039511
- Han S young, Inoue H, Terada T, et al. Design and synthesis of lignostilbene-alpha,beta-dioxygenase inhibitors. Bioorg Med Chem Lett. 2002;12:1139–1142.10.1016/S0960-894X(02)00126-9
- Han S-Y, Inoue H, Terada T, et al. N-benzylideneaniline and N-benzylaniline are potent inhibitors of lignostilbene-alpha, beta-dioxygenase, a key enzyme in oxidative cleavage of the central double bond of lignostilbene. J Enzyme Inhib Med Chem. 2003;18:279–283.10.1080/1475636031000080207
- Han S, Kitahata N, Saito T, et al. A new lead compound for abscisic acid biosynthesis inhibitors targeting 9-cis-epoxycarotenoid dioxygenase. Bioorg Med Chem Lett. 2004;14:3033–3036.10.1016/j.bmcl.2004.04.035
- Kitahata N, Han S-Y, Noji N, et al. A 9-cis-epoxycarotenoid dioxygenase inhibitor for use in the elucidation of abscisic acid action mechanisms. Bioorg Med Chem. 2006;14:5555–5561.10.1016/j.bmc.2006.04.025
- Boyd J, Gai Y, Nelson KM, et al. Sesquiterpene-like inhibitors of a 9-cis-epoxycarotenoid dioxygenase regulating abscisic acid biosynthesis in higher plants. Bioorg Med Chem. 2009;17:2902–2912.10.1016/j.bmc.2009.01.076
- KrochkoJE, Abrams AD, Loewen MKet al. (+)-abscisic acid 8′-hydroxylase is a cytochrome P450 monooxygenase. Plant Physiol. 1998;118:849–860.10.1104/pp.118.3.849
- Kushiro T, Okamoto M, Nakabayashi K, et al. The Arabidopsis cytochrome P450 CYP707A encodes ABA 8’-hydroxylases: key enzymes in ABA catabolism. EMBO J. 2004;23:1647–1656.10.1038/sj.emboj.7600121
- Saito S, Hirai N, Matsumoto C, et al. Arabidopsis CYP707As encode (+)-abscisic acid 8'-hydroxylase, a key enzyme in the oxidative catabolism of abscisic acid. Plant Physiol. 2004;134:1439–1449.10.1104/pp.103.037614
- Kitahata N, Saito S, Miyazawa Y, et al. Chemical regulation of abscisic acid catabolism in plants by cytochrome P450 inhibitors. Bioorganic Med Chem. 2005;13:4491–4498.10.1016/j.bmc.2005.04.036
- Saito S, Okamoto M, Shinoda S, et al. A plant growth retardant, uniconazole, is a potent inhibitor of ABA catabolism in arabidopsis. Biosci Biotechnol Biochem. 2006;70:1731–1739.10.1271/bbb.60077
- Todoroki Y, Aoyama H, Hiramatsu S, et al. Enlarged analogues of uniconazole, new azole containing inhibitors of ABA 8′-hydroxylase CYP707A. Bioorg Med Chem Lett. 2009;19:5782–5786.10.1016/j.bmcl.2009.07.137
- Okazaki M, Nimitkeatkai H, Muramatsu T, et al. Abscinazole-E1, a novel chemical tool for exploring the role of ABA 8′-hydroxylase CYP707A. Bioorg Med Chem. 2011;19:406–413.10.1016/j.bmc.2010.11.011
- Okazaki M, Kittikorn M, Ueno K, et al. Abscinazole-E2B, a practical and selective inhibitor of ABA 8’-hydroxylase CYP707A. Bioorg Med Chem. 2012;20:3162–3172.10.1016/j.bmc.2012.03.068
- Todoroki Y, Kobayashi K, Shirakura M, et al. Abscinazole-F1, a conformationally restricted analogue of the plant growth retardant uniconazole and an inhibitor of ABA 8′-hydroxylase CYP707A with no growth-retardant effect. Bioorg Med Chem. 2009;17:6620–6630.10.1016/j.bmc.2009.07.070
- Todoroki Y, Naiki K, Aoyama H, et al. Selectivity improvement of an azole inhibitor of CYP707A by replacing the monosubstituted azole with a disubstituted azole. Bioorg Med Chem Lett. 2010;20:5506–5509.10.1016/j.bmcl.2010.07.067
- Takeuchi J, Okamoto M, Mega R, et al. Abscinazole-E3M, a practical inhibitor of abscisic acid 8’-hydroxylase for improving drought tolerance. Sci Rep. 2016;6:37060.10.1038/srep37060
- Araki Y, Miyawaki A, Miyashita T, et al. A new non-azole inhibitor of ABA 8′-hydroxylase: Effect of the hydroxyl group substituted for geminal methyl groups in the six-membered ring. Bioorg Med Chem Lett. 2006;16:3302–3305.10.1016/j.bmcl.2006.03.024
- Weng J-K, Ye M, Li B, et al. Co-evolution of hormone metabolism and signaling networks expands plant adaptive plasticity. Cell. 2016;166:881–893.10.1016/j.cell.2016.06.027
- Lim EK, Doucet CJ, Hou B, et al. Resolution of (+)-abscisic acid using an Arabidopsis glycosyltransferase. Tetrahedron Asymmetry. 2005;16:143–147.10.1016/j.tetasy.2004.11.062
- Xu Z-J, Nakajima M, Suzuki Y, et al. Cloning and characterization of the abscisic acid-specific glucosyltransferase gene from adzuki bean seedlings. Pant Physiol. 2002;129:1285–1295.
- Hartung W, Sauter A, Hose E. Abscisic acid in the xylem: where does it come from, where does it go to? J Exp Bot. 2002;53:27–32.10.1093/jexbot/53.366.27
- Sauter A, Dietz K-J, Hartung W. A possible stress physiological role of abscisic acid conjugates in root-to-shoot signalling. Plant Cell Environ. 2002;25:223–228.10.1046/j.1365-3040.2002.00747.x
- Lee KH, Piao HL, Kim H-Y, et al. Activation of glucosidase via stress-induced polymerization rapidly increases active pools of abscisic acid. Cell. 2006;126:1109–1120.10.1016/j.cell.2006.07.034
- Kang J, Hwang J-U, Lee M, et al. PDR-type ABC transporter mediates cellular uptake of the phytohormone abscisic acid. Proc Natl Acad Sci U S A. 2010;107:2355–2360.10.1073/pnas.0909222107
- Kuromori T, Miyaji T, Yabuuchi H, et al. ABC transporter AtABCG25 is involved in abscisic acid transport and responses. Proc Natl Acad Sci U S A. 2010;107:2361–2366.10.1073/pnas.0912516107
- Kanno Y, Hanada A, Chiba Y, et al. Identification of an abscisic acid transporter by functional screening using the receptor complex as a sensor. Proc Natl Acad Sci U S A. 2012;109:9653–9658.10.1073/pnas.1203567109
- Ma Y, Szostkiewicz I, Korte A, et al. Regulators of PP2C phosphatase activity function as abscisic acid sensors. Science. 2009;324:1064–1068.
- Miyakawa T, Fujita Y, Yamaguchi-Shinozaki K, et al. Structure and function of abscisic acid receptors. Trends Plant Sci. 2013;18:259–266.10.1016/j.tplants.2012.11.002
- Okamoto M, Peterson FC, Defries A, et al. Activation of dimeric ABA receptors elicits guard cell closure, ABA-regulated gene expression, and drought tolerance. Proc Natl Acad Sci U S A. 2013;110:12132–12137.10.1073/pnas.1305919110
- Cao M, Liu X, Zhang Y, et al. An ABA-mimicking ligand that reduces water loss and promotes drought resistance in plants. Cell Res. 2013;23:1043–1054.10.1038/cr.2013.95
- Miyazono K-I, Miyakawa T, Sawano Y, et al. Structural basis of abscisic acid signalling. Nature. 2009;462:609–614.10.1038/nature08583
- Melcher K, Ng L-M, Zhou XE, et al. A gate-latch-lock mechanism for hormone signalling by abscisic acid receptors. Nature. 2009;462:602–608.10.1038/nature08613
- Nishimura N, Hitomi K, Arvai AS, et al. Structural mechanism of abscisic acid binding and signaling by dimeric PYR1. Science. 2009;326:1372–1379.
- Yin P, Fan H, Hao Q, et al. Structural insights into the mechanism of abscisic acid signaling by PYL proteins. Nat Struct Mol Biol. 2009;16:1230–1236.10.1038/nsmb.1730
- Takeuchi J, Okamoto M, Akiyama T, et al. Designed abscisic acid analogs as antagonists of PYL-PP2C receptor interactions. Nat Chem Biol. 2014;10:477–482.10.1038/nchembio.1524
- Ye Y, Zhou L, Liu X, et al. A novel chemical inhibitor of ABA signaling targets all ABA receptors. Plant Physiol. 2017;173:2356–2369.10.1104/pp.16.01862
- Wilen RW, Hays DB, Mandel RM, et al. Competitive inhibition of abscisic acid-regulated gene expression by stereoisomeric acetylenic analogs of abscisic acid. Plant Physiol. 1993;101:469–476.10.1104/pp.101.2.469
- Asami T, Kim B-T, Morita K, et al. Inhibition of α -amylase induction in barley seed and the induction of stomatal pore closure by artificial abscisic acid analogs (RCA series). Biosci Biotechnol Biochem. 1992;56:2089–2090.10.1271/bbb.56.2089
- Asami T, Kim B-T, Yoshida S. One pot synthesis and optical resolution of synthetic mimic of abscisic acid affecting plant’s physiology. Tetrahedron Lett. 1994;35:6117–6118.10.1016/0040-4039(94)88091-3
- Asami T, Robertson M, Yamamoto S, et al. Biological activities of an abscisic acid analog in barley, cress, and rice. Plant Cell Physiol. 1998;39:342–348.10.1093/oxfordjournals.pcp.a029375
- Park S-Y, Peterson FC, Mosquna A, et al. Agrochemical control of plant water use using engineered abscisic acid receptors. Nature. 2015;520:545–548.10.1038/nature14123
- Nakashima K, Fujita Y, Kanamori N, et al. Three arabidopsis SnRK2 Protein Kinases, SRK2D/SnRK2.2, SRK2E/SnRK2.6/OST1 and SRK2I/SnRK2.3, involved in ABA signaling are essential for the control of seed development and dormancy. Plant Cell Physiol. 2009;50:1345–1363.10.1093/pcp/pcp083
- Fujita Y, Nakashima K, Yoshida T, et al. Three SnRK2 protein kinases are the main positive regulators of abscisic acid signaling in response to water stress in arabidopsis. Plant Cell Physiol. 2009;50:2123–2132.10.1093/pcp/pcp147
- Fujii H, Zhu J-K. Arabidopsis mutant deficient in 3 abscisic acid-activated protein kinases reveals critical roles in growth, reproduction, and stress. Proc Natl Acad Sci U S A. 2009;106:8380–8385.10.1073/pnas.0903144106
- Umezawa T, Sugiyama N, Mizoguchi M, et al. Type 2C protein phosphatases directly regulate abscisic acid-activated protein kinases in Arabidopsis. Proc Natl Acad Sci U S A. 2009;106:17588–17593.10.1073/pnas.0907095106
- Yoshida T, Nishimura N, Kitahata N, et al. ABA-hypersensitive germination3 encodes a protein phosphatase 2C (AtPP2CA) that strongly regulates abscisic acid signaling during germination among Arabidopsis protein phosphatase 2Cs. Plant Physiol. 2006;140:115–126.
- Yoshida R, Umezawa T, Mizoguchi T, et al. The regulatory domain of SRK2E/OST1/SnRK2.6 interacts with ABI1 and integrates abscisic acid (ABA) and osmotic stress signals controlling stomatal closure in arabidopsis. J Biol Chem. 2006;281:5310–5318.10.1074/jbc.M509820200
- Kende H. Plant biology and the nobel prize. Science. 1998;282:627.10.1126/science.282.5389.627b
- Larsen PB. Mechanisms of ethylene biosynthesis and response in plants. Essays Biochem. 2015;58:61–70.10.1042/bse0580061
- Yang SF, Hoffman NE. Ethylene biosynthesis and its regulation in higher plants. Annu Rev Plant Physiol. 1984;35:155–189.10.1146/annurev.pp.35.060184.001103
- Owens LD, Lieberman M, Kunishi A. Inhibition of ethylene production by rhizobitoxine. Plant Physiol. 1971;48:1–4.10.1104/pp.48.1.1
- Boller T, Herner RC, Kende H. Assay for and enzymatic formation of an ethylene precursor, 1-aminocyclopropane-1-carboxylic acid. Planta. 1979;145:293–303.10.1007/BF00454455
- Yu Y-B, Yang SF. Auxin-induced ethylene production and its inhibition by aminoethyoxyvinylglycine and cobalt ion. Plant Physiol. 1979;64:1074–1077.10.1104/pp.64.6.1074
- Bradford kJ, Hsiao TC, Yang SF. Inhibition of ethylene synthesis in tomato plants subjected to anaerobic root stress. Plant Physiol. 1982;70:1503–1507.10.1104/pp.70.5.1503
- Satoh S, Esashi Y. Alpha-aminoisabutyric acid, propyl gallate and cobalt ion and the mode of inhibition of ethylene production by cotyledonary segments of cocklebur seeds. Physiol Plant. 1983;57:521–526.10.1111/ppl.1983.57.issue-4
- Kosugi Y, Matsuoka A, Higashi A, et al. 2-Aminooxyisobutyric acid inhibits the in vitro activities of both 1-aminocyclopropane-1-carboxylate (ACC) synthase and ACC oxidase in ethylene biosynthetic pathway and prolongs vase life of cut carnation flowers. J Plant Biol. 2014;57:218–224.10.1007/s12374-014-0180-4
- Satoh S, Esashi Y. alpha-Aminoisabutyric acid, propyl gallate and cobalt ion and the mode of inhibition of ethylene production by cotyledonary segments of cocklebur seeds. Physiol Plant. 1983;57:521–526.10.1111/ppl.1983.57.issue-4
- Sun X, Li Y, He W, et al. Pyrazinamide and derivatives block ethylene biosynthesis by inhibiting ACC oxidase. Nat Commun. 2017;8:15758.10.1038/ncomms15758
- Guo H, Ecker JR. The ethylene signaling pathway: new insights. Curr Opin Plant Biol. 2004;7:40–49.10.1016/j.pbi.2003.11.011
- Ju C, Chang C. Mechanistic insights in ethylene perception and signal transduction. Plant Physiol. 2015;169:85–95.10.1104/pp.15.00845
- Zhang F, Qi B, Wang L, et al. EIN2-dependent regulation of acetylation of histone H3K14 and non-canonical histone H3K23 in ethylene signalling. Nat Commun. 2016;7:13018.10.1038/ncomms13018
- Zhang F, Wang L, Qi B, et al. EIN2 mediates direct regulation of histone acetylation in the ethylene response. Proc Natl Acad Sci U S A. 2017;114:10274–10279.10.1073/pnas.1707937114
- Burg SP, Burg EA. Molecular requirements for the biological activity of ethylene. Plant Physiol. 1967;42:144–152.10.1104/pp.42.1.144
- Sisler EC, Blankenship SM, Guest M. Competition of cyclooctenes and cyclooctadienes for ethylene binding and activity in plants. Plant Growth Regul. 1990;9:157–164.10.1007/BF00027443
- Schaller GE, Bleecker AB. Ethylene-binding sites generated in yeast expressing the arabidopsis ETR1 gene. Science. 1995;270:1809–1811.10.1126/science.270.5243.1809
- Serek M, Tamari G, Sisler EC, et al. Inhibition of ethylene-induced cellular senescence symptoms by 1-methylcyclopropene, a new inhibitor of ethylene action. Physiol Plant. 1995;94:229–232.10.1111/ppl.1995.94.issue-2
- Sisler EC, Blankenship SM. Diazocyclopentadiene (DACP), a light sensitive reagent for the ethylene receptor in plants. Plant Growth Regul. 1993;12:125–132.10.1007/BF00144593
- Sisler EC, Serek M. Compounds interacting with the ethylene receptor in plants. Plant Biol. 2003;5:473–480.10.1055/s-2003-44782
- Hirayama T, Kieber JJ, Hirayama N, et al. Responsive-to-antagonist1, a Menkes/Wilson disease–related copper transporter, is required for ethylene signaling in arabidopsis. Cell. 1999;97:383–393.10.1016/S0092-8674(00)80747-3
- Thompson JS, Harlow RL, Whitney JF. Copper(I)-olefin complexes. Support for the proposed role of copper in the ethylene effect in plants. J Am Chem Soc. 1983;105:3522–3527.10.1021/ja00349a026
- Li W, Lacey RF, Ye Y, et al. Triplin, a small molecule, reveals copper ion transport in ethylene signaling from ATX1 to RAN1. PLoS Genet. 2017;13:e1006703.10.1371/journal.pgen.1006703
- Clouse SD. Brassinosteroids. Arab. B. 2011;9:e0151.10.1199/Tab.0151
- Zhu J-Y, Sae-Seaw J, Wang Z-Y. Brassinosteroid signalling. Development. 2013;140:1615–1620.
- Li J, Nagpal P, Vitart V, et al. A role for brassinosteroids in light-dependent development of arabidopsis. Science. 1996;272:398–401.10.1126/science.272.5260.398
- Szekeres M, Németh K, Koncz-Kálmán Z, et al. Brassinosteroids rescue the deficiency of CYP90, a cytochrome P450, controlling cell elongation and de-etiolation in arabidopsis. Cell. 1996;85:171–182.10.1016/S0092-8674(00)81094-6
- Choe S, Dilkes BP, Fujioka S, et al. The DWF4 gene of Arabidopsis encodes a cytochrome P450 that mediates multiple 22alpha-hydroxylation steps in brassinosteroid biosynthesis. Plant Cell. 1998;10:231–243.
- Choe S, Dilkes BP, Gregory BD, et al. The arabidopsis dwarf1 mutant is defective in the conversion of 24-methylenecholesterol to campesterol in brassinosteroid biosynthesis. Plant Physiol. 1999;119:897–907.10.1104/pp.119.3.897
- Gachotte D, Husselstein T, Bard M, et al. Isolation and characterization of an Arabidopsis thaliana cDNA encoding a delta 7-sterol-C-5-desaturase by functional complementation of a defective yeast mutant. Plant J. 1996;9:391–398.10.1046/j.1365-313X.1996.09030391.x
- Choe S, Noguchi T, Fujioka S, et al. The Arabidopsis dwf7/ste1 mutant is defective in the delta7 sterol C-5 desaturation step leading to brassinosteroid biosynthesis. Plant Cell. 1999;11:207–221.
- Asami T, Yoshida S. Brassinosteroid biosynthesis inhibitors. Trends Plant Sci. 1999;4:348–353.10.1016/S1360-1385(99)01456-9
- Chung Y, Choe S. The regulation of brassinosteroid biosynthesis in arabidopsis. CRC. Crit Rev Plant Sci. 2013;32:396–410.10.1080/07352689.2013.797856
- Clouse SD, Langford M, McMorris TC. A brassinosteroid-insensitive mutant in arabidopsis thaliana exhibits multiple defects in growth and development. Plant Physiol. 1996;111:671–678.10.1104/pp.111.3.671
- Li J, Chory J, Wilford R, et al. A putative leucine-rich repeat receptor kinase involved in brassinosteroid signal transduction. Cell. 1997;90:929–938.10.1016/S0092-8674(00)80357-8
- Friedrichsen DM, Joazeiro CAP, Li J, et al. Brassinosteroid-insensitive-1 is a ubiquitously expressed leucine-rich repeat receptor serine/threonine kinase. Plant Physiol. 2000;123:1247–1255.10.1104/pp.123.4.1247
- He Z, Wang ZY, Li J, et al. Perception of brassinosteroids by the extracellular domain of the receptor kinase BRI1. Science. 2000;288:2360–2363.10.1126/science.288.5475.2360
- Wang Z-Y, Seto H, Fujioka S, et al. BRI1 is a critical component of a plasma-membrane receptor for plant steroids. Nature. 2001;410:380–383.10.1038/35066597
- Asami T, Min YK, Nagata N, et al. Characterization of brassinazole, a triazole-type brassinosteroid biosynthesis inhibitor. Plant Physiol. 2000;123:93–100.10.1104/pp.123.1.93
- Asami T, Mizutani M, Fujioka S, et al. Selective interaction of triazole derivatives with DWF4, a Cytochrome P450 monooxygenase of the brassinosteroid biosynthetic pathway, correlates with brassinosteroid deficiency in planta. J Biol Chem. 2001;276:25687–25691.10.1074/jbc.M103524200
- Sekimata K, Kimura T, Kaneko I, et al. A specific brassinosteroid biosynthesis inhibitor, Brz 2001: evaluation of its effects on Arabidopsis, cress, tobacco, and rice. Planta. 2001;213:716–721.10.1007/s004250100546
- Sekimata K, Han S-Y, Yoneyama K, et al. A specific and potent inhibitor of brassinosteroid biosynthesis possessing a dioxolane ring. J Agric Food Chem. 2002;50:3486–3490.10.1021/jf011716w
- Oh K, Yamada K, Asami T, et al. Synthesis of novel brassinosteroid biosynthesis inhibitors based on the ketoconazole scaffold. Bioorg Med Chem Lett. 2012;22:1625–1628.10.1016/j.bmcl.2011.12.120
- Yamada K, Yoshizawa Y, Oh K. Synthesis of 2RS,4RS-1-[2-Phenyl-4-[2-(2-trifluromethoxy-phenoxy)-ethyl]-1,3-dioxolan-2-yl-methyl]-1H-1,2,4-triazole derivatives as potent inhibitors of brassinosteroid biosynthesis. Molecules. 2012;17:4460–4473.10.3390/molecules17044460
- Yamada K, Yajima O, Yoshizawa Y, et al. Synthesis and biological evaluation of novel azole derivatives as selective potent inhibitors of brassinosteroid biosynthesis. Bioorg Med Chem. 2013;21:2451–2461.10.1016/j.bmc.2013.03.006
- Oh K, Matsumoto T, Yamagami A, et al. Fenarimol, a pyrimidine-type fungicide, inhibits brassinosteroid biosynthesis. Int J Mol Sci. 2015;16:17273–17288.10.3390/ijms160817273
- Oh K, Matsumoto T, Yamagami A, et al. YCZ-18 Is a new brassinosteroid biosynthesis inhibitor. PLoS One. 2015;10:e0120812.10.1371/journal.pone.0120812
- Wang ZY, Nakano T, Gendron J, et al. Nuclear-localized BZR1 mediates brassinosteroid-induced growth and feedback suppression of brassinosteroid biosynthesis. Dev Cell. 2002;2:505–513.10.1016/S1534-5807(02)00153-3
- He J-X, Gendron JM, Sun Y, et al. BZR1 Is a transcriptional repressor with dual roles in brassinosteroid homeostasis and growth responses. Science. 2005;307:1634–1638.10.1126/science.1107580
- Bekh-Ochir D, Shimada S, Yamagami A, et al. A novel mitochondrial DnaJ/Hsp40 family protein BIL2 promotes plant growth and resistance against environmental stress in brassinosteroid signaling. Planta. 2013;237:1509–1525.10.1007/s00425-013-1859-3
- Yamagami A, Nakazawa M, Matsui M, et al. Chemical genetics reveal the novel transmembrane protein BIL4, which mediates plant cell elongation in brassinosteroid signaling. Biosci Biotechnol Biochem. 2009;73:415–421.10.1271/bbb.80752
- Yamagami A, Saito C, Nakazawa M, et al. Evolutionarily conserved BIL4 suppresses the degradation of brassinosteroid receptor BRI1 and regulates cell elongation. Sci Rep. 2017;7:5739.10.1038/s41598-017-06016-2
- Shimada S, Komatsu T, Yamagami A, et al. Formation and dissociation of the BSS1 protein complex regulates plant development via brassinosteroid signaling. Plant Cell. 2015;27:375–390.10.1105/tpc.114.131508
- Lin L-C, Chueh C-M, Wang L-C. Investigating the Phytohormone ethylene response pathway by chemical genetics. Methods Mol. Biol. 2014:63–77.10.1007/978-1-62703-751-8
- Belkhadir Y, Jaillais Y. The molecular circuitry of brassinosteroid signaling. New Phytol. 2015;206:522–540.10.1111/nph.13269
- Nolan T, Chen J, Yin Y. Cross-talk of brassinosteroid signaling in controlling growth and stress responses. Biochem J. 2017;474:2641–2661.10.1042/BCJ20160633
- Kinoshita T, Caño-Delgado A, Seto H, et al. Binding of brassinosteroids to the extracellular domain of plant receptor kinase BRI1. Nature. 2005;433:167–171.10.1038/nature03227
- Watanabe B. Structure-activity relationship studies of insect and plant steroid hormones. J Pestic Sci. 2015;40:146–151.10.1584/jpestics.J15-04
- Cerana R, Bonetti A, Marre MT, et al. Effects of a brassinosteroid on growth and electrogenic proton extrusion in Azuki bean epicotyls. Physiol Plant. 1983;59:23–27.10.1111/ppl.1983.59.issue-1
- Wada K, Marumo S, Ikekawa N, et al. Brassinolide and homobrassinolide promotion of lamina inclination of rice seedlings. Plant Cell Physiol. 1981;22:323–325.
- Wada K, Marumo S, Abe H, et al. A rice lamina inclination test–a micro- quantitative bioassay for brassinosteroids. Agric Biol Chem. 1984;483:719–726.
- Wada K, Kondo H, Marumo S. A simple bioassay for brassinosteroids: A wheat leaf-unrolling test. Agric Biol Chem. 1985;49:2249–2251.
- Kim SK, Yokota T, Takahashi N. Brassinosteroids in phaseolus vulgaris. Part III. 25-Methyldolichosterone. A new brassinosteroid with a tertiary butyl group from immature seed of Phaseolus vulgaris. Agric Biol Chem. 1987;51:2303–2305.
- Mori K, Takeuchi T. Brassinolide and its analogues, VIII. Synthesis of 25-methyldolichosterone, 25-methyl-2,3-diepidolichosterone, 25-methylcastasterone and 25-methylbrassinolide. Liebigs Ann der. Chemie. 1988;8:815–818.
- Watanabe B, Yamamoto S, Yokoi T, et al. Brassinolide-like activity of castasterone analogs with varied side chains against rice lamina inclination. Bioorg Med Chem. 2017;25:4566–4578.10.1016/j.bmc.2017.06.012
- Thussagunpanit J, Jutamanee K, Homvisasevongsa S, et al. Characterization of synthetic ecdysteroid analogues as functional mimics of brassinosteroids in plant growth. J Steroid Biochem. Mol. Biol. 2017;172:1–8.10.1016/j.jsbmb.2017.05.003
- Park K-H, Yokota T, Sakurai A, et al. Occurrence of Castasterone, Brassinolide and Methyl 4-Chloroindole- 3-acetate in Immature Vicia faba Seeds. Agric Biol Chem. 1987;51:3081–3086.
- Choi Y-H, Inoue T, Fujioka S, et al. Identification of brassinosteroid-like active substances in plant-cell cultures. Biosci Biotechnol Biochem. 1993;57:860–861.10.1271/bbb.57.860
- Park K-H, Park J-D, Hyun K-H, et al. Brassinosteroids and monoglycerides with brassinosteroid-like activity in immature seeds of oryza sativa and Perilla frutescens and in cultured cells of Nicotiana tabacum. Biosci Biotechnol Biochem. 1994;58:2241–2243.10.1271/bbb.58.2241
- Park K-H, Park J-D, Hyun K-H, et al. Brassinosteroids and monoglycerides in immature seeds of cassia tora as the active principles in the rice lamina inclination bioassay. Biosci Biotechnol Biochem. 1994;58:1343–1344.10.1271/bbb.58.1343
- Muto T, Todoroki Y. Brassinolide-2,3-acetonide: a brassinolide-induced rice lamina joint inclination antagonist. Bioorg Med Chem. 2013;21:4413–4419.10.1016/j.bmc.2013.04.048
- Takimoto S, Sugiura A, Minami S, et al. In silico exploration for agonists/antagonists of brassinolide. Bioorg Med Chem Lett. 2016;26:1709–1714.10.1016/j.bmcl.2016.02.054
- Sugiura A, Horoiwa S, Aoki T, et al. Discovery of a nonsteroidal brassinolide-like compound, NSBR1. J Pestic Sci. 2017;42:105–111.10.1584/jpestics.D17-035
- Irani NG, Di Rubbo S, Mylle E, et al. Fluorescent castasterone reveals BRI1 signaling from the plasma membrane. Nat Chem Biol. 2012;8:583–589.10.1038/nchembio.958
- De Rybel B, Audenaert D, Vert G, et al. Chemical inhibition of a subset of arabidopsis thaliana GSK3-like kinases activates brassinosteroid signaling. Chem. Biol. 2009;16:594–604.10.1016/j.chembiol.2009.04.008
- Rozhon W, Wang W, Berthiller F, et al. Bikinin-like inhibitors targeting GSK3/Shaggy-like kinases: characterisation of novel compounds and elucidation of their catabolism in planta. BMC Plant Biol. 2014;14:172.10.1186/1471-2229-14-172
- Wasternack C, Hause B. Jasmonates: biosynthesis, perception, signal transduction and action in plant stress response, growth and development. An update to the 2007 review in Annals of Botany. Ann Bot. 2007;2013(111):1021–1058.
- Santino A, Taurino M, De Domenico S, et al. Jasmonate signaling in plant development and defense response to multiple (a)biotic stresses. Plant Cell Rep. 2013;32:1085–1098.10.1007/s00299-013-1441-2
- Wang L, Wu J. The essential role of jasmonic acid in plant–herbivore interactions – using the wild tobacco Nicotiana attenuata as a model. J Genet Genomics. 2013;40:597–606.10.1016/j.jgg.2013.10.001
- Yan C, Xie D. Jasmonate in plant defence: sentinel or double agent? Plant Biotechnol J. 2015;13:1233–1240.10.1111/pbi.12417
- Yuan Z, Zhang D. Roles of jasmonate signalling in plant inflorescence and flower development. Curr Opin Plant Biol. 2015;27:44–51.10.1016/j.pbi.2015.05.024
- Yamane H, Sugawara J, Suzuki Y, et al. Syntheses of jasmonic acid related compounds and their structure-activity relationships on the growth of rice seedings. Agric Biol Chem. 1980;44:2857–2864.
- Yamane H, Takagi H, Abe H, et al. Identification of jasmonic acid in three species of higher plants and its biological activities. Plant Cell Physiol. 1981;22:689–697.
- Ueda J, Kato J. Isolation and identification of a senescence-promoting substance from wormwood (Artemisia absinthium L.). Plant Physiol. 1980;66:246–249.10.1104/pp.66.2.246
- Wasternack C. Jasmonates: an update on biosynthesis, signal transduction and action in plant stress response, growth and development. Ann. Bot. 2007;100:681–697.10.1093/aob/mcm079
- Browse J. The power of mutants for investigating jasmonate biosynthesis and signaling. Phytochemistry. 2009;70:1539–1546.10.1016/j.phytochem.2009.08.004
- Schaller A, Stintzi A. Enzymes in jasmonate biosynthesis – Structure, function, regulation. Phytochemistry. 2009;70:1532–1538.10.1016/j.phytochem.2009.07.032
- Wasternack C, Kombrink E. Jasmonates: structural requirements for lipid-derived signals active in plant stress responses and development. ACS Chem Biol. 2010;5:63–77.10.1021/cb900269u
- Kombrink E. Chemical and genetic exploration of jasmonate biosynthesis and signaling paths. Planta. 2012;236:1351–1366.10.1007/s00425-012-1705-z
- Pena-Cortés H, Albrecht T, Prat S, et al. Aspirin prevents wound-induced gene expression in tomato leaves by blocking jasmonic acid biosynthesis. Planta. 1993;191:123–128.
- Meesters C, Mönig T, Oeljeklaus J, et al. A chemical inhibitor of jasmonate signaling targets JAR1 in Arabidopsis thaliana. Nat Chem Biol. 2014;10:830–836.10.1038/nchembio.1591
- Staswick PE, Tiryaki I. The oxylipin signal jasmonic acid is activated by an enzyme that conjugates it to isoleucine in arabidopsis. Plant Cell. 2004;16:2117–2127.10.1105/tpc.104.023549
- Seo HS, Song JT, Cheong J-J, et al. Jasmonic acid carboxyl methyltransferase: a key enzyme for jasmonate-regulated plant responses. Proc Natl Acad Sci U S A. 2001;98:4788–4793.10.1073/pnas.081557298
- Kitaoka N, Matsubara T, Sato M, et al. Arabidopsis CYP94B3 encodes Jasmonyl-l-Isoleucine 12-Hydroxylase, a key enzyme in the oxidative catabolism of jasmonate. Plant Cell Physiol. 2011;52:1757–1765.10.1093/pcp/pcr110
- Koo AJK, Cooke TF, Howe GA. Cytochrome P450 CYP94B3 mediates catabolism and inactivation of the plant hormone jasmonoyl-L-isoleucine. Proc Natl Acad Sci U S A. 2011;108:9298–9303.10.1073/pnas.1103542108
- Heitz T, Widemann E, Lugan R, et al. Cytochromes P450 CYP94C1 and CYP94B3 catalyze two successive oxidation steps of plant hormone jasmonoyl-isoleucine for catabolic turnover. J Biol Chem. 2012;287:6296–6306.10.1074/jbc.M111.316364
- Glauser G, Grata E, Dubugnon L, et al. Spatial and temporal dynamics of jasmonate synthesis and accumulation in arabidopsis in response to wounding. J Biol Chem. 2008;283:16400–16407.10.1074/jbc.M801760200
- Miersch O, Neumerkel J, Dippe M, et al. Hydroxylated jasmonates are commonly occurring metabolites of jasmonic acid and contribute to a partial switch-off in jasmonate signaling. New Phytol. 2007;177:114–127.
- Gidda SK, Miersch O, Levitin A, et al. Biochemical and molecular characterization of a hydroxyjasmonate sulfotransferase from Arabidopsis thaliana. J Biol Chem. 2003;278:17895–17900.10.1074/jbc.M211943200
- Ueda M, Okazaki M, Ueda K, et al. A Leaf-Closing substance of albizzia julibrissin durazz. Tetrahedron. 2000;56:8101–8105.10.1016/S0040-4020(00)00729-8
- Ueda M, Nakamura Y. Chemical basis of plant leaf movement. Plant Cell Physiol. 2007;48:900–907.10.1093/pcp/pcm060
- Nakamura Y, Miyatake R, Inomata S, et al. Synthesis and bioactivity of potassium beta-D-glucopyranosyl 12-hydroxy jasmonate and related compounds. Biosci Biotechnol Biochem. 2008;72:2867–2876.10.1271/bbb.80338
- Nakamura Y, Mithöfer A, Kombrink E, et al. 12-Hydroxyjasmonic acid glucoside Is a COI1-JAZ-independent activator of leaf-closing movement in samanea saman. Plant Physiol. 2011;155:1226–1236.10.1104/pp.110.168617
- Feys B, Benedetti CE, Penfold CN, et al. Arabidopsis mutants selected for resistance to the phytotoxin coronatine are male sterile, insensitive to methyl jasmonate, and resistant to a bacterial pathogen. Plant cell. 1994;6:751–759.10.1105/tpc.6.5.751
- Xie D-X, Feys BF, James S, et al. COI1: an arabidopsis gene required for jasmonate-regulated defense and fertility. Science. 1998;280:1091–1094.10.1126/science.280.5366.1091
- Boter M, Ruíz-Rivero O, Abdeen A, et al. Conserved MYC transcription factors play a key role in jasmonate signaling both in tomato and Arabidopsis. Genes Dev. 2004;18:1577–1591.10.1101/gad.297704
- Dombrecht B, Xue GP, Sprague SJ, et al. MYC2 differentially modulates diverse jasmonate-dependent functions in arabidopsis. Plant Cell. 2007;19:2225–22245.10.1105/tpc.106.048017
- Chini A, Fonseca S, Fernández G, et al. The JAZ family of repressors is the missing link in jasmonate signalling. Nature. 2007;448:666–671.10.1038/nature06006
- Thines B, Katsir L, Melotto M, et al. JAZ repressor proteins are targets of the SCF(COI1) complex during jasmonate signalling. Nature. 2007;448:661–665.10.1038/nature05960
- Santner A, Estelle M. The JAZ Proteins link jasmonate perception with transcriptional changes. Plant Cell. 2008;19:3835–3842.
- Sheard LB, Tan X, Mao H, et al. Jasmonate perception by inositol-phosphate-potentiated COI1-JAZ co-receptor. Nature. 2010;468:400–405.10.1038/nature09430
- Fonseca S, Chini A, Hamberg M, et al. (+)-7-iso-Jasmonoyl-L-isoleucine is the endogenous bioactive jasmonate. Nat Chem Biol. 2009;5:344–350.10.1038/nchembio.161
- Monte I, Hamberg M, Chini A, et al. Rational design of a ligand-based antagonist of jasmonate perception. Nat Chem Biol. 2014;10:671–676.10.1038/nchembio.1575
- Zhang L, Yao J, Withers J, et al. Host target modification as a strategy to counter pathogen hijacking of the jasmonate hormone receptor. Proc. Natl. Acad. Sci. 2015;12:14354–14359.10.1073/pnas.1510745112
- MSA NM. Miraculous role of salicylic acid in plant and animal system. Am J Plant Physiol. 2007;2:51–58.
- Dong X. SA, JA, ethylene, and disease resistance in plants. Curr Opin Plant Biol. 1998;1:316–323.10.1016/1369-5266(88)80053-0
- Durrant WE, Dong X. Systemic Acquired Resistance. Annu Rev Phytopathol. 2004;42:185–209.10.1146/annurev.phyto.42.040803.140421
- Heil M, Ton J. Long-distance signalling in plant defence. Trends Plant Sci. 2008;13:264–272.10.1016/j.tplants.2008.03.005
- Vlot AC, Dempsey DA, Klessig DF. Salicylic acid, a multifaceted hormone to combat disease. Annu Rev Phytopathol. 2009;47:177–206.10.1146/annurev.phyto.050908.135202
- Boatwright JL, Pajerowska-Mukhtar K. Salicylic acid: an old hormone up to new tricks. Mol Plant Pathol. 2013;14:623–634.10.1111/mpp.2013.14.issue-6
- Shah J, Chaturvedi R, Chowdhury Z, et al. Signaling by small metabolites in systemic acquired resistance. Plant J. 2014;79:645–658.10.1111/tpj.2014.79.issue-4
- Rivas-San Vicente M, Plasencia J. Salicylic acid beyond defence: its role in plant growth and development. J Exp Bot. 2011;62:3321–3338.10.1093/jxb/err031
- Yuan S, Lin H-H. Role of salicylic acid in plant abiotic stress. Z. Naturforsch. C. 2008;63:313–320.
- Miura K, Tada Y. Regulation of water, salinity, and cold stress responses by salicylic acid. Front Plant Sci. 2014;5:4.
- Khan MIR, Fatma M, Per TS, et al. Salicylic acid-induced abiotic stress tolerance and underlying mechanisms in plants. Front Plant Sci. 2015;6:462.
- Liu Z, Ding Y, Wang F, et al. Role of salicylic acid in resistance to cadmium stress in plants. Plant Cell Rep. 2016;35:719–731.10.1007/s00299-015-1925-3
- Yoshimoto K. Plant autophagy puts the brakes on cell death by controlling salicylic acid signaling. Autophagy. 2010;6:192–193.10.4161/auto.6.1.10843
- Yoshimoto K, Jikumaru Y, Kamiya Y, et al. Autophagy negatively regulates cell death by controlling NPR1-dependent salicylic acid signaling during senescence and the innate immune response in arabidopsis. Plant cell. 2009;21:2914–2927.10.1105/tpc.109.068635
- Lenz HD, Haller E, Melzer E, et al. Autophagy differentially controls plant basal immunity to biotrophic and necrotrophic pathogens. Plant J. 2011;66:818–830.10.1111/j.1365-313X.2011.04546.x
- Chen Z, Zheng Z, Huang J, et al. Biosynthesis of salicylic acid in plants. Plant Signal Behav. 2009;4:493–496.10.4161/psb.4.6.8392
- Bandurska H, Niedziela J, Chadzinikolau T. Separate and combined responses to water deficit and UV-B radiation. Plant Sci. 2013;213:98–105.10.1016/j.plantsci.2013.09.003
- Wildermuth MC, Dewdney J, Wu G, et al. Isochorismate synthase is required to synthesize salicylic acid for plant defence. Nature. 2001;414:562–565.10.1038/35107108
- Dempsey DA, Vlot AC, Wildermuth MC, et al. Salicylic acid biosynthesis and metabolism. Arab B. 2011;9:e0156.10.1199/Tab.0156
- Yalpani N, Raskin I. Salicylic acid: a systemic signal in induced plant disease resistance. Trends Microbiol. 1993;1:88–92.10.1016/0966-842X(93)90113-6
- León J, Shulaev V, Yalpani N, et al. Benzoic acid 2-hydroxylase, a soluble oxygenase from tobacco, catalyzes salicylic acid biosynthesis. Proc Natl Acad Sci U S A. 1995;92:10413–10417.10.1073/pnas.92.22.10413
- Silverman P, Seskar M, Kanter D, et al. Salicylic scid in rice (biosynthesis, conjugation, and possible role). Plant Physiol. 1995;108:633–639.10.1104/pp.108.2.633
- Coquoz J-L, Buchala A, Metraux J-P. The biosynthesis of salicylic acid in potato plants. Plant Physiol. 1998;117:1095–1101.10.1104/pp.117.3.1095
- Garcion C, Lohmann A, Lamodière E, et al. Characterization and biological function of the ISOCHORISMATE SYNTHASE2 gene of arabidopsis. Plant Physiol. 2008;147:1279–1287.10.1104/pp.108.119420
- Pallas JA, Paiva NL, Lamb C, et al. Tobacco plants epigenetically suppressed in phenylalanine ammonia-lyase expression do not develop systemic acquired resistance in response to infection by tobacco mosaic virus. Plant J. 1996;10:281–293.10.1046/j.1365-313X.1996.10020281.x
- Howles PA, Sewalt V, Paiva NL, et al. Overexpression of L-phenylalanine ammonia-lyase in transgenic tobacco plants reveals control points for flux into phenylpropanoid biosynthesis. Plant Physiol. 1996;112:1617–1624.10.1104/pp.112.4.1617
- Meuwly P, Molders W, Buchala A, et al. Local and systemic biosynthesis of salicylic acid in infected cucumber plants. Plant Physiol. 1995;109:1107–1114.10.1104/pp.109.3.1107
- Seskar M, Shulaev V, Raskin I. Endogenous methyl salicylate in pathogen-inoculated tobacco plants. Plant Physiol. 1998;116:387–392.10.1104/pp.116.1.387
- Dudareva N, Raguso RA, Wang J, et al. Floral scent production in Clarkia breweri. III. Enzymatic synthesis and emission of benzenoid esters. Plant Physiol. 1998;116:599–604.10.1104/pp.116.2.599
- Park S-W, Liu P-P, Forouhar F, et al. Use of a synthetic salicylic acid analog to investigate the roles of methyl salicylate and its esterases in plant disease resistance. J Biol Chem. 2009;284:7307–7317.10.1074/jbc.M807968200
- Dong X. NPR1, all things considered. Curr Opin Plant Biol. 2004;7:547–552.10.1016/j.pbi.2004.07.005
- Fu ZQ, Dong X. Systemic acquired resistance: turning local infection into global defense. Annu Rev Plant Biol. 2013;64:839–863.10.1146/annurev-arplant-042811-105606
- Fu ZQ, Yan S, Saleh A, et al. NPR3 and NPR4 are receptors for the immune signal salicylic acid in plants. Nature. 2012;486:228–232.
- Wu Y, Zhang D, Chu JY, et al. The arabidopsis NPR1 protein is a receptor for the plant defense hormone salicylic acid. Cell Rep. 2012;1:639–647.10.1016/j.celrep.2012.05.008
- Friedrich L, Lawton K, Ruess W, et al. A benzothiadiazole derivative induces systemic acquired resistance in tobacco. Plant J. 1996;10:61–70.10.1046/j.1365-313X.1996.10010061.x
- Görlach J, Volrath S, Knauf-Beiter G, et al. Benzothiadiazole, a novel class of inducers of systemic acquired resistance, activates gene expression and disease resistance in wheat. Plant Cell. 1996;8:629–643.10.1105/tpc.8.4.629
- Lawton KA, Friedrich L, Hunt M, et al. Benzothiadiazole induces disease resistance in Arabidopsis by activation of the systemic acquired resistance signal transduction pathway. Plant J. 1996;10:71–82.10.1046/j.1365-313X.1996.10010071.x
- Yoshioka K, Nakashita H, Klessig DF, et al. Probenazole induces systemic acquired resistance in Arabidopsis with a novel type of action. Plant J. 2001;25:149–157.10.1046/j.1365-313x.2001.00952.x
- Nakashita H, Yoshioka K, Yasuda M, et al. Probenazole induces systemic acquired resistance in tobacco through salicylic acid accumulation. Physiol Mol Plant Pathol. 2002;61:197–203.10.1006/pmpp.2002.0426
- Lebel E, Heifetz P, Thorne L, et al. Functional analysis of regulatory sequences controlling PR-1 gene expression in Arabidopsis. Plant J. 1998;16:223–233.10.1046/j.1365-313x.1998.00288.x
- Kesarwani M, Yoo J, Dong X. Genetic interactions of TGA transcription factors in the regulation of pathogenesis-related genes and disease resistance in arabidopsis. Plant Physiol. 2007;144:336–346.10.1104/pp.106.095299
- Fitzgerald HA, Chern M-S, Navarre R, et al. Overexpression of (At) NPR1 in rice leads to a BTH- and environment-induced lesion-mimic/cell death phenotype. Mol Plant-Microbe Interact. 2004;17:140–151.10.1094/MPMI.2004.17.2.140
- Bai W, Chern M, Ruan D, et al. Enhanced disease resistance and hypersensitivity to BTH by introduction of an NH1/OsNPR1 paralog. Plant Biotechnol J. 2011;9:205–215.10.1111/pbi.2010.9.issue-2
- Yasuda M, Nakashita H, Hasegawa S, et al. N -Cyanomethyl-2-chloroisonicotinamide induces systemic acquired resistance in arabidopsis without salicylic acid accumulation. Biosci Biotechnol Biochem. 2003;67:322–328.10.1271/bbb.67.322
- Seo E-K, Nakamura H, Mori M, et al. Screening and characterization of a chemical regulator for plant disease resistance. Bioorg Med Chem Lett. 2012;22:1761–1765.10.1016/j.bmcl.2011.12.082
- Jiang K, Kurimoto T, Seo E, et al. Development of inhibitors of salicylic acid signaling. J Agric Food Chem. 2015;63:7124–7133.10.1021/acs.jafc.5b01521
- Noutoshi Y, Okazaki M, Shirasu K. Imprimatins A and B. Plant Signal Behav. 2012;7:1715–1717.10.4161/psb.22368
- Noutoshi Y, Okazaki M, Kida T, et al. Novel plant immune-priming compounds identified via high-throughput chemical screening target salicylic acid glucosyltransferases in arabidopsis. Plant Cell. 2012;24:3795–3804.10.1105/tpc.112.098343
- Noutoshi Y, Jikumaru Y, Kamiya Y, et al. ImprimatinC1, a novel plant immune-priming compound, functions as a partial agonist of salicylic acid. Sci Rep. 2012;2:705.10.1038/srep00705
- Noutoshi Y, Ikeda M, Shirasu K, et al. Diuretics prime plant immunity in arabidopsis thaliana. PLoS One. 2012;7:e48443.10.1371/journal.pone.0048443
- Noutoshi Y, Ikeda M, Saito T, et al. Sulfonamides identified as plant immune-priming compounds in high-throughput chemical screening increase disease resistance in Arabidopsis thaliana. Front Plant Sci. 2012;3:245.
- Cook CE, Whichard LP, Turner B, et al. Germination of witchweed (Striga lutea Lour.): isolation and properties of a potent stimulant. Science. 1966;154:1189–1190.10.1126/science.154.3753.1189
- Akiyama K, Matsuzaki K, Hayashi H. Plant sesquiterpenes induce hyphal branching in arbuscular mycorrhizal fungi. Nature. 2005;435:824–827.10.1038/nature03608
- Umehara M, Hanada A, Yoshida S, et al. Inhibition of shoot branching by new terpenoid plant hormones. Nature. 2008;455:195–200.10.1038/nature07272
- Gomez-Roldan V, Fermas S, Brewer PB, et al. Strigolactone inhibition of shoot branching. Nature. 2008;455:189–194.10.1038/nature07271
- Kapulnik Y, Delaux P-M, Resnick N, et al. Strigolactones affect lateral root formation and root-hair elongation in Arabidopsis. Planta. 2011;233:209–216.10.1007/s00425-010-1310-y
- Sun H, Tao J, Hou M, et al. A strigolactone signal is required for adventitious root formation in rice. Ann Bot. 2015;115:1155–1162.10.1093/aob/mcv052
- Agusti J, Herold S, Schwarz M, et al. Strigolactone signaling is required for auxin-dependent stimulation of secondary growth in plants. Proc Natl Acad Sci U S A. 2011;108:20242–20247.10.1073/pnas.1111902108
- Yamada Y, Umehara M. Possible roles of strigolactones during leaf senescence. Plants. 2015;4:664–677.10.3390/plants4030664
- Toh S, Kamiya Y, Kawakami N, et al. Thermoinhibition uncovers a role for strigolactones in arabidopsis seed germination. Plant Cell Physiol. 2012;53:107–117.10.1093/pcp/pcr176
- Li GD, Pan LN, Jiang K, et al. Strigolactones are involved in sugar signaling to modulate early seedling development in Arabidopsis. Plant Biotechnol. 2016;33:87–97.10.5511/plantbiotechnology.16.0326a
- Zwanenburg B, Mwakaboko AS, Kannan C. Suicidal germination for parasitic weed control. Pest Manag Sci. 2016;72:2016–2025.10.1002/ps.2016.72.issue-11
- Akiyama K, Hayashi H. Strigolactones: chemical signals for fungal symbionts and parasitic weeds in plant roots. Ann Bont. 2006;97:925–931.10.1093/aob/mcl063
- Brewer PB, Koltai H, Beveridge CA. Diverse roles of strigolactones in plant development. Mol Plant. 2013;6:18–28.10.1093/mp/sss130
- Yoneyama K, Xie X, Yoneyama K, et al. Strigolactones: structures and biological activities. Pest Manag Sci. 2009;65:467–470.10.1002/ps.v65:5
- Johnson AW, Gowada G, Hassanali A, et al. The preparation of synthetic analogues of strigol. J Chem Soc Perkin Trans. 1981;1:1734–1743.10.1039/p19810001734
- Mangnus EM, Dommerholt FJ, De Jong RLP, et al. Improved synthesis of strigol analog GR24 and evaluation of the biological activity of its diastereomers. J Agric Food Chem. 1992;40:1230–1235.10.1021/jf00019a031
- Mangnus EM, Van Vliet LA, Vandenput DAL, et al. Structural modifications of strigol analogs. Influence of the B and C rings on the bioactivity of the germination stimulant GR24. J Agric Food Chem. 1992;40:1222–1229.10.1021/jf00019a030
- Nefkens Gérard H L, Jan Willem J, Thuring F, Beenakkers Marco F M, et al. Synthesis of a phthaloylglycine-derived strigol analogue and its germination stimulatory activity toward seeds of the parasitic weeds Striga hermonthica and Orobanche crenata. 1997;45:2273–2277.
- Jan Willem J, Thuring F, Bitter Harry H, de Kok Margreet M, et al. N-phthaloylglycine-derived strigol analogues. Influence of the D-ring on seed germination activity of the parasitic weeds Striga hermonthica and Orobanche crenata. 1997;45:2284–2290.
- Mwakaboko AS, Zwanenburg B. Strigolactone analogs derived from ketones using a working model for germination stimulants as a blueprint. Plant Cell Physiol. 2011;52:699–715.10.1093/pcp/pcr031
- Zwanenburg B, Mwakaboko AS. Strigolactone analogues and mimics derived from phthalimide, saccharine, p-tolylmalondialdehyde, benzoic and salicylic acid as scaffolds. Bioorg Med Chem. 2011;19:7394–7400.10.1016/j.bmc.2011.10.057
- Fukui K, Ito S, Ueno K, et al. New branching inhibitors and their potential as strigolactone mimics in rice. Bioorg Med Chem Lett. 2011;21:4905–4908.10.1016/j.bmcl.2011.06.019
- Fukui K, Ito S, Asami T. Selective mimics of strigolactone actions and their potential use for controlling damage caused by root parasitic weeds. Mol Plant. 2013;6:88–99.10.1093/mp/sss138
- Takahashi I, Fukui K, Asami T. Chemical modification of a phenoxyfuranone-type strigolactone mimic for selective effects on rice tillering or Striga hermonthica seed germination. Pest Manag Sci. 2016;72:2048–2053.10.1002/ps.2016.72.issue-11
- Boyer F-D, de Saint Germain A, Pillot J-P, et al. Structure-activity relationship studies of strigolactone-related molecules for branching inhibition in garden pea: molecule design for shoot branching. Plant Physiol. 2012;159:1524–1544.10.1104/pp.112.195826
- Alder A, Jamil M, Marzorati M, et al. The path from β-carotene to carlactone, a strigolactone-like plant hormone. Science. 2012;335:1348–1351.10.1126/science.1218094
- Abe S, Sado A, Tanaka K, et al. Carlactone is converted to carlactonoic acid by MAX1 in Arabidopsis and its methyl ester can directly interact with AtD14 in vitro. Proc Natl Acad Sci U S A. 2014;111:18084–18089.10.1073/pnas.1410801111
- Brewer PB, Yoneyama K, Filardo F, et al. LATERAL BRANCHING OXIDOREDUCTASE acts in the final stages of strigolactone biosynthesis in Arabidopsis. Proc Natl Acad Sci U S A. 2016;113:6301–6306.10.1073/pnas.1601729113
- Ueno K, Furumoto T, Umeda S, et al. Heliolactone, a non-sesquiterpene lactone germination stimulant for root parasitic weeds from sunflower. Phytochemistry. 2014;108:122–128.10.1016/j.phytochem.2014.09.018
- Jia K-P, Kountche BA, Jamil M, et al. Nitro-phenlactone, a carlactone analog with pleiotropic strigolactone activities. Mol Plant. 2016;9:1341–1344.10.1016/j.molp.2016.05.017
- Boyer F-D, de Saint Germain A, Pouvreau J-B, et al. New strigolactone analogs as plant hormones with low activities in the rhizosphere. Mol Plant. 2014;7:675–690.10.1093/mp/sst163
- Pereira RG, Cala A, Fernández-Aparicio M, et al. Gibberellic and kaurenoic hybrid strigolactone mimics for seed germination of parasitic weeds. Pest Manag Sci. 2017;73:2529–2537.10.1002/ps.2017.73.issue-12
- Cala A, Ghooray K, Fernández-Aparicio M, et al. Phthalimide-derived strigolactone mimics as germinating agents for seeds of parasitic weeds. Pest Manag Sci. 2016;72:2069–2081.10.1002/ps.2016.72.issue-11
- Dvorakova M, Soudek P, Vanek T. Triazolide strigolactone mimics influence root development in arabidopsis. J Nat Prod. 2017;80:1318–1327.10.1021/acs.jnatprod.6b00879
- Tsuchiya Y, Vidaurre D, Toh S, et al. A small-molecule screen identifies new functions for the plant hormone strigolactone. Nat Chem Biol. 2010;6:741–749.10.1038/nchembio.435
- Toh S, Holbrook-Smith D, Stogios PJ, et al. Structure-function analysis identifies highly sensitive strigolactone receptors in Striga. Science. 2015;350:203–207.10.1126/science.aac9476
- Lin H, Wang R, Qian Q, et al. DWARF27, an iron-containing protein required for the biosynthesis of strigolactones, regulates rice tiller bud outgrowth. Plant Cell. 2009;21:1512–1525.10.1105/tpc.109.065987
- Waters MT, Brewer PB, Bussell JD, et al. The arabidopsis ortholog of Rice DWARF27 Acts Upstream of MAX1 in the control of plant development by strigolactones. Plant Physiol. 2012;159:1073–1085.10.1104/pp.112.196253
- Zhang Y, van Dijk ADJ, Scaffidi A, et al. Rice cytochrome P450 MAX1 homologs catalyze distinct steps in strigolactone biosynthesis. Nat Chem Biol. 2014;10:1028–1033.10.1038/nchembio.1660
- Nakamura H, Asami T. Target sites for chemical regulation of strigolactone signaling. Front Plant Sci. 2014;5:623.
- Kitahata N, Asami T. Chemical biology of abscisic acid. J Plant Res. 2011;124:549–557.10.1007/s10265-011-0415-0
- Ito S, Kitahata N, Umehara M, et al. A new lead chemical for strigolactone biosynthesis inhibitors. Plant Cell Physiol. 2010;51:1143–1150.10.1093/pcp/pcq077
- Ito S, Umehara M, Hanada A, et al. Effects of triazole derivatives on strigolactone levels and growth retardation in rice. PLoS One. 2011;6:e21723.10.1371/journal.pone.0021723
- Ito S, Umehara M, Hanada A, et al. Effects of strigolactone-biosynthesis inhibitor TIS108 on Arabidopsis. Plant Signal Behav. 2013;8:e24193.10.4161/psb.24193
- Ito S, Umehara M, Hanada A, et al. Tebuconazole derivatives are potent inhibitors of strigolactone biosynthesis. J Pestic Sci. 2013;38:147–151.10.1584/jpestics.D13-011
- Sergeant MJ, Li J-J, Fox C, et al. Selective inhibition of carotenoid cleavage dioxygenases. J Biol Chem. 2009;284:5257–5264.10.1074/jbc.M805453200
- Ito S, Yamagami D, Umehara M, et al. Regulation of strigolactone biosynthesis by gibberellin signaling. Plant Physiol. 2017;174:1250–1259.10.1104/pp.17.00301
- Arite T, Umehara M, Ishikawa S, et al. d14, a strigolactone-insensitive mutant of rice, shows an accelerated outgrowth of tillers. Plant Cell Physiol. 2009;50:1416–1424.10.1093/pcp/pcp091
- Gao Z, Qian Q, Liu X, et al. Dwarf 88, a novel putative esterase gene affecting architecture of rice plant. Plant Mol Biol. 2009;71:265–276.10.1007/s11103-009-9522-x
- Liu W, Wu C, Fu Y, et al. Identification and characterization of HTD2: a novel gene negatively regulating tiller bud outgrowth in rice. Planta. 2009;230:649–658.10.1007/s00425-009-0975-6
- Gaiji N, Cardinale F, Prandi C, et al. The computational-based structure of Dwarf14 provides evidence for its role as potential strigolactone receptor in plants. BMC Res Notes. 2012;5:307.10.1186/1756-0500-5-307
- Hamiaux C, Drummond RSM, Janssen BJ, et al. DAD2 Is an α/β hydrolase likely to be involved in the perception of the plant branching hormone, strigolactone. Curr Biol. 2012;22:2032–2036.10.1016/j.cub.2012.08.007
- Nakamura H, Xue Y-L, Miyakawa T, et al. Molecular mechanism of strigolactone perception by DWARF14. Nat Commun. 2013;4:2613.
- Kagiyama M, Hirano Y, Mori T, et al. Structures of D14 and D14L in the strigolactone and karrikin signaling pathways. Genes Cells. 2013;18:147–160.10.1111/gtc.2013.18.issue-2
- Zhao L-H, Zhou XE, Wu Z-S, et al. Crystal structures of two phytohormone signal-transducing α/β hydrolases: karrikin-signaling KAI2 and strigolactone-signaling DWARF14. Cell Res. 2013;23:436–439.10.1038/cr.2013.19
- Zhao L-H, Zhou XE, Yi W, et al. Destabilization of strigolactone receptor DWARF14 by binding of ligand and E3-ligase signaling effector DWARF3. Cell Res. 2015;25:1219–1236.10.1038/cr.2015.122
- Jiang L, Liu X, Xiong G, et al. DWARF 53 acts as a repressor of strigolactone signalling in rice. Nature. 2013;504:401–405.10.1038/nature12870
- Zhou F, Lin Q, Zhu L, et al. D14–SCFD3-dependent degradation of D53 regulates strigolactone signalling. Nature. 2013;504:406–410.10.1038/nature12878
- Wang L, Wang B, Jiang L, et al. Strigolactone signaling in arabidopsis regulates shoot development by targeting D53-like SMXL repressor proteins for ubiquitination and degradation. Plant Cell. 2015;27:3128–3142.10.1105/tpc.15.00605
- Soundappan I, Bennett T, Morffy N, et al. SMAX1-LIKE/D53 family members enable distinct MAX2-dependent responses to strigolactones and karrikins in arabidopsis. Plant Cell. 2015;27:3143–3159.10.1105/tpc.15.00562
- Liu Q, Zhang Y, Matusova R, et al. Striga hermonthica MAX2 restores branching but not the Very Low Fluence Response in the Arabidopsis thaliana max2 mutant. New Phytol. 2014;202:531–541.10.1111/nph.12692
- Conn CE, Bythell-Douglas R, Neumann D, et al. Convergent evolution of strigolactone perception enabled host detection in parasitic plants. Science. 2015;349:540–543.10.1126/science.aab1140
- Yao R, Ming Z, Yan L, et al. DWARF14 is a non-canonical hormone receptor for strigolactone. Nature. 2016;536:469–473.
- Fukui K, Yamagami D, Ito S, et al. A taylor-made design of phenoxyfuranone-type strigolactone mimic. Front Plant Sci. 2017;8:936.10.3389/fpls.2017.00936
- Tsuchiya Y, Yoshimura M, Sato Y, et al. Parasitic plants. Probing strigolactone receptors in Striga hermonthica with fluorescence. Science. 2015;349:864–868.10.1126/science.aab3831
- de Saint Germain A, Clavé G, Badet-Denisot M-A, et al. An histidine covalent receptor and butenolide complex mediates strigolactone perception. Nat Chem Biol. 2016;12:787–794.10.1038/nchembio.2147
- Holbrook-Smith D, Toh S, Tsuchiya Y, et al. Small-molecule antagonists of germination of the parasitic plant Striga hermonthica. Nat Chem Biol. 2016;12:724–729.10.1038/nchembio.2129
- Xiang H, Yao R, Quan T, et al. Simple β-lactones are potent irreversible antagonists for strigolactone receptors. Cell Res. 2017;25:1525–1528.10.1038/cr.2017.105
- Mashita O, Koishihara H, Fukui K, et al. Discovery and identification of 2-methoxy-1-naphthaldehyde as a novel strigolactone-signaling inhibitor. J Pestic Sci. 2016;41:71–78.10.1584/jpestics.D16-028
- Yao R, Wang F, Ming Z, et al. ShHTL7 is a non-canonical receptor for strigolactones in root parasitic weeds. Cell Res. 2017;27:838–841.10.1038/cr.2017.3
- Shannon DA, Weerapana E. Covalent protein modification: the current landscape of residue-specific electrophiles. Curr Opin Chem Biol. 2015;24:18–26.10.1016/j.cbpa.2014.10.021
- Waters MT, Scaffidi A, Flematti GR, et al. The origins and mechanisms of karrikin signalling. Curr Opin Plant Biol. 2013;16:667–673.10.1016/j.pbi.2013.07.005
- Smith SM, Li J. Signalling and responses to strigolactones and karrikins. Curr Opin Plant Biol. 2014;21:23–29.10.1016/j.pbi.2014.06.003
- De Cuyper C, Struk S, Braem L, et al. Strigolactones, karrikins and beyond. Plant Cell Environ. 2017;40:1691–1703.10.1111/pce.v40.9
- Stanga JP, Smith SM, Briggs WR, et al. SUPPRESSOR OF MORE AXILLARY GROWTH2 1 controls seed germination and seedling development in arabidopsis. Plant Physiol. 2013;163:318–330.10.1104/pp.113.221259
- Morffy N, Faure L, Nelson DC. Smoke and hormone mirrors: action and evolution of karrikin and strigolactone signaling. Trends Genet. 2016;32:176–188.10.1016/j.tig.2016.01.002
- Conn CE, Nelson DC. Evidence that KARRIKIN-INSENSITIVE2 (KAI2) receptors may perceive an unknown signal that is not karrikin or strigolactone. Front Plant Sci. 2016;6:1219.
- Hicks GR, Raikhel NV. Small molecules present large opportunities in plant biology. Annu Rev Plant Biol. 2012;63:261–282.10.1146/annurev-arplant-042811-105456
- Hicks GR, Raikhel NV. Plant chemical biology: are we meeting the promise? Front Plant Sci. 2014;5:455.
- Dejonghe W, Russinova E. Plant chemical genetics: from phenotype-based screens to synthetic biology. Plant Physiol. 2017;174:5–20.10.1104/pp.16.01805
- Nobelprize.org. Nobel Media AB 2014. The 2017 Nobel Prize in Chemistry - Press Release. Nobel Prize. 2017.
- Wang H-W, Lei J, Shi Y. Biological cryo-electron microscopy in China. Protein Sci. 2017;26:16–31.10.1002/pro.v26.1
- Jonić S. Cryo-electron microscopy analysis of structurally heterogeneous macromolecular complexes. Comput Struct Biotechnol J. 2016;14:385–390.
- Merino F, Raunser S. Electron cryo-microscopy as a tool for structure-based drug development. Angew Chemie Int Ed. 2017;56:2846–2860.10.1002/anie.201608432
- Chockalingam K, Zhao H. Creating new specific ligand–receptor pairs for transgene regulation. Trends Biotechnol. 2005;23:333–335.10.1016/j.tibtech.2005.05.002
- Shah K. Orthogonal chemical genetic approaches for unraveling signaling pathways. International Union Biochem. Mol Biol Life. 2005;57:397–405.10.1080/15216540500138238
- Mosquna A, Peterson FC, Park S-Y, et al. Potent and selective activation of abscisic acid receptors in vivo by mutational stabilization of their agonist-bound conformation. Proc Natl Acad Sci U S A. 2011;108:20838–20843.10.1073/pnas.1112838108
- Miyakawa T, Tanokura M. Structural basis for the regulation of phytohormone receptors. Biosci Biotechnol Biochem. 2017;81:1261–1273.10.1080/09168451.2017.1313696
- Shimojo E, Yamaguchi I, Murofushi N. Increase of indole-3-acetic acid in human esophagea cancer tissue. Proc Japan Acad Ser B Phys Biol Sci. 1997;73:182–185.10.2183/pjab.73.182
- Wardman P. Indole-3-acetic acids and horseradish peroxidase: a new prodrug/enzyme combination for targeted cancer therapy. Curr Pharm Des. 2002;8:1363–1374.10.2174/1381612023394610
- Folkes LK, Wardman P. Enhancing the efficacy of photodynamic cancer therapy by radicals from plant auxin (indole-3-acetic acid). Cancer Res. 2003;63:776–779.
- Choi HW, Tian M, Song F, et al. Aspirin’s active metabolite salicylic acid targets high mobility group box 1 to modulate inflammatory responses. Mol Med. 2015;21:526–535.
- Bruzzone S, Moreschi I, Usai C, et al. Abscisic acid is an endogenous cytokine in human granulocytes with cyclic ADP-ribose as second messenger. Proc Natl Acad Sci U S A. 2007;104:5759–5764.10.1073/pnas.0609379104
- Zocchi E, Hontecillas R, Leber A, et al. Abscisic acid: a novel nutraceutical for glycemic control. Front Nutr. 2017;4:24.10.3389/fnut.2017.00024
- Flescher E. Jasmonates in cancer therapy. Cancer Lett. 2007;245:1–10.10.1016/j.canlet.2006.03.001
- Russo A, Espinoza CL, Caggia S, et al. A new jasmonic acid stereoisomeric derivative induces apoptosis via reactive oxygen species in human prostate cancer cells. Cancer Lett. 2012;326:199–205.10.1016/j.canlet.2012.08.025
- Zhang M, Zhang MW, Zhang L, et al. Methyl jasmonate and its potential in cancer therapy. Plant Signal Behav. 2015;10:e1062199.10.1080/15592324.2015.1062199
- Pollock CB, Koltai H, Kapulnik Y, et al. Strigolactones: a novel class of phytohormones that inhibit the growth and survival of breast cancer cells and breast cancer stem-like enriched mammosphere cells. Breast Cancer Res Treat. 2012;134:1041–1055.10.1007/s10549-012-1992-x
- Mayzlish-Gati E, Laufer D, Grivas CF, et al. Strigolactone analogs act as new anti-cancer agents in inhibition of breast cancer in xenograft model. Cancer Biol Ther. 2015;16:1682–1688.10.1080/15384047.2015.1070982