ABSTRACT
In the present study, we purified and characterized three formaldehyde dismutases (Fdms) (EC 1.2.98.1) (Fdm1, Fdm2, and Fdm3) of Methylobacterium sp. FD1. These Fdms (with His-tag) were produced in the recombinant E. coli and purified by immobilized metal affinity chromatography from the E. coli extracts. In each of the three Fdms, the enzyme-bound coenzyme was nicotinamide adenine dinucleotide (NAD(H)) and the enzyme-bound metal was zinc. The quaternary structures of these Fdms were estimated as homotetrameric. The optimal pHs and temperatures of Fdm1, Fdm2, and Fdm3 were approximately 6.5, 6.0, and 6.0, and 35°C, 25°C, and 30°C, respectively. The Km values of Fdm1, Fdm2, and Fdm3 were 621, 865, and 414 mM, respectively. These results were similar to the properties of already-known Fdms. However, each of the Fdms of FD1 had methanol:p-nitroso-N,N-dimethylaniline oxidoreductase activity that is not found in already-known Fdms.
GRAPHICAL ABSTRACT
Formaldehyde dismutases with methanol: p-nitroso-N,N-dimethylaniline oxidoreductase activity of Methylobacterium sp. FD1.
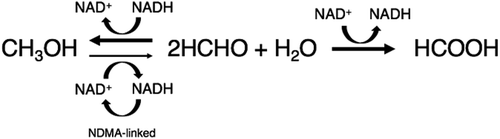
Formaldehyde (HCHO) is widely used as a raw material in a variety of chemical products, such as resins, as well as a preservative for cosmetics, detergents, and human cadavers and animal organs for research. Degradation of formaldehyde is necessary to prevent environmental pollution. Although a number of technologies are available to remove or degrade formaldehyde in liquid pollution, biological methods are desirable that are environmentally friendly. In our preceding paper [Citation1]), we described the isolation of a formaldehyde-degrading bacterium, Methylobacterium sp. FD1, from soil; the resting cells of FD1 degraded high concentrations of formaldehyde (< 2.7 M) and produced formic acid and methanol in molar equivalents of one-half of the degraded formaldehyde. These results suggested that the formaldehyde degradation enzyme of FD1 was formaldehyde dismutase (Fdm) (EC 1.2.98.1). Fdm is known to catalyze the dismutation of formaldehyde in a ping-pong mechanism without the addition of coenzymes because the enzyme is a nicotinoprotein that contains nicotinamide adenine dinucleotide (NAD(H)) as a tightly bound, redox-active cofactor [Citation2]). For the industrial use of Fdm or the bacterial cells producing the enzyme, those that do not require an expensive coenzyme for the degradation of formaldehyde of high concentration, is desirable. Fdm genes appear to be widely spread through bacteria, based on various genomic sequence data [Citation3]). However, Fdm genes have been cloned only from Pseudomonas putida F61 [Citation4]), P. putida J3 [Citation5]), and Methylobacterium sp. FD1 [Citation3]). Fdms have been purified from and characterized in only P. putida F61 [Citation6–Citation8]) and P. putida J3 [Citation9]). Recently, we sequenced the FD1 genome (unpublished data) and found two more Fdm genes (named fdm2 and fdm3) in addition to the Fdm gene that we cloned previously (fdm1) [Citation3]).
In the present study, we purified and characterized Fdm1, Fdm2, and Fdm3 of FD1 from the recombinant E. coli corresponding to each gene and found that these Fdms have methanol:p-nitroso-N,N-dimethylaniline (NDMA) oxidoreductase (EC 1.1.99.37) activity.
Materials and methods
Chemicals
Formaldehyde solution (16% [w/v], methanol-free) was purchased from Thermo Fisher Scientific (Rockford, IL, USA). Formaldehyde solution (37% [w/v], containing 5-10% [w/v] methanol), kanamycin sulfate, chloramphenicol, rhamnose monohydrate, arabinose, and acetylacetone were purchased from Wako Pure Chemical Industries (Osaka, Japan). p-Nitroso-N,N-dimethylaniline (NDMA) was purchased from Tokyo Chemical Industry (Tokyo, Japan). NAD+ and NADH were purchased from Oriental Yeast (Tokyo, Japan). Bacto™ tryptic soy broth (soybean-casein digest medium) and Bacto™ tryptone (enzymatic digest of casein) were purchased from Becton, Dickinson and Company (Sparks, MD, USA). Yeast extract was purchased from Life Technologies (Paisley, Scotland).
Bacterial strains and plasmid
Methylobacterium sp. FD1 [Citation1]) was used for the genomic DNA extraction. Expresso® Rhamnose Cloning and Expression System (Lucigen, Middleton, WI, USA) containing E. coli (strain E. cloni® 10 G) and a plasmid (pRham™ C-His Kan vector with L-rhamnose-inducible rhaPBAD promoter [Citation10])) was used for the expression of the heterologous protein. pGro7 (Takara, Shiga, Japan), containing chaperone (GroESL) genes and a chloramphenicol resistant gene, was used for chaperone coexpression in E. coli.
Cloning of formaldehyde dismutase genes and expression in E. coli
Extraction of the genomic DNA from Methylobacterium sp. FD1 grown in tryptic soy broth and the polymerase chain reaction (PCR) amplification of formaldehyde dismutase genes of FD1 (attached 6× His-tag sequence at 3ʹ terminal) were described previously [Citation3]). The primers used are summarized in . Cloning and expression of the Fdm genes of FD1 in E. coli was conducted according to the manual of Expresso® Rhamnose Cloning and Expression System. pGro7 was introduced into the recombinant E. coli transformed with a Fdm gene. The induction of the expression of Fdm and GroESL genes in E. coli was performed as follows. Recombinant E. coli cells were grown in LB medium (10 g/L tryptone, 5 g/L yeast extract, and 10 g/L NaCl) containing 30 µg/mL kanamycin, 20 µg/mL chloramphenicol, and 0.15% glucose at 37°C under shaking condition for 10 h. Next, the culture was diluted (16:100) and incubated in LB medium (700 mL) containing 30 µg/mL kanamycin, 20 µg/mL chloramphenicol, 0.05% glucose, 0.2% rhamnose, and 0.2% arabinose. The culture was subsequently incubated at 37°C under shaking condition 17 h.
Table 1. Primers for PCR amplification of formaldehyde dismutase genes of FD1.
Purification of formaldehyde dismutase
The recombinant E. coli cells obtained from 700 mL of culture described above were harvested by centrifugation at 10,354 × g at 4°C, rinsed with 50 mL of buffer (50 mM sodium phosphate, 300 mM NaCl, pH 7.0), then suspended in 30 mL of the same buffer. The cells were disrupted with an ultrasonic disrupter (UD-211; TOMY SEIKO, Tokyo, Japan). The sonicated samples were centrifuged at 14,422 × g at 4°C, and the resulting supernatant was obtained as the soluble protein extract. To activate the chaperone (GroESL) in the soluble protein extract, ATP (0.4 mM) and Mg2+ (2.0 mM) were added to the soluble protein extract and the mixture was incubated at 4°C for 30 min.
Fdm was purified from the soluble protein extract by immobilized metal affinity chromatography (IMAC), TALON® Metal Affinity Resin (Takara Bio USA, Inc., Mountain view, CA, USA), according to the manufacturers’ instructions. The volume of the resin was 2 mL. Elution of Fdm from the resin was achieved using 10 mL of the buffer of pH 5.0, 4.5, and 4.0 (50 mM sodium acetate, 300 mM NaCl), respectively, in a step-wise manner. Each eluate was mixed immediately with 3.3 mL of 200 mM sodium phosphate buffer (pH 7.0). The elution was concentrated with ultrafiltration using Amicon® Ultra-4 Centrifugal Filter Ultracel®-30 K (Merck Millipore, Cork, Ireland) as required. Protein was measured using a Protein Assay BCA kit (Wako Pure Chemical Industries, Osaka, Japan). The purity of Fdm was estimated by sodium dodecyl sulfate polyacrylamide gel electrophoresis (SDS-PAGE), as described previously[Citation3]).
Enzyme assays
Formaldehyde dismutase activity: The formaldehyde dismutase activity of Fdm was determined as follows. Fdm was added to 0.5 mL of 100 mM potassium phosphate buffer (pH 7) containing 50 mM formaldehyde (methanol-free), and the mixture was incubated at 30°C. Subsequently, 0.2 mL of 2 M HCl was added to the mixture to stop the reaction. Formaldehyde was determined using Nash’s method [Citation11]). Formic acid and methanol were determined using high-performance liquid chromatography (HPLC) and gas chromatography (GC), respectively, as previously described [Citation1]). Determination of optimal temperature and pH for the formaldehyde dismutase activity was performed as follows. Formaldehyde dismutase activity was assayed in 0.5-mL buffer containing 50 mM formaldehyde (containing methanol). Effects of pH on formaldehyde dismutase activity were investigated in universal buffer (0.04 M H3BO3, 0.04 M H3PO4, 0.04 M CH3COOH that was adjusted to the desired pH, 2.0–9.0, with 2 M NaOH) at 30°C. Effects of temperature on formaldehyde dismutase activity were investigated in 100 mM sodium phosphate (pH 7.0) at different temperatures ranging from 0 to 50°C. After reaction, 0.2 mL of 2 M HCl was added to stop the reaction. Formaldehyde dismutase activity was estimated by measuring the formic acid generated from formaldehyde using HPLC. The reaction velocity (V) of Fdm was determined as follows. Fdm was added in 0.5 mL of 100 mM sodium phosphate buffer (pH 7.0) containing various concentrations of formaldehyde (containing methanol), and the mixture was incubated at 30°C. At the end of incubation, 0.2 mL of 2 M HCl was added to the mixture to stop the reaction. Fdm activity was estimated by measuring the formic acid generated from formaldehyde using HPLC. The velocity was expressed in terms of the amount of formaldehyde degraded (double amount of formic acid generated) by 1-mg protein per minute.
Substrate specificity: The aldehyde dismutase activities of Fdm were assayed as follows. Fdm was added in 0.5 mL of 100 mM sodium phosphate (pH 7.0) containing 50 mM substrate (acetaldehyde, propionaldehyde, butyraldehyde, and valeraldehyde), and the mixture was incubated at 30°C. After incubation, 0.2 mL of 2 M HCl was added to the mixture to stop the reaction. The activities were estimated by measuring the acid generated from aldehyde using HPLC. One unit corresponded to the amount of enzyme which converts 1 µmol of aldehyde in one minute.
Alcohol:p-nitroso-N,N-dimethylaniline (NDMA) (EC 1.1.99.36) oxidoreductase activity: Alcohol:NDMA oxidoreductase activity was assayed according to previous studies [Citation8,Citation12]) as follows. Fdm was added in 1 mL of 10 mM potassium phosphate buffer (pH 7.0) containing 50 mM alcohol and 38 µM of NDMA, and the mixture was incubated at 30°C. After the incubation, decrease of absorbance at 440 nm (A440) of NDMA was measured using a spectrophotometer V-630 (JASCO, Tokyo, Japan).
Determination of coenzyme NAD(H)
The enzyme-bound NAD(H) was extracted from Fdm in the solution desalinated by Amicon® Ultra-4 Centrifugal Filter Ultracel®-30 K (Merck Millipore) with 90% methanol at 0°C for 15 min [Citation8]). The extract was evaporated and dissolved in an appropriate volume of dH2O. NAD+ and NADH in the solution were determined using HPLC at 254 nm and compared with the authentic NAD+ and NADH. The HPLC apparatus was Prominence® (Shimadzu) with a reverse-phase column (Inertsil® ODS-3, 150 mm × 4.6 mm i.d., 5 µL) (GL Science, Tokyo, Japan), pump (LC-20AT, Shimadzu), and UV-Vis detector (SPD-20A, Shimadzu). The mobile phase was 5% methanol in 10 mM potassium phosphate buffer (pH 7.0) with a flow rate of 0.7 mL/min. The column temperature was maintained at 40°C.
Molecular mass determination
The molecular mass of Fdm was determined by size exclusion chromatography (SEC) with a Superdex™ 200 HR 10/30 column (GE Healthcare Biosciences, Piscataway, NJ, USA). The mobile phase was 50 mM sodium phosphate, 150 mM NaCl (pH 7.0) with a flow rate of 0.5 mL/min. Proteins were detected at 280 nm. For comparison, urease (Mr = 480,000) (Jack Bean, Wako), catalase (240,000) (bovine liver, Wako), alcohol dehydrogenase (148,000) (yeast, Oriental Yeast), BSA (66,500) (Wako), and DNaseI (31,000) (bovine pancreas, Wako) were used.
Zinc determination
Fdm was subjected to microwave digestion using ETHOS®-TC (Milestone, Italy) after addition of nitric acid [final concentration 24% (w/w)]. Zinc from the digested Fdm was determined by ICP-MS Agilent 7700 (Agilent Technologies, Santa Clara, CA, USA). Pretreatment and ICP-MS analysis were performed by Shimadzu Techno-Research, Inc. (Kyoto, Japan).
Nucleotide sequence accession numbers
The nucleotide sequences of fdm2 and fdm3 of FD1 were submitted to the DNA Data Bank of Japan under the accession numbers LC521332 and LC521333, respectively.
Results and discussion
Alignment of amino acid sequences of formaldehyde dismutases (Fdms) and glutathione-independent formaldehyde dehydrogenase (Fdh)
shows the alignment of Fdm1, Fdm2, and Fdm3 of FD1, the Fdm of P. putida F61 [Citation4]), and the glutathione-independent formaldehyde dehydrogenase (Fdh) (EC 1.2.1.46) with aldehyde dismutase activity of P. putida C-83 [Citation13]). The NAD(H)-binding residues of the glutathione-independent Fdh of P. putida C-83 [Citation2]) were conserved in all Fdms of FD1, suggesting that one molecule of NAD(H) is bound per enzyme molecule [Citation2,Citation8]). In addition, catalytic and structural zinc-binding residues of the glutathione-independent Fdh of P. putida C-83 [Citation2]) were also conserved in all Fdms of FD1, suggesting that two atoms of zinc are bound per enzyme molecule [Citation2,Citation8]). The identities between each Fdm obtained by ClustalW analysis at the DNA Data Bank of Japan (DDBJ) were 75.8% for Fdm1-Fdm2, 61.1% for Fdm1-Fdm3, 60.6% for Fdm1-F61-Fdm, 54.6% for Fdm2-Fdm3, 60.0% for Fdm2-F61-Fdm, and 49.9% for Fdm3-F61-Fdm.
Purification of Fdms of FD1
His-tagged Fdm1, Fdm2, and Fdm3 of FD1 were obtained as one band on SDS-PAGE in the elution of pH 4.5 on the IMAC (). Yields of Fdm1, Fdm2, and Fdm3 were 23.1%, 13.7%, and 33.2%, respectively ().
Figure 2. SDS-PAGE of Fdm1 (a), Fdm2 (b), and Fdm3 (c) of FD1 purified from each recombinant E. coli by IMAC.
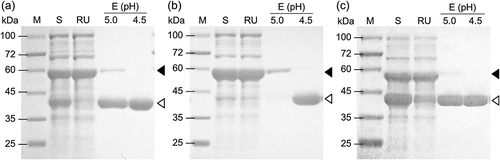
Table 2. Purification of Fdm1, Fdm2, and Fdm3 of FD1.
Enzyme-bound NAD(H)
To confirm whether NAD(H) is bound to the Fdms of FD1, the methanol extracts of the Fdms were anylyzed using HPLC. As a result, NAD+ and NADH from each of these extractions were detected at retention times of 4.2 and 5.7 min, respectively, as with authentic NAD+ and NADH. The amounts of NAD(H) of Fdm1, Fdm2, and Fdm3 were 0.43, 0.97, and 0.81 molecules/molecule-Fdm, respectively. Since the amino acid residues for one molecule of NAD(H) exist in the sequences of each Fdm of FD1, one molecule of NAD(H) may be bound per one enzyme molecule of each Fdm of FD1, as with the Fdm of P. putida F61 [Citation8]).
Enzyme-bound zinc
To confirm whether zinc is bound to the Fdms of FD1, the Fdms were analyzed using ICP-MS. As a result, the amounts of zinc of Fdm1, Fdm2, and Fdm3 were found to be 1.7, 0.71, and 1.2 atoms/molecule-Fdm, respectively. Since the amino acid residues for two atoms of zinc exist in the sequences of each Fdm of FD1, two zinc atoms may be bound per one enzyme molecule of each Fdm, as with the Fdm of P. putida F61 [Citation8]).
Quaternary structure
SEC revealed the molecular mass of Fdm1, Fdm2, and Fdm3 to be 184, 188, and 181 kDa, respectively. From these results, Fdm1, Fdm2, and Fdm3 were estimated to be homotetramers based on the molecular mass of each subunit (43.7, 43.6, and 44.6 kDa).
Formaldehyde dismutase activity
The formaldehyde dismutase activity of each of Fdm2 and Fdm3 was investigated. Fdm2 (2.5 µg) converted 6.9 ± 0.2 µmol of formaldehyde into 3.9 ± 0.3 and 4.1 ± 0.3 µmol of methanol and formic acid, respectively, in 30 min. Fdm3 (12 µg) converted 21 ± 0.9 µmol of formaldehyde into 11 ± 1.3 and 12 ± 0.5 µmol of methanol and formic acid, respectively, in 10 min. These results reveal that Fdm2 and Fdm3 show formaldehyde dismutase activities that can convert formaldehyde into methanol and formic acid that were molar equivalents of approximately one-half of the converted formaldehyde, as with Fdm1 [Citation3]).
Optimal pH
The optimal pH of Fdm1, Fdm2, and Fdm3 of FD1 were approximately 6.5, 6.0, and 6.0, respectively ()). These optimal pHs of the Fdms of FD1 were different from those of the Fdm of P. putida F61 (pH 8.0)[Citation6]) and that of P. putida J3 (pH 8.0) [Citation9]). Fdm3 maintained 80% of the activity for the optimal pH even at pH 3.0. This acid resistance of Fdm3 is not found in Fdm1, Fdm2, the Fdm of P. putida F61 [Citation6]), or the Fdm of P. putida J3 [Citation9]). Fdm3 is useful for industrial use for formaldehyde degradation, since Fdm3 is not easily affected by lowered pH due to formic acid generation from formaldehyde.
Optimal temperature
The optimal temperatures of Fdm1, Fdm2, and Fdm3 were approximately 35, 25, and 30°C, respectively ()), and were lower than those of the Fdm of P. putida F61 (40°C) [Citation6]) and that of P. putida J3 (45°C) [Citation9]).
Kinetic properties
shows Michaelis-Menten curves and Lineweaver-Burk plots of Fdm1, Fdm2, and Fdm3 of FD1 on formaldehyde dismutase activity, and shows the enzyme kinetics parameters of each Fdm. Each Km value of the three Fdms of FD1 was of the same order of magnitude as those of the Fdm of P. putida F61 [Citation6]) and P. putida J3 [Citation9]) (350 mM and 544 mM, respectively). These Km values were several tens times higher than those for the formaldehyde dismutase activity of the methanol:NDMA oxidoreductases (MNOs) (EC 1.1.99.37) of Amycolatopsis methanolica and Mycobacterium gastri (19.5 mM and 12.4 mM, respectively)[Citation14]). Each Vmax value of the Fdms of FD1 was of the same order of magnitude as that of the Fdm of P. putida J3 (2,770 μmol-formaldehyde/mg-protein/min) [Citation9]). The Vmax values of these Fdms were several tens times higher than that of MNOs of A. methanolica and M. gastri (85 µmol-formaldehyde/mg-protein/min and 132 µmol-formaldehyde/mg-protein/min) [Citation14]).
Figure 4. Effect of formaldehyde concentration on the formaldehyde dismutase activity of the Fdms of FD1 and the Lineweaver-Burk plots (inset).
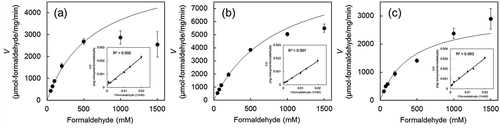
Table 3. Enzymatic kinetic parameters of Fdm1, Fdm2, and Fdm3 of FD1.
The actual formaldehyde dismutase activities of Fdm1 and Fdm2 were lower than those of the theoretical Michaelis–Menten curve in the range where formaldehyde concentrations exceeded 500 mM. These results suggest that substrate inhibition by formaldehyde occurred in Fdm1 and Fdm2 at formaldehyde concentrations over 500 mM. However, the activity of Fdm3 was not inhibited even at 1500 mM formaldehyde.
Substrate specificity
The substrate specificity of Fdm1, Fdm2, and Fdm3 was examined using formaldehyde, acetaldehyde, propionaldehyde, butyraldehyde, and valeraldehyde. As shown in , the enzyme activities of the Fdms for formaldehyde was tens to hundreds of times higher than those of the other substrates. Fdms of FD1 were active toward propionaldehyde and butyraldehyde, differing from the Fdm of P. putida F61[Citation6]).
Alcohol:NDMA oxidoreductase activity
Kato et al. showed that the Fdm of P. putida F61 has alcohol:NDMA oxidoreductase (EC 1.1.99.36) activity for various alcohols (except methanol) as a substrate [Citation8]). We examined whether the Fdms of FD1 have alcohol:NDMA oxidoreductase activity using methanol and ethanol as substrates. As a result, each of the Fdms of FD1 showed alcohol:NDMA oxidoreductase activity for methanol and ethanol (). This result reveals that the Fdms of FD1 have a methanol:NDMA oxidoreductase activity that is not found in the Fdm of P. putida F61 [Citation8]). The methanol:NDMA oxidoreductases (MNOs) with formaldehyde dismutase activity of A. methanolica and M. gastri have methanol dehydrogenase activity [Citation15]). However, these MNOs are distinct from the Fdms of FD1 because no homology with the amino acid sequences of Fdms of the FD1 was detected (data not shown), the enzyme-bound coenzyme is NADP(H) [Citation14]), and the quaternary structure is homodecamer [Citation14]). From the above, Fdm1, Fdm2, and Fdm3 of FD1 are different from already-known Fdms.
Table 4. Alcohol-NDMA oxidoreductase activities of Fdm1, Fdm2, and Fdm3 of FD1.
Conclusion
In the present study, three formaldehyde dismutases (Fdms) (with His-tag) (Fdm1, Fdm2, and Fdm3) of Methylobacterium sp. FD1 were produced in the recombinant E. coli and purified by immobilized metal affinity chromatography (IMAC) from the E. coli extracts, and characterized. Each of the Fdms of FD1 showed methanol:p-nitroso-N,N-dimethylaniline (NDMA) oxidoreductase activity that is not found in the Fdms of P. putida F61 and P. putida J3.
Authors’ contributions
S. I. and H. Y. performed the experiments in . S. I., N. T., and H. Y. performed the experiments in and . S. I. performed the experiments in , and , . N. T. and N. K. performed the experiment in . S. I., N. T., H. Y., and N. K. analyzed the data. H. Y. designed and directed the project. S. I. wrote the article.
Acknowledgments
We thank Dr. Yoshiharu Okuno of National Institute of Technology, Wakayama College for helping for GC.
Disclosure statement
No potential conflict of interest was reported by the authors.
References
- Yonemitsu H, Shiozaki E, Hitotsuda F, et al. Biodegradation of high concentrations of formaldehyde by lyophilized cells of Methylobacterium sp. FD1. Biosci Biotechnol Biochem. 2016;80(11):2264–2270..
- Tanaka N, Kusakabe Y, Ito K, et al. Crystal structure of formaldehyde dehydrogenase from Pseudomonas putida : the structural origin of the tightly bound cofactor in nicotinoprotein dehydrogenases. J Mol Biol. 2002;324(3):519–533. .
- Yonemitsu H, Kikuchi Y. Biodegradation of high concentrations of formaldehyde using Escherichia coli expressing the formaldehyde dismutase gene of Methylobacterium sp. FD1. Biosci Biotechnol Biochem. 2018;82(1):49–56.
- Yanase H, Noda H, Aoki K, et al. Cloning, sequence analysis, and expression of the gene encoding formaldehyde dismutase from Pseudomonas putida F61. Biosci Biotechnol Biochem. 1995;59(2):197–202. .
- Blaschke L, Wagner W, Werkmeister C, et al. Development of a simplified purification method for a novel formaldehyde dismutase variant from Pseudomonas putida J3. J Biotechnol. 2017;241:69–75.
- Kato N, Shirakawa K, Kobayashi H, et al. The dismutation of aldehydes by a bacterial enzyme. Agric Biol Chem. 1983;47:39–46.
- Kato N, Kobayashi H, Shimao M, et al. Properties of formaldehyde dismutation catalyzing enzyme of Pseudomonas putida F61. Agric Biol Chem. 1984;48:2017–2023.
- Kato N, Yamagami T, Shimao M, et al. Formaldehyde dismutase, a novel NAD-binding oxidoreductase from Pseudomonas putida F61. Eur J Biochem. 1986;156(1):59–64. .
- Blaschke L. Heterologe Expression, Charakterisierung und Anwendung einer Formaldehyd-Dismutase zur Gewinnung von Methanol aus Methan. Universität Stuttgart;2017, Thesis.
- Haldimann A, Daniels LL, Wanner BL. Use of new methods for construction of tightly regulated arabinose and rhamnose promoter fusions in studies of theEscherichia coli phosphate regulon. J. Bacteiol. 1998;180(5):1277–1286. .
- Nash T. The colorimetric estimation of formaldehyde by means of the Hantzsch reaction. Biochem J. 1953;55(3):416–421.
- Dunn MF, Bernhard SA. Rapid kinetic evidence for adduct formation between the substrate analog p-nitroso-N, N-dimethylaniline and reduced nicotinamide-adenine dinucleotide during enzymic reduction. Biochem. 1971;10(24):4569–4575.
- Ito K, Takahashi M, Yoshimoto T, et al. Cloning and high-level expression of the glutathione-independent formaldehyde dehydrogenase gene from Pseudomonas putida. J Bacteriol. 1994;176(9):2483–2491. .
- Bystrykh LV, Vonck J, van Bruggen EF, et al. Electron microscopic analysis and structural characterization of novel NADP(H)-containing methanol: N, N’-dimethyl-4-nitrosoaniline oxidoreductases from the gram-positive methylotrophic bacteria Amycolatopsis methanolica and Mycobacterium gastri MB19. J Bacteriol. 1993;175(6):1814–1822. .
- Bystrykh LV, Govorukhina NI, van Ophem PW, et al. Formaldehyde dismutase activities in Gram-positive bacteria oxidizing methanol. J Gen Microbiol. 1993;139(9):1979–1985.