Abstract
Noncoding RNAs (ncRNAs) are a group of RNA molecules that cannot encode proteins, and a better understanding of the complex interaction networks coordinated by ncRNAs will provide a theoretical basis for the development of therapeutics targeting the regulatory effects of ncRNAs. Platelets are produced upon the differentiation of hematopoietic stem cells into megakaryocytes, 1011 per day, and are renewed every 8–9 days. The process of thrombopoiesis is affected by multiple factors, in which ncRNAs also exert a significant regulatory role. This article reviewed the regulatory roles of ncRNAs, mainly microRNAs (miRNAs), circRNAs (circular RNAs), and long non-coding RNAs (lncRNAs), in thrombopoiesis in recent years as well as their roles in primary immune thrombocytopenia (ITP).
Plain Language Summary
What is the context?
Platelets are produced from progenitor cells named megakaryocytes (MKs) differentiated from bone marrow-derived hematopoietic stem cells (HSCs).
Thrombopoiesis refers to the process by which platelet-producing MKs release platelet granules into peripheral blood under the shear force of blood flow for further development and maturation.
The process of megakaryocytopoiesis and thrombopoiesis is affected by multiple factors, wherein some ncRNAs also exert a significant regulatory role.
miRNAs/lncRNAs play a promising role in t primary immune thrombocytopenia (ITP).
What is new?
This article reviewed the regulatory roles of ncRNAs, mainly microRNAs (miRNAs), circRNAs (circular RNAs), and long non-coding RNAs (lncRNAs), in thrombopoiesis.
This article also reviewed the roles of ncRNAs in ITP.
What is the impact?
Changes in ncRNA expression are associated with changes in the production of MKs, thrombopoiesis, and platelet function, which allows a new understanding of the pathogenesis of many congenital or acquired platelet-related diseases.
Introduction
Noncoding RNAs (ncRNAs), a class of RNAs that do not encode proteins [Citation1], are actually transcribed by most of the mammalian genome [Citation2], and act as key regulators of physiological processes in the human body [Citation3,Citation4]. Therefore, an in-depth understanding of the complicated interaction network coordinated by non-coding RNAs will provide a substantial theoretical basis for developing therapeutic measures targeting the regulatory role of ncRNAs [Citation5,Citation6]. Platelets are produced from progenitor cells named megakaryocytes (MKs) differentiated from bone marrow-derived hematopoietic stem cells (HSCs) under the action of a series of hematopoietic growth factors [Citation7,Citation8]. At a steady state, MKs can support a daily production of 1011 platelets with a renewal every 8–9 days. This process is greatly influenced by environmental alterations, which may lead to a more than 10-fold increase in the production responding to stimulation [Citation9]. Thrombopoiesis refers to the process by which platelet-producing MKs release platelet granules into peripheral blood under the shear force of blood flow for further development and maturation [Citation10–12]. Thrombocytopenia is a disorder characterized by defective or low platelet production with a risk of possible life-threatening bleeding. Although a variety of key factors have been identified to mediate MK maturation and platelet release [Citation13–16], the regulatory mechanism of thrombopoiesis is still undefined. Despite lacking a nucleus, platelets possess multiple types of RNA inherited from MKs, encompassing protein-coding messenger RNAs (mRNAs) and ncRNAs such as microRNAs (miRNAs) and long noncoding RNAs (lncRNAs) [Citation17,Citation18], which have the major features of post-transcriptional RNA metabolism [Citation19,Citation20]. The process of thrombopoiesis is affected by multiple factors, wherein some ncRNAs also exert a significant regulatory role. Herein, this study systematically reviewed the research progress on the regulatory roles of non-coding RNAs, mainly miRNAs, and lncRNAs, on thrombopoiesis in recent years.
miRNA-mediated regulation of thrombopoiesis
MiRNAs are a category of endogenous small RNAs that are approximately 20–24 nt in length [Citation21]. The majority of miRNAs are localized in intronic regions that are highly conserved in different species [Citation22,Citation23] and are highly homologous in sequence, which is strongly linked to the significance of its function [Citation24], exhibiting fine-tuned control of protein expression through recognition, binding, and translational repression of complementary sequences to protein-coding mRNA transcripts [Citation25,Citation26]. The diverse functions and expression patterns of miRNAs in platelets or MKs reveal that they may play key modulatory roles in the biology of platelets. Circulating platelets are essential in the physiology of blood coagulation that maintains the hemostatic balance and protects vascular integrity [Citation27]. Conversely, abnormality in platelet reactivity is related to diverse pathological factors such as cardiovascular disorders, inflammation, etc., leading to morbidity and mortality [Citation28–31]. Multiple miRNAs have been uncovered in platelets, but their roles remain greatly unknown. One such miRNA, miR-223, is documented to be the most abundant miRNA in platelets [Citation32,Citation33], followed by miR-126 [Citation20,Citation34]. MiR-96, miR-200b, miR-495, miR-107, and miR-223 are underscored to closely correlate with reactivity, aggregation, secretion, and adhesion of platelets [Citation35].
In recent years, miRNAs have gradually attracted attention, and changes in the miRNA expression signatures in cancers have been observed, rendering them prognostic and auxiliary diagnostic predictors [Citation36]. Additionally, miRNAs are important mediators of hematopoietic progenitor cell differentiation into MKs in the hematopoietic system [Citation37]. Present research focusing on the regulatory mechanism underlying the MK differentiation is the most in-depth stage across the whole process of thrombopoiesis, in which the action of miRNAs in the differentiation of MKs has also been gradually excavated. For instance, miR-125b and miR-660 can augment the number of polyploid MKs, but reversely, overexpression of the miR-23a/27A/24-2 cluster in human HSCs attenuates the MK maturation- and thrombopoiesis-related clone formation [Citation38]; miR-223-3p stimulates MK polyploidy via mediating the expression of human myosin heavy chain 10 (MYH10) [Citation39]; however, miR-146a inhibits the polyploidy in MKs [Citation40,Citation41]; miR-125b expedites the maturation and differentiation of MKs by controlling cell cycle [Citation42,Citation43]; miR-130a and miR-10a facilitate MK differentiation by regulating transcription factors MAFB and HOXA1, respectively, and both the two miRNAs are up-regulated in acute megakaryocytic leukemia cell lines [Citation37]; miR-22 inhibits the transcription of growth factor independent-1 (GFI1) to accelerate MK differentiation [Citation44]; miR-34a stimulates megakaryopoiesis by promoting the formation of MK colonies from HSCs and its expression is also increased during induction of MK differentiation in K562 cells [Citation45,Citation46]; miR-150 facilitates MK differentiation by targeting the transcription factor MYB [Citation47]; reversely, miR-155 restrains the differentiation of HSCs into MKs through cell cycle regulation [Citation48,Citation49]; miR-125a-5p acts on LCP1-encoded actin-binding protein L-plastin to reduce its expression, thereby affecting MK migration and proplatelet formation [Citation50] (). Since the discovery of miRNAs in platelets, regulatory roles of more and more miRNAs have been successively reported, revealing new regulatory mechanisms during MK differentiation (). Increasing attention has been paid to the expression patterns of different platelet miRNAs in a healthy or diseased status, and despite unique challenges, there is plenty of room for improvement in the research field of miRNA-dependent regulation of MK-platelet to shed light on the unknown molecular mechanisms that potentially modulate thrombopoiesis and platelet function.
Figure 1. miRNAs regulate the differentiation of hematopoietic stem cells to MKs to produce platelets. miRNAs act on the 3‘end of mRNAs to inhibit their degradation or translation of proteins by targeting the MRE.
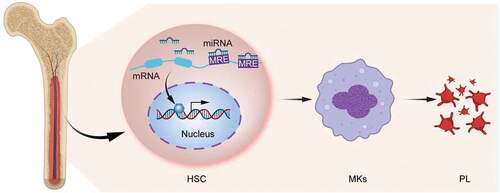
Table I. Functions and mechanisms of long non-coding RNAs.
LncRNA-mediated regulation of thrombopoiesis
Long non-coding RNAs (lncRNAs) refer to a class of RNAs longer than 200 nt that are not translated into proteins [Citation67]. LncRNAs exert regulatory effects on the expression of genes by binding to DNA, RNA, and proteins [Citation68–70], which are also referred to as competing endogenous RNAs (ceRNAs) such as HOTAIR [Citation58], PTV1 [Citation59], HOXa11-AS [Citation71], PFL [Citation72], and ARSR [Citation73]. LncRNA-miRNA interaction networks seem to be a major theme that existed in the biology of lncRNAs, which have been proven to be either the source or suppressors of miRNAs. LncRNAs play a significant role in the fine-tuning translation machinery and its regulation by modulating the key functions of other ncRNAs such as miRNAs [Citation74]. LncRNAs are derived not only from their own promoters but also from promoters shared with differently transcribed coding or non-coding genes, or from enhancer sequences [Citation75,Citation76]. LncRNAs have a unique evolutionary conserved pattern with higher tissue specificity [Citation77]. For years, studies have unraveled that multiple regulatory paradigms controlled by several established lncRNAs affect a wide range of cellular activities and are functionally relevant to normal development and pathophysiology in multiple diseases [Citation78,Citation79], including several types of cancers, neurological and cardiovascular diseases, immune and metabolic disorders. Several types of lncRNAs have been identified based on different parameters such as transcript length, association with annotated protein-coding genes,
and similarity to mRNA [Citation80]. These classes of RNA molecules are distinct from other RNAs relying on some unique characteristics, including alternative forms of biogenesis [Citation81], unique regulatory mechanism [Citation82], cis-regulatory activity, and RNA-binding domain with functional structure [Citation83,Citation84] (). Although lncRNAs have attracted attention from different research teams, much is still unknown about their functions and structures.
LncRNAs play a crucial role in maintaining the human HSC population and are well-known to be critical for the identification of specific differentiation pathways of HSCs. For example, lncHSC-2 regulates the self-renewal and differentiation of HSCs [Citation85]. Another lncRNA shlnc-EC6 is related to erythroid differentiation [Citation86]. HOTAIRM1 acts as a cell cycle regulator during myeloid maturation in addition to granulocyte differentiation [Citation87,Citation88]. EGO positively regulates several genes essential for eosinophil production [Citation89]. LINC00173 can stimulate granulocyte differentiation and myeloid colony formation [Citation90]. Hematopoietic development shows similar processes in both mice and humans [Citation91]. However, the lncRNAs involved in mouse and human hematopoiesis are poorly conserved, suggesting different evolution patterns of lncRNAs [Citation92]. Nevertheless, the implications of lncRNAs in MK differentiation and thrombopoiesis represent a largely unexplored area. Megakaryopoiesis is controlled by intricate networks involving bone marrow hematopoietic growth factors [Citation93]. Among diverse regulators, lncRNAs are also a group of critical regulators for the induction of MKs and thrombopoiesis (). Little is known about the roles of lncRNAs in megakaryopoiesis. Many past studies have revealed that some lncRNAs are abnormally expressed in myeloid progenitors and MKs. LncRNA AS-RBM15, which is situated on chromosome 1 with a total length of 52 797 bp, transcribed in the antisense orientation, and overlapped with the 5’ untranslated region (UTR) of the protein-coding gene RNA-binding protein 15 (RBM15), is initially unveiled to control the differentiation of MKs [Citation98]. RBM15 is highly expressed during hematopoiesis, particularly in myeloid differentiation and other hematopoietic cell lines, in which its mechanism is mediated by RBP-Jkappa-dependent Notch signaling activation, but lowly expressed in MKs [Citation94]. Differential expression of RBM15 crucially functions in the development of myeloid, MKs, and progenitor cell subsets as well as dysfunction-related disorders [Citation95]. There is also evidence in another study supporting the involvement of this antisense RNA transcript lncRNA AS-RBM15 in the transcription and translation regulation of the RBM15 protein in MKs [Citation96]. Activation of lncRNA AS-RBM15 plays a direct inducing role in the protein synthesis of RBM15, an extremely important control component of MK-terminal differentiation [Citation99] (). Besides, the transcription factor RUNX1 restrains the erythroid gene expression program during MK differentiation [Citation100] and maintains the activities of RBM15 and AS-RBM15 as a consequence of erythroid gene expression patterns. These genes are restricted by epigenetic repression of the master erythroid regulator, krueppel-like factor 1 (KLF1), during MK differentiation [Citation101]. Furthermore, the current study shows the high AS-RBM15 expression reflecting that the function of RUNX1 affects the balance between erythroid and MK differentiation [Citation80]. In addition, a recent study has reported that lncRNA NEAT1 participates in MK differentiation and thrombopoiesis and its mechanism of action affects the cell cycle and promotes the differentiation of MEG-01 cells and production of platelet granules [Citation97]. There is scientific evidence demonstrating that the biological functions of lncRNAs cannot be ignored and integrated complementary approaches are demanded for these critical lncRNAs. These approaches are varied depending on their modes of action in the development of MKs and disease processes. Furthermore, these lncRNAs may be implicated in multiple pathways related to the developmental process of MKs.
Figure 2. LncAS-RBM15 participation in the regulation of megakaryocytopoiesis and thrombopoiesis. LncAS-RBM15 combines with RBM15-mRNA and enhances RBM15 protein translation. The transcription factor RUNX1 can activate the expression of both RBM15 and AS-RBM15.
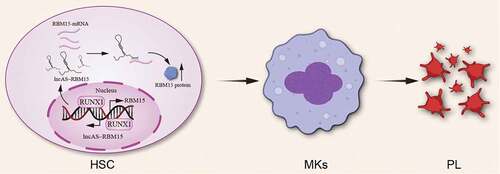
Table II. Examples of mechanistically studied miRNAs and lncRNAs with roles during hematopoiesis.
Abundant circular RNAs expression in differentiating megakaryocytes
CircRNAs are a subgroup of highly stable non-coding RNA, which are dominantly generated from pre-mRNA back-splicing [Citation102]. Recently, studies have shown several potential functions of circRNAs, which can interact with RNA-binding proteins or modulate transcription by sponging miRNAs [Citation103,Citation104]. Furthermore, circRNAs are currently recognized as important regulatory molecules in cell differentiation, cell proliferation, and apoptosis [Citation105]. However, the functions of circRNAs during hematopoietic differentiation are still not clear. High-throughput sequencing revealed that circRNAs are highly enriched in human platelets [Citation106]. The mRNAs decay more rapidly than circRNAs in platelet formation. Therefore, circRNAs are hugely enriched in platelets compared with megakaryocytes. By comparing the expression pattern of circRNAs and mRNAs, circRNAs were demonstrated to modulate hematopoietic differentiation and their expression significantly increased in differentiating megakaryocytes [Citation107]. Xia et al. identify a circular RNA named cia-cGAS, which can regulate the balance between self-renewal and differentiation of hematopoietic stem cells [Citation108]. These results suggest that circRNAs might play important role in megakaryocytopoiesis and thrombopoiesis.
The roles of ncRNAs in primary immune thrombocytopenia
Primary immune thrombocytopenia (ITP) is a clinically common, acquired autoimmune hemorrhagic disease in the absence of obvious exogenous factors, predominantly characterized by increased platelet destruction, peripheral blood thrombocytopenia, and normal MKs or accompanied by maturation disorder. This disease frequently recurs and persists and can be divided into acute and chronic disorders according to the duration of the disease: acute ITP patients are more commonly present in children often with a history of infection before the onset, showing a relatively short disease course, most of which can be self-cured; chronic ITP is most often diagnosed in adults with unclear etiology, slow onset, and frequent recurrence that is difficult to relieve spontaneously [Citation109,Citation110]. As an autoimmune disease, abnormal immune responses develop in vivo following ITP, yet its pathogenesis is still unclear [Citation111]. It is currently believed that the pathogenesis of ITP is a multi-factorial process. In addition to the involvement of cellular immunity, humoral immunity, MK maturation disorder, and the complement system, some recently identified new mechanisms may be suggested to participate in the onset of ITP, such as the dysregulation of miRNAs/lncRNAs [Citation112], FcγR-independent platelet clearance pathway [Citation113,Citation114], soluble programmed cell death receptor-1 (sPD-1) [Citation115–117], and metalloproteinase 10 (ADAM10) [Citation118,Citation119]. Existing research data have elucidated an immunoregulatory effect of miRNAs/lncRNAs on immune response and immune cells, indicating that miRNAs/lncRNAs play a promising role in the pathogenesis of ITP. The regulatory role of miRNAs/lncRNAs in thrombopoiesis has recently become a research hotspot. Currently, the effect of miRNAs/lncRNAs on platelet function in ITP patients is still undetermined. Accumulating studies have suggested that abnormally expressed miRNAs/lncRNAs are involved in the pathogenesis of ITP [Citation120]. One such miRNA, miR-55, targets the suppressor of cytokine signaling 1 (SOCS1), promoting the occurrence of ITP [Citation121]; miR-130a is also involved in the development of ITP via targeting transforming growth factor (TGF-β1) and interleukin 18 (IL-18) [Citation122]; silencing miR-155-5p upregulates SOCS1 and accelerates PD1/PDL1 pathway-mediated M2 macrophage polarization to prevent the development of ITP [Citation123]; miR-106b-5p induces the imbalance of immune cells Treg/Th17 in ITP through the NR4A3/Foxp3 pathway [Citation124]; miR-557 suppresses the differentiation and maturation of MKs in ITP [Citation125]; miR-409-3p expression is upregulated in the PBMCs of ITP patients after effective treatment, and IFN-γ is confirmed to be a target gene of miR-409-3p [Citation126]. LncRNA TMEVPG1 stimulates IFN-γ transcription to slow down the progression of ITP [Citation127]. LncRNA MEG3 inhibits the miR-125a-5p expression and triggers an immune imbalance of Treg/Th17 in ITP [Citation128]; LncRNA GAS5 alleviates ITP by motivating STAT3 ubiquitination and inhibiting the differentiation of immune cells Th17 [Citation129]; LncRNA PVT1 targets NOTCH1 to elicit the immune imbalance of Treg/Th17 ratio, facilitating the occurrence of ITP [Citation130]. With a deepened understanding of ncRNAs, it is still worth exploring the significance of ncRNAs in the pathogenesis of ITP; for instance, whether the differential expression of ncRNAs in plasma can serve as specific indicators of ITP (). All these issues require an illustration through more thorough fundamental research and clinical observations. Therapeutic targeting of miRNAs and lncRNAs represents a promising approach for the treatment of many diseases such as cardiovascular disease and cancer. Over the past few decades, several RNA-based therapies, including ASO anti-microRNAs (antimiRs), short hairpin RNAs (shRNAs), small interfering RNAs (siRNAs), and antisense oligonucleotides (ASOs) have been trying to translate into clinical application. However, only more than ten RNA-based therapeutics are approved by the Food and Drug Administration (FDA) and the European Medicines Agency (EMA) until now. All these RNA drugs are either ASOs or siRNAs, which can cause specific gene degradation in the central nervous system, muscle, or liver [Citation131]. Currently, no therapeutics targeting miRNAs and lncRNAs in megakaryopoiesis and thrombopoiesis enter the clinic. In the future, the issues associated with tolerability, delivery methods, and specificity must be overcome.
Table III. Examples of mechanistically studied miRNAs and lncRNAs with roles during ITP.
Perspectives
With the changing understanding of ncRNA based on the continuous deepening exploration, ncRNAs have gradually emerged as important regulators of gene expression in the human body; the aberrant expression of ncRNA has become a pathogenic factor to the human body and also they serve as targets for the treatment of various human diseases. At present, much is unknown about the multiple functions, corresponding targets, and specific molecular mechanisms of ncRNAs. Although thousands of ncRNAs are expressed during hematopoiesis, studies on the functions of specific ncRNAs with experimental validation are limited. The increasing demand to decipher the exact functions of ncRNAs in the development of MKs and thrombopoiesis raises further research questions. Up to now, the significance of ncRNAs in megakaryocytopoiesis in relation to lineage-specific development has only been described in a few studies. Under different physiological conditions, an in-depth understanding of the changes in the ncRNA transcriptome in MKs, particularly how these changes indicate their importance in the development and progression of MKs, may contribute to the identification of new cellular pathways related to the generation of mature MKs from hematopoietic stem/progenitor cells (HSPCs). Understanding the ncRNA profile is also of crucial importance to provide information to directly reflect MK lineage relationship and functional similarities to the hematopoietic system. Furthermore, deep excavation of the ncRNA profiles in the MK population is also an efficient way to identify and predict their novel developmental roles in terminal megakaryocytopoiesis and subsequent platelet production.
Taken together, changes in ncRNA expression are associated with changes in the production of MKs, thrombopoiesis, and platelet function. In recent years, scientists have developed methods to generate platelets by in vitro inducing the differentiation of umbilical cord blood stem cells, bone marrow HSCs, human induced pluripotent stem cells, and mesenchymal stem cells into MKs. Nevertheless, due to limited stem cell sources, deficient platelet production, and other factors such as the potential carcinogenic risk of induced pluripotent stem cells, these studies are still in the laboratory exploration stage, unable to achieve mass production for clinical application. Research on MK differentiation suggests the possibility of producing clinical-grade platelets in vitro on a large scale. Meanwhile, the improvement of platelet isolation technology aids in improving the quality and quantity of platelets. Great progress has been made in the investigation of molecular mechanisms, which allows a new understanding of the pathogenesis of many congenital or acquired platelet-related diseases.
Acknowledgments
We thank the Home for Researchers editorial team (www.home-for-researchers.com) for editing the language of the manuscript.
Disclosure statement
No potential conflict of interest was reported by the authors.
Additional information
Funding
References
- Wu J, Nagy LE, Liangpunsakul S, Wang L. Non-coding RNA crosstalk with nuclear receptors in liver disease. Biochim Biophys Acta Mol Basis Dis. 2021;1867(5):166083. doi:10.1016/j.bbadis.2021.166083. Epub 2021/01/27.
- Zhong X, Zhang D, Xiong M, Zhang L. Noncoding RNA for cancer gene therapy. Recent Results Cancer Res. 2016;209:51–10. Epub 2017/01/20.
- Farber BA, Lalazar G, Simon EP, Hammond WJ, Requena D, Bhanot UK, La Quaglia MP, Simon SM. Non coding RNA analysis in fibrolamellar hepatocellular carcinoma. Oncotarget. 2018;9(12):10211–10227. doi:10.18632/oncotarget.23325. Epub 2018/03/15.
- Webb A, Papp AC, Curtis A, Newman LC, Pietrzak M, Seweryn M, Handelman SK, Rempala GA, Wang D, Graziosa E, et al. RNA sequencing of transcriptomes in human brain regions: protein-coding and non-coding RNAs, isoforms and alleles. BMC Genomics. 2015;16(1):990. doi:10.1186/s12864-015-2207-8. Epub 2015/11/26.
- Rossi JJ. A novel nuclear miRNA mediated modulation of a non-coding antisense RNA and its cognate sense coding mRNA. EMBO J. 2011;30(21):4340–4341. doi:10.1038/emboj.2011.373. Epub 2011/11/04.
- Ruwe H, Schmitz-Linneweber C. Short non-coding RNA fragments accumulating in chloroplasts: footprints of RNA binding proteins? Nucleic Acids Res. 2012;40(7):3106–3116. doi:10.1093/nar/gkr1138. Epub 2011/12/06.
- Ozturk HM, Ozturk S, Yetkin E. Linkage between cardiovascular diseases and major depression: contribution of platelet cells. Psychiatry Res. 2020;287:111026. doi:10.1016/j.psychres.2017.11.079. Epub 2018/01/11.
- Olas B. Biochemistry of blood platelet activation and the beneficial role of plant oils in cardiovascular diseases. Adv Clin Chem. 2020;95:219–243. Epub 2020/03/04.
- Kaushansky K. The molecular mechanisms that control thrombopoiesis. J Clin Invest. 2005;115(12):3339–3347. doi:10.1172/JCI26674. Epub 2005/12/03.
- Giannini S, Lee-Sundlov MM, Rivadeneyra L, Di Buduo CA, Burns R, Lau JT, Falet H, Balduini A, Hoffmeister KM. β4GALT1 controls β1 integrin function to govern thrombopoiesis and hematopoietic stem cell homeostasis. Nat Commun. 2020;11(1):356. doi:10.1038/s41467-019-14178-y. Epub 2020/01/19.
- Asquith NL, Machlus KR. Teamwork makes the dream work in thrombopoiesis. Blood. 2019;134(10):791–792. doi:10.1182/blood.2019002306. Epub 2019/09/07.
- Kaushansky K. Thrombopoiesis. Semin Hematol. 2015;52(1):4–11. doi:10.1053/j.seminhematol.2014.10.003. Epub 2015/01/13.
- Wang H, Liu C, Liu X, Wang M, Wu D, Gao J, Su P, Nakahata T, Zhou W, Xu Y, et al. MEIS1 regulates hemogenic endothelial generation, megakaryopoiesis, and thrombopoiesis in human pluripotent stem cells by targeting TAL1 and FLI1. Stem Cell Reports. 2018;10(2):447–460. doi:10.1016/j.stemcr.2017.12.017. Epub 2018/01/24.
- Metcalf DB. Thrombopoietin — at last. Nature. 1994;369(6481):519–520. doi:10.1038/369519a0. Epub 1994/06/16.
- Kaushansky K, Lok S, Holly RD, Broudy VC, Lin N, Bailey MC, Forstrom JW, Buddle MM, Oort PJ, Hagen FS, et al. Promotion of megakaryocyte progenitor expansion and differentiation by the c-Mpl ligand thrombopoietin. Nature. 1994;369(6481):568–571. doi:10.1038/369568a0. Epub 1994/06/16.
- Chen S, Qi Y, Wang S, Xu Y, Shen M, Hu M, Du C, Chen F, Chen M, Lu Y, et al. Melatonin enhances thrombopoiesis through ERK1/2 and Akt activation orchestrated by dual adaptor for phosphotyrosine and 3-phosphoinositides. J Pineal Res. 2020;68(3):e12637. doi:10.1111/jpi.12637. Epub 2020/02/14.
- Yan S, Liu X, Ke X, Xian Z, Peng C, Wang X, Chen M. Screening on platelet LncRNA expression profile discloses novel residual platelet reactivity biomarker. Int J Lab Hematol. 2020;42(5):661–668. doi:10.1111/ijlh.13261. Epub 2020/06/23.
- Tran JQD, Pedersen OH, Larsen ML, Grove EL, Kristensen SD, Hvas AM, Nissen PH. Platelet microRNA expression and association with platelet maturity and function in patients with essential thrombocythemia. Platelets. 2020;31(3):365–372. doi:10.1080/09537104.2019.1636019. Epub 2019/06/27.
- Mukai N, Nakayama Y, Ishi S, Ogawa S, Maeda S, Anada N, Murakami S, Mizobe T, Sawa T, Nakajima Y. Changes in MicroRNA expression level of circulating platelets contribute to platelet defect after cardiopulmonary bypass. Crit Care Med. 2018;46(8):e761–767. doi:10.1097/CCM.0000000000003197. Epub 2018/05/10.
- Zhou M, Gao M, Luo Y, Gui R, Ji H. Long non-coding RNA metallothionein 1 pseudogene 3 promotes p2y12 expression by sponging miR-126 to activate platelet in diabetic animal model. Platelets. 2019;30(4):452–459. doi:10.1080/09537104.2018.1457781.
- Fiedler J, Jazbutyte V, Kirchmaier BC, Gupta SK, Lorenzen J, Hartmann D, Galuppo P, Kneitz S, Pena JT, Sohn-Lee C, et al. MicroRNA-24 regulates vascularity after myocardial infarction. Circulation. 2011;124(6):720–730. doi:10.1161/CIRCULATIONAHA.111.039008. Epub 2011/07/27.
- Citrin KM, Fernandez-Hernando C, Suarez Y. MicroRNA regulation of cholesterol metabolism. Ann N Y Acad Sci. 2021;1495(1):55–77. doi:10.1111/nyas.14566. Epub 2021/02/02.
- Chen FY, Bi L, Qian L, Gao J, Jiang YC, Chen WP. Identification of multidrug resistance gene MDR1 associated microRNA of salvianolic acid a reversal in lung cancer. Zhongguo Zhong Yao Za Zhi. 2016;41(17):3279–3284. doi:10.4268/cjcmm20161726. Epub 2017/09/19.
- Yang J, Ma D, Fesler A, Zhai H, Leamniramit A, Li W, Wu S, Ju J. Expression analysis of microRNA as prognostic biomarkers in colorectal cancer. Oncotarget. 2017;8(32):52403–52412. doi:10.18632/oncotarget.14175. Epub 2017/09/09.
- Dangwal S, Thum T. MicroRnas in platelet biogenesis and function. Thromb Haemost. 2012;108(10):599–604. doi:10.1160/TH12-03-0211. Epub 2012/07/12.
- Gatsiou A, Boeckel JN, Randriamboavonjy V, Stellos K. MicroRnas in platelet biogenesis and function: implications in vascular homeostasis and inflammation. Curr Vasc Pharmacol. 2012;10(5):524–531. doi:10.2174/157016112801784611. Epub 2012/02/18.
- Faria AVS, Andrade SS, Peppelenbosch MP, Ferreira-Halder CV, Fuhler GM. The role of phospho-tyrosine signaling in platelet biology and hemostasis. Biochim Biophys Acta Mol Cell Res. 2021;1868(3):118927. doi:10.1016/j.bbamcr.2020.118927. Epub 2020/12/15.
- Boilard E, Nigrovic PA, Larabee K, Watts GF, Coblyn JS, Weinblatt ME, Massarotti EM, Remold-O’Donnell E, Farndale RW, Ware J, et al. Platelets amplify inflammation in arthritis via collagen-dependent microparticle production. Science. 2010;327(5965):580–583. doi:10.1126/science.1181928. Epub 2010/01/30.
- Jain S, Harris J, Ware J. Platelets: linking hemostasis and cancer. Arterioscler Thromb Vasc Biol. 2010;30(12):2362–2367. doi:10.1161/ATVBAHA.110.207514. Epub 2010/11/13.
- Kottke-Marchant K. Importance of platelets and platelet response in acute coronary syndromes. Cleve Clin J Med. 2009;76(Suppl 1):S2–7. doi:10.3949/ccjm.76.s1.01. Epub 2009/04/03.
- Nurden AT. Platelets, inflammation and tissue regeneration. Thromb Haemost. 2011;105(Suppl 1):S13–33. doi:10.1160/THS10-11-0720. Epub 2011/04/12.
- Parra-Izquierdo I, McCarty OJT, Aslan JE. Platelet miR-223 delivery rescues vascular cells in Kawasaki disease. Circ Res. 2020;127(7):874–876. doi:10.1161/CIRCRESAHA.120.317796. Epub 2020/09/11.
- Su M, Fan S, Ling Z, Fan X, Xia L, Liu Y, Li S, Zhang Y, Zeng Z, Tang WH. Restoring the platelet miR-223 by Calpain inhibition alleviates the neointimal hyperplasia in diabetes. Front Physiol. 2020;11:742. doi:10.3389/fphys.2020.00742. Epub 2020/08/01.
- Sun Y, Liu XL, Zhang D, Liu F, Cheng YJ, Ma Y, Zhou YJ, Zhao YX. Platelet-derived exosomes affect the proliferation and migration of human umbilical vein endothelial cells via miR-126. Curr Vasc Pharmacol. 2019;17(4):379–387. doi:10.2174/1570161116666180313142139. Epub 2018/03/14.
- Kondkar AA, Bray MS, Leal SM, Nagalla S, Liu DJ, Jin Y, Dong JF, Ren Q, Whiteheart SW, Shaw C, et al. VAMP8/Endobrevin is overexpressed in hyperreactive human platelets: suggested role for platelet microRNA. J Thromb Haemost. 2010;8(2):369–378. doi:10.1111/j.1538-7836.2009.03700.x.
- Pichler M, Calin GA. MicroRnas in cancer: from developmental genes in worms to their clinical application in patients. Br J Cancer. 2015;113(4):569–573. doi:10.1038/bjc.2015.253. Epub 2015/07/15.
- Garzon R, Pichiorri F, Palumbo T, Iuliano R, Cimmino A, Aqeilan R, Volinia S, Bhatt D, Alder H, Marcucci G, et al. MicroRNA fingerprints during human megakaryocytopoiesis. Proc Natl Acad Sci USA. 2006;103(13):5078–5083. doi:10.1073/pnas.0600587103.
- Emmrich S, Henke K, Hegermann J, Ochs M, Reinhardt D, Klusmann JH. miRnas can increase the efficiency of ex vivo platelet generation. Ann Hematol. 2012;91(11):1673–1684. doi:10.1007/s00277-012-1517-z. Epub 2012/07/06.
- Shi R, Zhou X, Ji WJ, Zhang YY, Ma YQ, Zhang JQ, Li YM. The emerging role of miR-223 in platelet reactivity: implications in antiplatelet therapy. Biomed Res Int. 2015;2015:981841. doi:10.1155/2015/981841. Epub 2015/07/30.
- Opalinska JB, Bersenev A, Zhang Z, Schmaier AA, Choi J, Yao Y, D’Souza J, Tong W, Weiss MJ. MicroRNA expression in maturing murine megakaryocytes. Blood. 2010;116(23):e128–138. doi:10.1182/blood-2010-06-292920. Epub 2010/08/20.
- Labbaye C, Spinello I, Quaranta MT, Pelosi E, Pasquini L, Petrucci E, Biffoni M, Nuzzolo ER, Billi M, Foa R, et al. A three-step pathway comprising PLZF/miR-146a/cxcr4 controls megakaryopoiesis. Nat Cell Biol. 2008;10(7):788–801. doi:10.1038/ncb1741. Epub 2008/06/24.
- Kandi R, Undi R, Gutti RK. MiR-125b regulates cell proliferation and survival in neonatal megakaryocytes. Ann Hematol. 2014;93(6):1065–1066. doi:10.1007/s00277-013-1928-5. Epub 2013/10/26.
- Qu M, Fang F, Zou X, Zeng Q, Fan Z, Chen L, Yue W, Xie X, Pei X. miR-125b modulates megakaryocyte maturation by targeting the cell-cycle inhibitor p19(ink4d). Cell Death Dis. 2016;7(10):e2430. doi:10.1038/cddis.2016.288. Epub 2016/10/21.
- Weiss CN, Ito K. microRNA-22 promotes megakaryocyte differentiation through repression of its target, GFI1. Blood Adv. 2019;3(1):33–46. doi:10.1182/bloodadvances.2018023804. Epub 2019/01/09.
- Ichimura A, Ruike Y, Terasawa K, Shimizu K, Tsujimoto G. MicroRNA-34a inhibits cell proliferation by repressing mitogen-activated protein kinase kinase 1 during megakaryocytic differentiation of K562 cells. Mol Pharmacol. 2010;77(6):1016–1024. doi:10.1124/mol.109.063321. Epub 2010/03/20.
- Navarro F, Gutman D, Meire E, Caceres M, Rigoutsos I, Bentwich Z, Lieberman J. miR-34a contributes to megakaryocytic differentiation of K562 cells independently of p53. Blood. 2009;114(10):2181–2192. doi:10.1182/blood-2009-02-205062. Epub 2009/07/09.
- Emambokus N, Vegiopoulos A, Harman B, Jenkinson E, Anderson G, Frampton J. Progression through key stages of haemopoiesis is dependent on distinct threshold levels of c-Myb. EMBO J. 2003;22:4478–4488. doi:10.1093/emboj/cdg434. Epub 2003/08/28.
- Lu J, Guo S, Ebert BL, Zhang H, Peng X, Bosco J, Pretz J, Schlanger R, Wang JY, Mak RH, et al. MicroRNA-mediated control of cell fate in megakaryocyte-erythrocyte progenitors. Dev Cell. 2008;14(6):843–853. doi:10.1016/j.devcel.2008.03.012. Epub 2008/06/10.
- Georgantas RW, 3rd, Hildreth R, Morisot S, Alder J, Liu CG, Heimfeld S, Calin GA, Croce CM, Civin CI. CD34+ hematopoietic stem-progenitor cell microRNA expression and function: a circuit diagram of differentiation control. Proc Natl Acad Sci USA. 2007;104(8):2750–2755. doi:10.1073/pnas.0610983104. Epub 2007/02/13.
- Bhatlekar S, Manne BK, Basak I, Edelstein LC, Tugolukova E, Stoller ML, Cody MJ, Morley SC, Nagalla S, Weyrich AS, et al. miR-125a-5p regulates megakaryocyte proplatelet formation via the actin-bundling protein L-plastin. Blood. 2020;136(15):1760–1772. doi:10.1182/blood.2020005230. Epub 2020/08/28.
- Fanucchi S, Fok ET, Dalla E, Shibayama Y, Borner K, Chang EY, Stoychev S, Imakaev M, Grimm D, Wang KC, et al. Immune genes are primed for robust transcription by proximal long noncoding RNAs located in nuclear compartments. Nat Genet. 2019;51(1):138–150. doi:10.1038/s41588-018-0298-2. Epub 2018/12/12.
- Ariel F, Lucero L, Christ A, Mammarella MF, Jegu T, Veluchamy A, Mariappan K, Latrasse D, Blein T, Liu C, et al. R-loop mediated trans action of the APOLO long noncoding RNA. Mol Cell. 2020;77(5):1055–1065 e1054. doi:10.1016/j.molcel.2019.12.015. Epub 2020/01/19.
- Rom A, Melamed L, Gil N, Goldrich MJ, Kadir R, Golan M, Biton I, Perry RBT, Ulitsky I. Regulation of CHD2 expression by the Chaserr long noncoding RNA gene is essential for viability. Nat Commun. 2019;10(1). doi:10.1038/s41467-019-13075-8.
- Grossi E, Raimondi I, Goni E, Gonzalez J, Marchese FP, Chapaprieta V, Martin-Subero JI, Guo S, Huarte M. A lncRNA-SWI/SNF complex crosstalk controls transcriptional activation at specific promoter regions. Nat Commun. 2020;11(1):936. doi:10.1038/s41467-020-14623-3. Epub 2020/02/20.
- Jain AK, Xi YX, McCarthy R, Allton K, Akdemir KC, Patel LR, Aronow B, Lin CR, Li W, Yang LQ, et al. LncPRESS1 is a p53-regulated LncRNA that safeguards pluripotency by disrupting SIRT6-mediated De-acetylation of Histone H3K56. Mol Cell. 2016;64(5):967–981. doi:10.1016/j.molcel.2016.10.039.
- Dimitrova N, Zamudio JR, Jong RM, Soukup D, Resnick R, Sarma K, Ward AJ, Raj A, Lee JT, Sharp PA, et al. LincRNA-p21 activates p21 in cis to promote Polycomb target gene expression and to enforce the G1/S checkpoint. Mol Cell. 2014;54(5):777–790. doi:10.1016/j.molcel.2014.04.025. Epub 2014/05/27.
- Yari H, Jin L, Teng L, Wang YF, Wu YY, Liu GZ, Gao W, Liang J, Xi YF, Feng YC, et al. LncRNA REG1CP promotes tumorigenesis through an enhancer complex to recruit FANCJ helicase for REG3A transcription. Nat Commun. 2019;10(1). doi:10.1038/s41467-019-13313-z.
- Cai H, Yao J, An Y, Chen X, Chen W, Wu D, Luo B, Yang Y, Jiang Y, Sun D, et al. LncRNA HOTAIR acts a competing endogenous RNA to control the expression of notch3 via sponging miR-613 in pancreatic cancer. Oncotarget. 2017;8(20):32905–32917. doi:10.18632/oncotarget.16462. Epub 2017/04/19.
- Wang BJ, Ding HW, Ma GA. Long noncoding RNA PVT1 promotes melanoma progression via endogenous sponging miR-26b. Oncol Res. 2018;26(5):675–681. doi:10.3727/096504017X14920318811730. Epub 2017/04/15.
- Guo CJ, Ma XK, Xing YH, Zheng CC, Xu YF, Shan L, Zhang J, Wang S, Wang Y, Carmichael GG, et al. Distinct processing of lncRNAs contributes to non-conserved functions in stem cells. Cell. 2020;181(3):621–636 e622. doi:10.1016/j.cell.2020.03.006. Epub 2020/04/08.
- Miao H, Wang LL, Zhan HM, Dai JS, Chang YB, Wu F, Liu T, Liu ZY, Gao CF, Li L, et al. A long noncoding RNA distributed in both nucleus and cytoplasm operates in the PYCARD-regulated apoptosis by coordinating the epigenetic and translational regulation. PLoS Genet. 2019;15(5):e1008144. doi:10.1371/journal.pgen.1008144.
- Lee S, Kopp F, Chang TC, Sataluri A, Chen B, Sivakumar S, Yu H, Xie Y, Mendell JT. Noncoding RNA NORAD regulates genomic stability by sequestering PUMILIO proteins. Cell. 2016;164(1–2):69–80. doi:10.1016/j.cell.2015.12.017.
- Schmitt AM, Garcia JT, Hung T, Flynn RA, Shen Y, Qu K, Payumo AY, Peres-da-Silva A, Broz DK, Baum R, et al. An inducible long noncoding RNA amplifies DNA damage signaling. Nat Genet. 2016;48(11):1370–1376. doi:10.1038/ng.3673. Epub 2016/10/28.
- Marchese FP, Grossi E, Marin-Bejar O, Bharti SK, Raimondi I, Gonzalez J, Martinez-Herrera DJ, Athie A, Amadoz A, Brosh RM, Jr., et al. A long noncoding RNA regulates sister chromatid cohesion. Mol Cell. 2016;63(3):397–407. doi:10.1016/j.molcel.2016.06.031. Epub 2016/08/02.
- Lewandowski JP, Lee JC, Hwang T, Sunwoo H, Goldstein JM, Groff AF, Chang NP, Mallard W, Williams A, Henao-Meija J, et al. The Firre locus produces a trans-acting RNA molecule that functions in hematopoiesis. Nat Commun. 2019;10(1). doi:10.1038/s41467-019-12970-4.
- Yin QF, Yang L, Zhang Y, Xiang JF, Wu YW, Carmichael GG, Chen LL. Long noncoding RNAs with snoRNA ends. Mol Cell. 2012;48(2):219–230. doi:10.1016/j.molcel.2012.07.033. Epub 2012/09/11.
- Guttman M, Rinn JL. Modular regulatory principles of large non-coding RNAs. Nature. 2012;482(7385):339–346. doi:10.1038/nature10887. Epub 2012/02/18.
- Guo X, Gao L, Wang Y, Chiu DK, Wang T, Deng Y. Advances in long noncoding RNAs: identification, structure prediction and function annotation. Brief Funct Genomics. 2016;15(1):38–46. doi:10.1093/bfgp/elv022. Epub 2015/06/15.
- Paralkar VR, Weiss MJ. Long noncoding RNAs in biology and hematopoiesis. Blood. 2013;121(24):4842–4846. doi:10.1182/blood-2013-03-456111. Epub 2013/05/07.
- Satpathy AT, Chang HY. Long noncoding RNA in hematopoiesis and immunity. Immunity. 2015;42(5):792–804. doi:10.1016/j.immuni.2015.05.004. Epub 2015/05/21.
- Chen D, Zhang M, Ruan J, Li X, Wang S, Cheng X, Zhao H, Zeng Y, Liu J, He K, et al. The long non-coding RNA HOXA11-AS promotes epithelial mesenchymal transition by sponging miR-149-3p in colorectal cancer. J Cancer. 2020;11(20):6050–6058. doi:10.7150/jca.49809. Epub 2020/09/15.
- Liang H, Pan Z, Zhao X, Liu L, Sun J, Su X, Xu C, Zhou Y, Zhao D, Xu B, et al. LncRNA PFL contributes to cardiac fibrosis by acting as a competing endogenous RNA of let-7d. Theranostics. 2018;8(4):1180–1194. doi:10.7150/thno.20846. Epub 2018/02/22.
- Li S, Zhu K, Liu L, Gu J, Niu H, Guo J. lncARSR sponges miR-34a-5p to promote colorectal cancer invasion and metastasis via hexokinase-1-mediated glycolysis. Cancer Sci. 2020;111(10):3938–3952. doi:10.1111/cas.14617. Epub 2020/08/17.
- Paraskevopoulou MD, Hatzigeorgiou AG. Analyzing MiRNA-LncRNA Interactions. Methods Mol Biol. 2016;1402:271–286. Epub 2016/01/02.
- Al-Tobasei R, Paneru B, Salem M. Genome-wide discovery of long non-coding RNAs in rainbow trout. PLoS One. 2016;11(2):e0148940. doi:10.1371/journal.pone.0148940. Epub 2016/02/20.
- Hu S, Wu J, Chen L, Shan G. Signals from noncoding RNAs: unconventional roles for conventional pol III transcripts. Int J Biochem Cell Biol. 2012;44(11):1847–1851. doi:10.1016/j.biocel.2012.07.013. Epub 2012/07/24.
- Vance KW, Ponting CP. Transcriptional regulatory functions of nuclear long noncoding RNAs. Trends Genet. 2014;30(8):348–355. doi:10.1016/j.tig.2014.06.001. Epub 2014/06/30.
- Melissari MT, Grote P. Roles for long non-coding RNAs in physiology and disease. Pflugers Arch. 2016;468(6):945–958. doi:10.1007/s00424-016-1804-y. Epub 2016/03/06.
- Giroud M, Scheideler M. Long non-coding RNAs in metabolic organs and energy homeostasis. Int J Mol Sci. 2017;18(12):2578. doi:10.3390/ijms18122578. Epub 2017/12/01.
- Kumari P, Sampath K. cncRnas: Bi-functional RNAs with protein coding and non-coding functions. Semin Cell Dev Biol. 2015;47-48:40–51. doi:10.1016/j.semcdb.2015.10.024. Epub 2015/10/27.
- Spurlock CF, 3rd, Crooke PS, 3rd, Aune TM. Biogenesis and transcriptional regulation of long noncoding RNAs in the human immune system. J Immunol. 2016;197(12):4509–4517. doi:10.4049/jimmunol.1600970. Epub 2016/12/04.
- Marchese FP, Raimondi I, Huarte M. The multidimensional mechanisms of long noncoding RNA function. Genome Biol. 2017;18(1):206. doi:10.1186/s13059-017-1348-2.
- Kornienko AE, Guenzl PM, Barlow DP, Pauler FM. Gene regulation by the act of long non-coding RNA transcription. BMC Biol. 2013;11:59. Epub 2013/06/01.
- Zampetaki A, Albrecht A, Steinhofel K. Long non-coding RNA structure and function: is there a link? Front Physiol. 2018;9:1201. Epub 2018/09/11.
- Luo M, Jeong M, Sun D, Park HJ, Rodriguez BA, Xia Z, Yang L, Zhang X, Sheng K, Darlington GJ, et al. Long non-coding RNAs control hematopoietic stem cell function. Cell Stem Cell. 2015;16:426–438. Epub 2015/03/17.
- Wang C, Wu X, Shen F, Li Y, Zhang Y, Yu D. Shlnc-EC6 regulates murine erythroid enucleation by Rac1-PIP5K pathway. Dev Growth Differ. 2015;57(6):466–473. doi:10.1111/dgd.12225.
- Wei S, Zhao M, Wang X, Li Y, Wang K. PU.1 controls the expression of long noncoding RNA HOTAIRM1 during granulocytic differentiation. J Hematol Oncol. 2016;9(1):44. doi:10.1186/s13045-016-0274-1.
- Zhang X, Weissman SM, Newburger PE. Long intergenic non-coding RNA HOTAIRM1 regulates cell cycle progression during myeloid maturation in NB4 human promyelocytic leukemia cells. RNA Biol. 2014;11(6):777–787. doi:10.4161/rna.28828.
- Wagner LA, Christensen CJ, Dunn DM, Spangrude GJ, Georgelas A, Kelley L, Esplin MS, Weiss RB, Gleich GJ. EGO, a novel, noncoding RNA gene, regulates eosinophil granule protein transcript expression. Blood. 2007;109(12):5191–5198. doi:10.1182/blood-2006-06-027987. Epub 2007/03/14.
- Schwarzer A, Emmrich S, Schmidt F, Beck D, Ng M, Reimer C, Adams FF, Grasedieck S, Witte D, Kabler S, et al. The non-coding RNA landscape of human hematopoiesis and leukemia. Nat Commun. 2017;8(1):218. doi:10.1038/s41467-017-00212-4. Epub 2017/08/11.
- de Freitas RM, da Costa Maranduba CM. Myeloproliferative neoplasms and the JAK/STAT signaling pathway: an overview. Rev Bras Hematol Hemoter. 2015;37(5):348–353. doi:10.1016/j.bjhh.2014.10.001. Epub 2015/09/27.
- Kutter C, Watt S, Stefflova K, Wilson MD, Goncalves A, Ponting CP, Odom DT, Marques AC. Rapid turnover of long noncoding RNAs and the evolution of gene expression. PLoS Genet. 2012;8(7):e1002841. doi:10.1371/journal.pgen.1002841. Epub 2012/07/31.
- Raghuwanshi S, Dahariya S, Musvi SS, Gutti U, Kandi R, Undi RB, Sahu I, Gautam DK, Paddibhatla I, Gutti RK. MicroRNA function in megakaryocytes. Platelets. 2019;30(7):809–816. doi:10.1080/09537104.2018.1528343. Epub 2018/10/26.
- Kazemzadeh M, Safaralizadeh R, Orang AV. LncRNAs: emerging players in gene regulation and disease pathogenesis. J Genet. 2015;94(4):771–784. doi:10.1007/s12041-015-0561-6. Epub 2015/12/23.
- Ma X, Renda MJ, Wang L, Cheng EC, Niu C, Morris SW, Chi AS, Krause DS. Rbm15 modulates Notch-induced transcriptional activation and affects myeloid differentiation. Mol Cell Biol. 2007;27(8):3056–3064. doi:10.1128/MCB.01339-06. Epub 2007/02/07.
- Garzon R, Volinia S, Papaioannou D, Nicolet D, Kohlschmidt J, Yan PS, Mrozek K, Bucci D, Carroll AJ, Baer MR, et al. Expression and prognostic impact of lncRNAs in acute myeloid leukemia. Proc Natl Acad Sci USA. 2014;111(52):18679–18684. doi:10.1073/pnas.1422050112.
- Bian W, Chen W, Jiang X, Qu H, Jiang J, Yang J, Liang X, Zhao B, Sun Y, Zhang C. Downregulation of long non-coding RNA nuclear Paraspeckle assembly transcript 1 inhibits MEG-01 differentiation and platelet-like particles activity. Front Genet. 2020;11:571467. doi:10.3389/fgene.2020.571467. Epub 2020/11/17.
- Tran NT, Su H, Khodadadi-Jamayran A, Lin S, Zhang L, Zhou D, Pawlik KM, Townes TM, Chen Y, Mulloy JC, et al. The AS-RBM15 lncRNA enhances RBM15 protein translation during megakaryocyte differentiation. EMBO Rep. 2016;17(6):887–900. doi:10.15252/embr.201541970. Epub 2016/04/28.
- Hu M, Yang Y, Ji Z, Luo J. RBM15 functions in blood diseases. Curr Cancer Drug Targets. 2016;16(7):579–585. doi:10.2174/1568009616666160112105706. Epub 2016/01/14.
- Kuvardina ON, Herglotz J, Kolodziej S, Kohrs N, Herkt S, Wojcik B, Oellerich T, Corso J, Behrens K, Kumar A, et al. RUNX1 represses the erythroid gene expression program during megakaryocytic differentiation. Blood. 2015;125(23):3570–3579. doi:10.1182/blood-2014-11-610519. Epub 2015/04/26.
- Lyu Y, Lou J, Yang Y, Feng J, Hao Y, Huang S, Yin L, Xu J, Huang D, Ma B, et al. Dysfunction of the WT1-MEG3 signaling promotes AML leukemogenesis via p53-dependent and -independent pathways. Leukemia. 2017;31(12):2543–2551. doi:10.1038/leu.2017.116. Epub 2017/04/13.
- Verduci L, Tarcitano E, Strano S, Yarden Y, Blandino G. CircRnas: role in human diseases and potential use as biomarkers. Cell Death Dis. 2021;12(5). doi:10.1038/s41419-021-03743-3.
- Bai X, Huang Y, Zhang K, Huang W, Mu Y, Li Y, Ouyang H. CircNf1-mediated CXCL12 expression in the spinal cord contributes to morphine analgesic tolerance. Brain Behav Immun. 2022;107:140–151. doi:10.1016/j.bbi.2022.09.018.
- Yang J, Hou G, Chen H, Chen W, Ge J. Circ_0000189 promotes the malignancy of Glioma cells via regulating miR-192-5p-ZEB2 Axis. Oxid Med Cell Longev. 2022;2022:2521951. doi:10.1155/2022/2521951.
- Liu X, Liu B, Zhou M, Fan F, Yu M, Gao C, Lu Y, Luo Y. Circular RNA HIPK3 regulates human lens epithelial cells proliferation and apoptosis by targeting the miR-193a/cryaa axis. Biochem Biophys Res Commun. 2018;503(4):2277–2285. doi:10.1016/j.bbrc.2018.06.149.
- Alhasan A, Izuogu OG, Al-Balool HH, Steyn JS, Evans A, Colzani M, Ghevaert C, Mountford JC, Marenah L, Elliott DJ, et al. Circular RNA enrichment in platelets is a signature of transcriptome degradation. Blood. 2016;127(9):E1–11. doi:10.1182/blood-2015-06-649434.
- Neu CT, Gutschner T, Haemmerle M. Post-transcriptional expression control in platelet biogenesis and function. Int J Mol Sci. 2020;21(20):7614. doi:10.3390/ijms21207614.
- Xia P, Wang S, Ye B, Du Y, Li C, Xiong Z, Qu Y, Fan Z. A circular RNA protects dormant hematopoietic stem cells from DNA sensor cGAS-mediated exhaustion. Immunity. 2018;48(4):688–701 e687. doi:10.1016/j.immuni.2018.03.016.
- Neunert C, Lim W, Crowther M, Cohen A, Solberg L, Jr., Crowther MA. American society of H. The American Society of Hematology 2011 evidence-based practice guideline for immune thrombocytopenia. Blood. 2011;117:4190–4207. doi:10.1182/blood-2010-08-302984. Epub 2011/02/18.
- Neunert CE. Current management of immune thrombocytopenia. Hematology Am Soc Hematol Educ Program. 2013;2013(1):276–282. doi:10.1182/asheducation-2013.1.276. Epub 2013/12/10.
- Varga-Szabo D, Pleines I, Nieswandt B. Cell adhesion mechanisms in platelets. Arterioscler Thromb Vasc Biol. 2008;28(3):403–412. doi:10.1161/ATVBAHA.107.150474. Epub 2008/01/05.
- Zuo B, Zhai J, You L, Zhao Y, Yang J, Weng Z, Dai L, Wu Q, Ruan C, He Y. Plasma microRnas characterising patients with immune thrombocytopenic purpura. Thromb Haemost. 2017;117(07):1420–1431. doi:10.1160/TH-16-06-0481. Epub 2017/04/21.
- Grozovsky R, Begonja AJ, Liu K, Visner G, Hartwig JH, Falet H, Hoffmeister KM. The Ashwell-Morell receptor regulates hepatic thrombopoietin production via JAK2-STAT3 signaling. Nat Med. 2015;21(1):47–54. doi:10.1038/nm.3770.
- Lambert MP, Gernsheimer TB. Clinical updates in adult immune thrombocytopenia. Blood. 2017;129(21):2829–2835. doi:10.1182/blood-2017-03-754119. Epub 2017/04/19.
- Wang Y, Pang N, Wang X, Liu Y, Wang X, Wang L, Sun M, Yasen H, Zhao F, Fan W, et al. Percentages of PD-1(+)CD4(+)T cells and PD-L1(+)DCs are increased and sPD-1 level is elevated in patients with immune thrombocytopenia. Hum Vaccin Immunother. 2018;14(4):832–838. doi:10.1080/21645515.2017.1342913. Epub 2018/01/16.
- Birtas Atesoglu E, Tarkun P, Demirsoy ET, Geduk A, Mehtap O, Batman A, Kaya F, Cekmen MB, Gulbas Z, Hacihanefioglu A. Soluble Programmed Death 1 (PD-1) is decreased in patients with Immune Thrombocytopenia (ITP): Potential Involvement of PD-1 pathway in ITP immunopathogenesis. Clin Appl Thromb Hemost. 2016;22(3):248–251. doi:10.1177/1076029614562952. Epub 2014/12/17.
- Zhong J, Chen S, Xu L, Lai J, Liao Z, Zhang T, Yu Z, Lu Y, Yang L, Wu X, et al. Lower expression of PD-1 and PD-L1 in peripheral blood from patients with chronic ITP. Hematology. 2016;21(9):552–557. doi:10.1080/10245332.2016.1155347. Epub 2016/04/15.
- Lownik JC, Luker AJ, Damle SR, Cooley LF, El Sayed R, Hutloff A, Pitzalis C, Martin RK, El Shikh MEM, Conrad DH. ADAM10-mediated ICOS ligand shedding on B cells is necessary for proper T cell ICOS regulation and T follicular helper responses. J Immunol. 2017;199(7):2305–2315. doi:10.4049/jimmunol.1700833. Epub 2017/08/18.
- Qiao J, Luo Q, Liu N, Wei G, Wu X, Lu J, Tang K, Wu Y, Zi J, Li X, et al. Increased ADAM10 expression in patients with immune thrombocytopenia. Int Immunopharmacol. 2018;55:63–68. doi:10.1016/j.intimp.2017.12.004. Epub 2017/12/11.
- Burenbatu, Borjigin M, Eerdunduleng, Huo W, Gong C, Hasengaowa, Zhang G, Longmei, Li M, Zhang X, et al. Profiling of miRNA expression in immune thrombocytopenia patients before and after Qishunbaolier (QSBLE) treatment. Biomed Pharmacother. 2015;75:196–204. doi:10.1016/j.biopha.2015.07.022. Epub 2015/08/25.
- Qian BH, Ye X, Zhang L, Sun Y, Zhang JR, Gu ML, Qin Q, Chen J, Deng AM. Increased miR-155 expression in peripheral blood mononuclear cells of primary immune thrombocytopenia patients was correlated with serum cytokine profiles. Acta Haematol. 2015;133(3):257–263. doi:10.1159/000362150. Epub 2014/11/22.
- Zhao H, Li H, Du W, Zhang D, Ge J, Xue F, Zhou Z, Yang R. Reduced MIR130A is involved in primary immune thrombocytopenia via targeting TGFB1 and IL18. Br J Haematol. 2014;166(5):767–773. doi:10.1111/bjh.12934. Epub 2014/05/08.
- Chang Y, Chen X, Tian Y, Gao X, Liu Z, Dong X, Wang L, He F, Zhou J. Downregulation of microRNA-155-5p prevents immune thrombocytopenia by promoting macrophage M2 polarization via the SOCS1-dependent PD1/PDL1 pathway. Life Sci. 2020;257:118057. doi:10.1016/j.lfs.2020.118057. Epub 2020/07/08.
- Li JQ, Tian JM, Fan XR, Wang ZY, Ling J, Wu XF, Yang FY, Xia YL. miR-106b-5p induces immune imbalance of Treg/Th17 in immune thrombocytopenic purpura through NR4A3/Foxp3 pathway. Cell Cycle. 2020;19(11):1265–1274. doi:10.1080/15384101.2020.1746485. Epub 2020/04/24.
- Wang Y, Guo Y, Zhang X, Zhao H, Zhang B, Wu Y, Zhang J. The role and mechanism of miR-557 in inhibiting the differentiation and maturation of megakaryocytes in immune thrombocytopenia. RNA Biol. 2021;18(11):1953–1968. doi:10.1080/15476286.2021.1884783. Epub 2021/02/16.
- Julious SA, Walters SJ. Estimating effect sizes for health-related quality of life outcomes. Stat Methods Med Res. 2014;23(5):430–439. doi:10.1177/0962280213476379. Epub 2013/02/22.
- Li H, Hao Y, Zhang D, Fu R, Liu W, Zhang X, Xue F, Yang R. Aberrant expression of long noncoding RNA TMEVPG1 in patients with primary immune thrombocytopenia. Autoimmunity. 2016;49(7):496–502. doi:10.3109/08916934.2016.1167192. Epub 2016/04/07.
- Li JQ, Hu SY, Wang ZY, Lin J, Jian S, Dong YC, Wu XF, Dai L, Cao LJ. Long non-coding RNA MEG3 inhibits microRNA-125a-5p expression and induces immune imbalance of Treg/Th17 in immune thrombocytopenic purpura. Biomed Pharmacother. 2016;83:905–911. doi:10.1016/j.biopha.2016.07.057. Epub 2016/10/25.
- Li J, Tian J, Lu J, Wang Z, Ling J, Wu X, Yang F, Xia Y. LncRNA GAS5 inhibits Th17 differentiation and alleviates immune thrombocytopenia via promoting the ubiquitination of STAT3. Int Immunopharmacol. 2020;80:106127. doi:10.1016/j.intimp.2019.106127. Epub 2020/01/25.
- Huang Y, Qiao Y, Zhao Y, Li Y, Yuan J, Zhou J, Sun H, Wang H. Large scale RNA-binding proteins/LncRNAs interaction analysis to uncover lncRNA nuclear localization mechanisms. Brief Bioinform. 2021;22(6): bbab195. doi:10.1093/bib/bbab195.
- Winkle M, El-Daly SM, Fabbri M, Calin GA. Noncoding RNA therapeutics — challenges and potential solutions. Nat Rev Drug Discov. 2021;20(8):629–651. doi:10.1038/s41573-021-00219-z.