Abstract
Antigenotoxic properties of plant- and plant-like derived foods may embody nutritional strategies against DNA damage. Marine macroalgae have shown DNA-protective effects, but their nutraceutical potential and the influence of growing conditions on these properties is underexplored. Hence, we aimed to assess the genoprotection potential of wild-harvested vs. aquacultured Ulva rigida on Drosophila melanogaster, following a dietary exposure, in the presence and absence of a genotoxic agent (streptonigrin) using phytochemical profiling. DNA damage was evaluated with a single cell gel electrophoresis (comet) assay improved with endonucleases. An origin-based phytochemical profile was found, with aquacultured algae showing higher relative amounts of fatty alcohols, sterols, sesquiterpenoids and glycerol esters. Although U. rigida from both sources showed a DNA-protective action, especially against streptonigrin-induced genotoxicity, aquacultured algae demonstrated higher potential, which may be linked to the distinctive phytochemical profile. Overall, this study provided scientific evidence for the genoprotective activity of U. rigida and its confirmation as a functional food.
Introduction
The human diet has been identified as a risk factor for the development of 30–35% of cancer cases (Ruiz & Hernández, Citation2014), but also as a source of compounds with DNA protection ability, against both endogenous and environmental challenges (Johnson & Fenwick, Citation2000). In recent decades, hundreds of DNA-protective and anticarcinogenic properties have been detected in several plant- and plant-like derived foods (DFG, Citation2000; Knasmüller et al., Citation2002). In this framework, the consumption of marine macroalgae has been associated with several properties valuable to human health (Mohamed et al., Citation2012; Wells et al., Citation2017). Macroalgae have been defended as a functional food by diverse authors (Holdt & Kraan, Citation2011; Mohamed et al., Citation2012; Tanna & Mishra, Citation2018), especially considering their antioxidant, antigenotoxic and anticarcinogenic properties (Athukorala et al., Citation2006; Yuan & Walsh, Citation2006; Celikler et al., Citation2008; Zubia et al., Citation2009; Valentão et al., Citation2010; Marques et al., Citation2018). Moreover, the strengthening of genome integrity underlying these capacities might clarify why human populations whose diet includes macroalgae as a significant element, namely in Asia, present minor prevalence of diet-related diseases (e.g. cardiovascular diseases and cancer), as demonstrated in diverse epidemiological studies (Yamori et al., Citation2001; Pisani et al., Citation2002; Yuan & Walsh, Citation2006).
The green algae (Chlorophyta) constitute one of the most diverse groups of algae, with more than 6600 species, capable of growing in a great diversity of habitats (Guiry & Guiry, Citation2019). The genus Ulva includes over 130 species (Guiry & Guiry, Citation2019) and has a wide distribution in marine, freshwater and brackish environments throughout the world (Canter-Lund & Lund, Citation1995; Hoek et al., Citation1995; Martins et al., Citation1999; McAvoy & Klug, Citation2005; Shimada et al., Citation2007). Among those species, U. rigida, known as sea lettuce, is one of the most studied. This edible macroalga (Guiry & Guiry, Citation2019) has a rich nutritional composition, consisting of polyunsaturated fatty acids, proteins, dietary fibres, sulphated polysaccharides, vitamins, minerals, phenolic compounds and pigments (Celikler et al., Citation2009, Citation2014; Taboada et al., Citation2010; Tabarsa et al., Citation2012; Yildiz et al., Citation2012; Mezghani et al., Citation2016).
Although poorly explored, there is some evidence that Ulva species can provide genoprotection. Ethanolic and aqueous extracts of U. lactuca have shown potential in diminishing the effects of γ-ionizing irradiation on rat livers, namely through the reduction of H2O2 formation, DNA fragmentation and micronuclei frequency (Alam et al., Citation2016). Moreover, crude extracts of U. rigida have shown antigenotoxic and anticlastogenic properties in human lymphocytes cultured in vitro, through the significant decrease of chromosomal aberrations and the frequency of both sister chromatid exchanges and micronuclei induced by mitomycin-C (Celikler et al., Citation2008). The same research group also found that an ethanolic extract of U. rigida diminished the micronuclei frequency in diabetic (Celikler et al., Citation2009) and hypothyroidic rats (Celikler et al., Citation2014). In parallel, an aqueous-ethanolic extract of U. fasciata prevented the formation of micro-nucleated polychromatic erythrocytes induced by benzo[a]pyrene in rats (Rodeiro et al., Citation2015). Sathivel et al. (Citation2014) found that a sulphated polysaccharide isolated from U. lactuca alleviates DNA fragmentation and necrosis in rats with D-galactosamine induced liver damage. Yet, most of these studies are solely evaluations of extracts or compounds isolated from the algae or in vitro trials, which means there is no knowledge of in vivo effects of whole macroalgae ingestion on the genome integrity preservation. As defended by the European Advisory Services (Citation2008) and echoed by Holdt & Kraan (Citation2011), functional food should not be consumed as pills or capsules, but instead remain food and be consumed in realistic amounts. Furthermore, only a few studies have addressed U. rigida regarding its DNA-protective properties, which, combined with factors such as edibility, wide geographic distribution and ease of being cultivated in aquaculture, makes it one of the most relevant Ulva species to study.
Environmental factors, such as light, temperature and salinity, have been shown to directly influence the variety and quantity of algal compounds (Marinho-Soriano et al., Citation2006). This legitimizes the hypothesis that the genome protective properties described for Ulva species may be indirectly influenced by the growing conditions (Lahaye et al., Citation1995; Gómez-Pinchetti et al., Citation1998; Abreu et al., Citation2009), which can be particularly pronounced when considering natural environments vs. conditions occurring under manipulated/controlled aquaculture scenarios. Nevertheless, to the authors’ knowledge, this is an underexplored perspective within the context of macroalgal genoprotective potential, despite being previously suggested by Marques et al. (Citation2018).
Considering the identified knowledge gaps and keeping in view the phytochemical and functional characterization of healthy foods, the present study was designed with the major goal of assessing the properties of U. rigida to strengthen genome protection. Hence, the antigenotoxic potential of this green alga was evaluated in Drosophila melanogaster (a model organism recurrently adopted in genotoxicity/antigenotoxicity studies (e.g. Carmona et al., Citation2011; Fernández-Bedmar et al., Citation2011)), following dietary exposure, both in the presence and absence of streptonigrin which is a well-known genotoxic/mutagenic agent (Gaivão et al., Citation1999; Bolzán & Bianchi, Citation2001). Hence, DNA damage in the flies’ neuroblast cells was evaluated by the single cell gel electrophoresis (comet) assay, improved with nucleoid digestion by endonucleases FPG formamidopyrimidine DNA glycosylase (FPG) and endonuclease III (EndoIII) to detect oxidized purines and pyrimidines, respectively. Thus, the discrimination of defence properties in relation to specific DNA damaging events is possible. Furthermore, the comparative evaluation of wild-harvested vs. aquacultured U. rigida antigenotoxic potential was supported by the respective phytochemical profiling of hexane and ethanolic algal extracts.
Materials and methods
Chemicals
The instant treatment Carolina Drosophila Medium Formula 4-24® (hereinafter referred as Instant Drosophila Medium; IDM) was purchased from Carolina Biological Supply Company (USA). Streptonigrin (CAS 3930-19-6) was obtained from Santa Cruz Biotechnology Inc. (California, USA). DNA lesion-specific repair enzymes, namely FPG and EndoIII, were purchased from Professor Andrew Collins (University of Oslo, Norway). Solvents (of analytical grade or bi-distilled commercial products) were purchased from Panreac AppliChem (Germany) and Acros Organics, Fisher Scientific (USA). All other chemicals were obtained from Sigma Aldrich Chemical Company (Spain).
Macroalgae harvesting and preparation
Ulva rigida from wild origin (hereinafter referred to as U1) was collected, in September 2015, at the Mindelo beach, Vila do Conde, Portugal (41°18’36.8”N, 8°44’25.9”W), a location with no significant pollution sources identified (Reis et al., Citation2014). Ulva rigida grown in aquaculture (hereinafter referred to as U2) was obtained from an integrated multi-trophic aquaculture (IMTA) system at ALGAplus, Lda. (certified organic production; Ílhavo, Portugal) and harvested in September 2015.
The wild-harvested and aquacultured batches of macroalgae were prepared as described in Marques et al. (Citation2018). Prior to the analyses and experiments, U. rigida from both origins was ground with a coffee mill, obtaining particles with a maximum surface area of ~1–2 mm2.
Species identification of both wild-harvested and aquacultured U. rigida specimens was done with DNA-barcoding as described in Marques et al. (Citation2018).
Macroalgae phytochemical characterization
Extract preparation
Algal material (U1 – 15.012 g; U2 – 15.004 g) was extracted in the dark, at room temperature, under agitation, for 72 h with hexane, renewing the solvent twice. After evaporation of the solvent from the 3 combined extractions using a rotary vacuum evaporator, 0.142 and 0.141 g were retained from U1 and U2 samples, respectively. The extraction yields of hexane extracts of U1 and U2 were 0.95 and 0.94%, respectively. Afterwards, the remaining algal material was reextracted following the same protocol with ethanol, renewing the solvent twice. After rotary evaporation of the solvent from the combined extractions, 0.242 and 0.333 g were retained from U1 and U2 ethanolic extracts (extraction yields were 1.61 and 2.22%, respectively).
Gas chromatography-mass spectrometry (GC-MS)
Three replicates of each dried hexane extract of U1 and U2 (~20 mg each) were dissolved in 0.5 ml of dichloromethane, and the internal standard (tetracosane) was added (30 mg ml–1 in dichloromethane). This mixture was silylated by adding 250 μl of pyridine, 250 μl of BSTFA and 50 μl of TMSCl, and then heated at 70ºC for 1 h, followed by immediate injection into the GC-MS (Faustino et al., Citation2017). GC-MS analyses were performed on a GC-MS QP2010 Ultra Shimadzu. The separation of compounds was carried out in a DB-5 J&W capillary column (30 m × 0.25 mm inner diameter, 0.25 μm film thickness) using helium as the carrier gas (35 cm s−1). The chromatographic conditions were as described by Rahmouni et al. (Citation2018).
The peaks from the total ion chromatogram were identified by comparing their mass spectra with the equipment mass spectral library (NIST 14 Mass Spectral and Wiley Registry of Mass Spectral Data), with MS spectra and MS fragmentation pattern described in the literature (Petersson, Citation1969; Füzfai et al., Citation2008; Razboršek et al., Citation2008; Hrabovski et al., Citation2012; Isca et al., Citation2014; Suttiarporn et al., Citation2015; AOCS, Citation2019; Golm Metabolome Database, Citation2019) and by comparing the retention times and mass spectra data of the standard compounds injected in the same chromatographic conditions. Moreover, when authentic standard is not available, the retention index relative to n-alkanes (C5–C36) was compared with retention indexes reported by NIST 14 database (when available).
Ultra-high-performance liquid chromatography-mass spectrometry (UHPLC-MS)
For the UHPLC-MS analysis, U1 and U2 ethanolic dried extracts (nearly 30 mg each) were dissolved in methanol (1 ml) and the resulting solution was filtered through a 0.2 µm Nylon membrane (Whatman). The analysis was performed using a Thermo Scientific Ultimate 3000RSLC (Dionex) equipped with a Dionex UltiMate 3000 RS diode array detector and coupled to a mass spectrometer. The column used was a Thermo Scientific Hypersil GOLD column (1000 × 20 mm) with a particle size of 1.9 µm and the temperature maintained at 32ºC. The mobile phase was composed of (A) 0.1% formic acid (v/v) and (B) acetonitrile/methanol (70:30). The solvent gradient started with 85% of solvent B over 3.9 min, followed by a 6.1 min upgrade until 100% of solvent B maintained for 25.3 min, then in 31 min lowered to 85% of solvent B, which was maintained for 38 min. The injection volume was 2 µl. UV-Vis spectral data were gathered in a range of 250–680 nm and the chromatographic profiles were documented at 430, 450 and 650 nm. The mass spectrometer used was an LTQ XL linear ion trap 2D equipped with an orthogonal electrospray ion source (ESI). The equipment was operated in negative-ion mode with electrospray ionization source of 5.00 kV and ESI capillary temperature of 275°C. The full scan covered a mass range of 50–2000 m/z. Collision-induced dissociation MS/MS and MSn experiments were simultaneously acquired for precursor ions.
Test organisms and experimental design
Stock cultures of D. melanogaster (Oregon-K strain) were maintained in a chamber at 25°C, with relative humidity of ~60%, in culture vials containing 20 ml of standard lab-made growth medium (10% sucrose, 10% yeast, 1.2% agar-agar, 0.2% NaCl and 0.5% propionic acid in water). Considering that this growth medium needs to be heated to 90°C, IDM was used in the experimental trials to prevent eventual denaturation of macroalgae bioactive components, as it does not need to be heated or sterilized.
Considering the choice of assessing the algae antigenotoxic potential on flies’ neuroblasts (the precursor cells of D. melanogaster brain), the assay was carried out on third-instar larvae, as described in Sierra et al. (Citation2014). Hence, to obtain those larvae, groups of 20 couples were mass-crossed in glass culture vials with standard growth medium for 2 days to increase fertility. Then, couples were transferred to new culture vials containing 20 ml of IDM (three per experimental condition, with 20 couples each). Flies were divided into two major groups: one unchallenged and another challenged with streptonigrin (S) (). Each major group was divided into two subgroups, corresponding to wild-harvested and aquacultured U. rigida supplementations. For each subgroup, two levels of supplementation (2.5 and 5%) and a control group (with no alga supplementation) were tested. Supplementation levels were expressed as percentage of alga relatively to IDM (weight/weight) to enable a better perception of the tested amount in relation to the total food intake. This option was previously elected in diverse studies with comparable goals (Sousa et al., Citation2009; Rezende et al., Citation2013). The levels of algal supplementation (2.5 and 5%) were selected based on the results of a preliminary study of our research team (Supplementary table 1), in which a broader range of alga concentrations (1.25–20.0%) were tested and the prolificacy (number of hatched individuals) per condition was recorded. The selection of the supplementation levels to test in the present study relied on the identification of the two lowest algal percentages, showing the higher prolificacy. Streptonigrin was added to IDM, dissolved in PBS, achieving the final concentration of 20 μM, selected according to the literature (Gaivão et al., Citation1999).
Fig. 1. Schematic representation of the experimental design, depicting the division of Drosophila melanogaster individuals into two major groups: one unchallenged and another streptonigrin-challenged (S). Each of the previous groups was divided into two subgroups, corresponding to wild-harvested and aquacultured Ulva rigida (U) trials (depicted in the groups codes 1 and 2, respectively). For each subgroup, two levels of supplementation (2.5 and 5%; represented in the groups codes by the number preceding the letter U) and a control group (with no alga supplementation; C or S) were tested
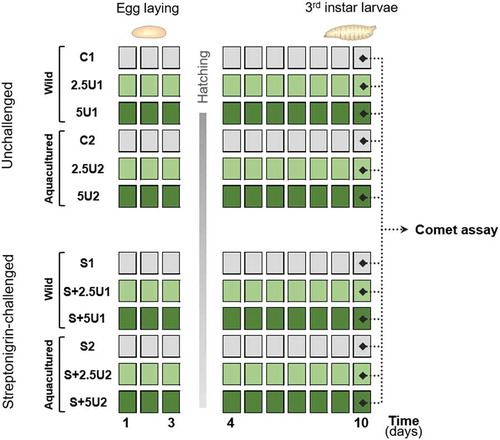
Mated flies laid eggs for 3 days, after which adults (F0) were discarded. Vials were kept at 25°C and, according to the D. melanogaster life cycle, it was expected that the feeding larvae (F1) were exposed to the alga/streptonigrin via ingestion for approximately between 5 and 8 days (). After this period, neuroblasts were excised in the third-instar stage individuals, before larvae reach the pupa stage. Briefly, from each triplicate (glass vial), four pools with two larvae each were assembled. For each pool, the brain ganglia were removed from the larvae immersed in Ringer solution under a stereo microscope with diascopic illumination (Leica Wild M3Z) with the help of two dissection needles. Thus, the brain ganglia were placed in microtubes with 20 µl of Ringer solution and maintained at 4°C. The suspension was then centrifuged at 1.7 g for 5 min (Thermo Scientific Sorvall Legend 14 microcentrifuge), after which the pellet composed of the brain ganglia was shredded with a dissection needle to release neuroblast cells and obtain a cell suspension.
Comet assay
The alkaline version of the comet assay was performed following the methods of Collins (Citation2004), as adapted by Guilherme et al. (Citation2012). The previously mentioned cell suspension was mixed with 140 µl of 1% low melting point agarose (in PBS). Then, two drops of 6 μl (per pool) were placed vertically onto the glass microscope slides, precoated with 1% normal melting point agarose, without coverslips. Each slide confined 12 mini-gels, as six columns of two. Then, slides were left ± 5 min at 4°C to solidify the agarose, immersed in a lysis solution (2.5 M NaCl, 0.1 M EDTA, 10 mM Tris, 1% Triton X-100, pH 10) and kept overnight at 4°C. After lysis of agarose-embedded cells, slides were washed three times with enzyme buffer (0.1 M KCl, 0.5 mM EDTA, 40 mM HEPES, 0.2 mg ml−1 bovine serum albumin, pH 8) at 4°C. Three sets of slides were prepared. Two sets were incubated with endonucleases (1) FPG and (2) EndoIII that convert oxidized purines and pyrimidines into DNA single strand breaks, respectively (Azqueta et al., Citation2009). The third set (3) was incubated only with the enzyme buffer. Hence, 30 μl of each enzyme diluted in enzyme buffer (and only buffer in the third set) was applied to each mini-gel, with coverslip, and the slides were incubated at 37°C for 30 min in a humidified chamber. Then, slides were immediately placed in the electrophoresis tank, immersed in electrophoresis solution (0.3 M NaOH, 1 mM EDTA, pH >13) for 20 min (alkaline treatment). Electrophoresis was performed for 20 min at a fixed voltage of 17 V and a current of 300 mA, which results in ~0.7 V cm−1 (achieved by adjusting the buffer volume in the electrophoresis tank). Following the electrophoresis, slides were neutralized with PBS (10 min, 4ºC) and with distilled water (10 min, 4°C). Finally, slides were fixed in absolute ethanol for 15 min, air-dried and stored at room temperature.
Slides were stained with ethidium bromide (20 μg ml−1) and 50 nucleoids per mini-gel were observed, using an Olympus BX 41 fluorescence microscope (400× magnification). The nucleoids were classified by visual scoring into 5 comet classes, according to the tail length and intensity from 0 (no tail) to 4 (almost all DNA in tail) (Collins, Citation2004). The final score (expressed as ‘arbitrary units’ in a range of 0–400) was obtained by multiplying the mean percentage of nucleoids in each class by the corresponding factor, according to this formula:
Genetic Damage Indicator (GDI) = [(% nucleoid class 0) × 0)] +
[(% nucleoid class 1) × 1)] + [(% nucleoid class 2) × 2)] +
[(% nucleoid class 3) × 3)] + [(% nucleoid class 4) × 4)]
The GDI and the parameters GDIFPG and GDIEndoIII were determined. Additionally, to assess the DNA breaks corresponding specifically to the net enzyme-sensitive sites (NSS), the scores obtained with GDI slides (lacking enzyme treatment) were subtracted to GDIFPG and GDIEndoIII values resulting in the parameters expressed as NSSFPG and NSSEndoIII, respectively.
Statistical analysis
Statistica 8.0 (StatSoft, Inc., USA) and Microsoft Excel (Microsoft Corporation, USA) software were used to perform the statistical analysis. First, data were tested for normality (Shapiro–Wilk test and graphical analysis), homogeneity of variances (Levene test) and data transformation was performed when those statistical assumptions were not met. Then, a 3-way ANOVA (factors: origin of algae, streptonigrin challenge and level of algae supplementation) was used to analyse significant effects and interaction between factors (simple main effects were assessed for each factor if this occurred). Finally, each ANOVA was followed by a post hoc Tukey test. Differences were considered significant when p < 0.05 (Zar, Citation1996).
Results
Ulva rigida phytochemical profiling
Phytochemical characterization allowed a semi-quantitative determination of several compounds from hexane and ethanolic extracts of wild-harvested and aquacultured U. rigida (U1 and U2 specimens). Five classes of compounds were identified in the hexane extracts: fatty acid derivatives, fatty alcohols, sterols, sesquiterpenoids and glycerol esters (). The first class was the most represented, with palmitic acid being the most abundant compound, regardless of the alga’s origin. Aquacultured U. rigida (U2) presented higher relative amounts of all compounds identified, particularly fatty alcohols, sterols, sesquiterpenoids and glycerol esters classes.
Table 1. Compounds identified from the hexane extracts of wild-harvested and aquacultured Ulva rigida
The metabolites identified in the ethanolic fractions belong to two classes: porphyrinolactones and chlorophylls, the latter being the richer group (). Despite some differences on the relative quantity of the metabolites between U1 and U2, origin-based patterns were not markedly visible in ethanolic profiles.
Table 2. Compounds identified from ethanolic extracts of wild-harvested and aquacultured Ulva rigida, and their molecular ions species and fragments (m/z) data
Evaluation of DNA damage
Slight variations were found on the GDI parameter of the unchallenged groups (), since the lower supplementation level of wild-harvested U. rigida (2.5U1) reduced GDI, either compared to the respective unsupplemented control or to 5U1 groups. In contrast, the lowest supplementation level of aquacultured U. rigida (2.5U2) induced an increase of GDI, in comparison to the respective unsupplemented control and 2.5U1 groups. All groups exposed to streptonigrin showed an increase of GDI in comparison to the unchallenged counterparts. Streptonigrin-challenged groups fed with wild-harvested U. rigida (regardless of the supplementation level) showed a reduction on GDI relative to the respective unsupplemented control group. Flies fed with both supplementation levels of aquacultured U. rigida also showed lower GDI than the unsupplemented control group, S+5U2 group presenting less DNA damage than S+2.5U2. Origin-based differences were noticed between groups S+5U1 and S+5U2, since the latter showed a decrease on GDI relative to the former.
Fig. 2. Mean values of DNA damage (GDI) in Drosophila melanogaster neuroblasts after a supplementation with Ulva rigida (2.5 and 5%; number preceding the letter U) from wild-harvesting (U1) and aquaculture (U2) origins. C and S represent the unsupplemented unchallenged and streptonigrin-challenged groups, respectively. Bars represent the standard error. Statistically significant differences between groups submitted to the supplementation with U. rigida of the same origin, under the same genotoxic challenge are marked by different letters; differences between U1 and U2 groups under the same genotoxic challenge and level of supplementation are marked by black diamond (♦); differences between groups unchallenged and streptonigrin-challenged, under the same level of supplementation, from the same origin are marked by asterisk (*)
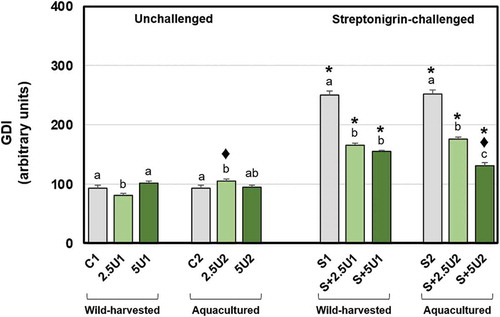
Regarding GDIFPG data (), the unchallenged group fed with the higher supplementation level of wild-harvested U. rigida (5U1) showed higher levels, relative to the respective unsupplemented control and 2.5U1 groups. Drosophila melanogaster fed with both supplementation levels of aquacultured U. rigida showed higher GDIFPG than the respective unsupplemented control group. Moreover, 2.5U2 group showed higher GDIFPG than 2.5U1, while the opposite pattern was seen comparing the higher supplementation level groups (5U). Streptonigrin-challenged groups (except S+5U1) showed higher GDIFPG values in comparison to the unchallenged ones. Flies fed with both supplementation levels of both U. rigida origins showed a reduction on GDIFPG parameter in comparison with the respective unsupplemented groups. A difference regarding the supplementation level was also found concerning aquacultured U. rigida, since flies fed with the higher supplementation showed lower damage. Moreover, an origin-based difference was evident concerning the lower U. rigida supplementation level, since S+2.5U2 group showed higher GDIFPG values compared with S+2.5U1.
Fig. 3-4. Mean values of oxidative purine DNA damage in Drosophila melanogaster neuroblasts after a supplementation with Ulva rigida (2.5 and 5%; number preceding the letter U) from wild-harvesting (U1) and aquaculture (U2) origins. C and S represent the unsupplemented unchallenged and streptonigrin-challenged groups, respectively. (Fig. 3) overall damage (GDIFPG) and partial scores, i.e. GDI plus additional DNA breaks corresponding to net FPG-sensitive sites (NSSFPG; dark grey diagonal stripes); (Fig. 4) NSSFPG alone. Bars represent the standard error. Statistically significant differences between groups submitted to the supplementation with U. rigida of the same origin, under the same genotoxic challenge are marked by different letters; differences between U1 and U2 groups under the same genotoxic challenge and level of supplementation are marked by black diamond (♦); differences between groups unchallenged and streptonigrin-challenged, under the same level of supplementation, from the same origin are marked by asterisk (*)
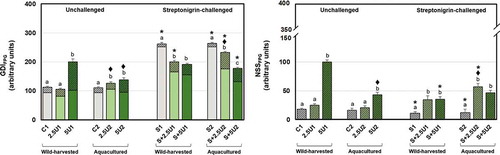
Regarding the NSSFPG parameter (), unchallenged 5U1 group showed higher values of GDI than the unsupplemented or 2.5U1 groups. The same pattern was found concerning the aquacultured U. rigida specimen, although to a lesser extent. Flies of 5U2 group showed less NSSFPG than the reciprocal group fed with wild-harvested alga. Unsupplemented groups exposed to the genotoxic showed lower NSSFPG in comparison to non-exposed ones, as also did the group S+5U1 compared with the 5U1 group. By contrast, the group exposed to streptonigrin and with a diet supplemented with 2.5% of aquacultured alga (S+2.5U2) showed higher NSSFPG values than the reciprocal unchallenged group. Concerning only streptonigrin-challenged groups, both alga supplementation levels (2.5 and 5%) showed higher NSSFPG in comparison to the respective unsupplemented groups, regardless of its origin. Moreover, an origin-based difference was found between groups S+2.5U1 and S+2.5U2, the latter showing higher NSSFPG values than the former.
Analysing GDIEndoIII data (), the unchallenged group fed with 5% of wild-harvested U. rigida (5U1) showed higher GDIEndoIII values relative to the unsupplemented group. A similar pattern was noticed for aqua-cultured alga supplementation, since 5U2 revealed higher GDIEndoIII than unsupplemented and 2.5U2 groups, while the latter also showed lower GDIEndoIII than 2.5U1, highlighting an origin-based difference. All streptonigrin-challenged flies showed an increase of GDIEndoIII levels in comparison to unchallenged ones. Both supplementation levels of U. rigida, of both origins, showed lower GDIEndoIII than the respective unsupplemented groups. Moreover, S+5U2 group revealed lower values of that parameter, either compared with S+2.5U2 or S+5U1 groups.
Fig. 5-6. Mean values of oxidative pyrimidine DNA damage in Drosophila melanogaster neuroblasts after a supplementation with Ulva rigida (2.5 and 5%; number preceding the letter U) from wild-harvesting (U1) and aquaculture (U2) origins. C and S represent the unsupplemented unchallenged and streptonigrin-challenged groups, respectively. (Fig. 5) overall damage (GDIEndoIII) and partial scores, i.e. GDI plus additional DNA breaks corresponding to net EndoIII-sensitive sites (NSSEndoIII; dark grey diagonal stripes); (Fig. 6) NSSEndoIII alone. Bars represent the standard error. Statistically significant differences between groups submitted to the supplementation with U. rigida of the same origin, under the same genotoxic challenge are marked by different letters; differences between U1 and U2 groups under the same genotoxic challenge and level of supplementation are marked by black diamond (♦); differences between groups unchallenged and streptonigrin-challenged, under the same level of supplementation, from the same origin are marked by asterisk (*)
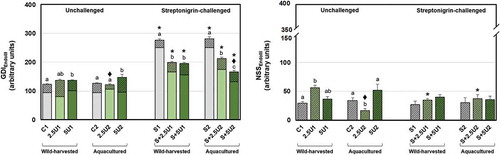
Regarding the NSSEndoIII parameter (), group 2.5U1 showed higher values, in comparison to the respective unsupplemented group. In contrast, the group 2.5U2 showed lower NSSEndoIII values relative to the unsupplemented and 5U2 groups, as well as in comparison with 2.5U1. Considering the genotoxic challenge inflicted by streptonigrin, S+2.5U1 showed lower NSSEndoIII levels than the corresponding unchallenged group, while the opposite was evident considering the same alga supplementation level of aquacultured alga.
Discussion
Origin-related phytochemical profiling of U. rigida
The phytochemical profile of wild-harvested and aquacultured U. rigida revealed an origin-based differential pattern, especially concerning lipophilic compounds (fatty alcohols, sterols, sesquiterpenoids and glycerol esters classes). In turn, fatty acid derivatives, as well as polar phytocompounds (porphyrinolactone- and chlorophyll-related molecules) did not reveal a distinct origin-based pattern. Nevertheless, the origin-based pattern observed may be explained by the higher fluctuations of the growing conditions to which the wild-harvested specimens are exposed, namely environmental factors such as water temperature, light and salinity, as well as nutrients and minerals availability (varying according to season and location). These factors are known to directly modulate the variety and quantity of macroalgae constituents (Marinho-Soriano et al., Citation2006). Moreover, U. rigida from aquaculture was farmed on a certified organic IMTA system, i.e. the macroalga could assimilate fish-excreted ammonia and/or nitrate, phosphate and CO2, converting them into potentially valuable biomass (Abreu et al., Citation2011). Indeed, the cultivation of seaweeds in IMTA systems allows higher productivity levels, promoting less variability than the natural seaweed beds due to higher and more constant nutrients availability (Abreu et al., Citation2009).
Streptonigrin-induced DNA damage
Although not the main purpose of the current study, it is important to address streptonigrin-induced genotoxicity, as formerly stated by some authors (Gaivão et al., Citation1999; Bolzán & Bianchi, Citation2001), namely to confirm the generation of an external genotoxic pressure that, hypothetically, can be counteracted by the macroalgae supplementation.
Regarding the non-specific DNA damage evaluated through the standard alkaline comet assay, D. melanogaster individuals exposed to streptonigrin showed higher levels of DNA damage (single and/or double strand breaks), in agreement with previous studies (Gaivão et al., Citation1999; Bolzán & Bianchi, Citation2001). Indeed, unsupplemented fly groups exposed to that agent (S1 and S2) have more than double the levels of DNA damage. Regarding oxidative DNA damage, the data revealed unexpected details. Hence, S1 and S2 groups revealed lower levels of oxidized purine bases (NSSFPG; sensitive sites converted into DNA breaks by FPG enzyme) than non-exposed groups. This pattern was not perceptible regarding pyrimidine bases (which oxidation would be detected by EndoIII and converted into DNA breaks), demonstrating that purines and pyrimidines are differently affected. Considering that streptonigrin has been described as a pro-oxidant agent (Bolzán & Bianchi, Citation2001), it can be hypothesized that (i) it may induce different types of oxidative DNA lesions not detected by FPG or EndoIII, but also that (ii) the level of DNA damage induced was so significantly strong that most of it may have been already shown in the form of single and/or double strand breaks (and expressed as GDI). It is known that oxidative stress may directly induce DNA strand breaks, beyond the oxidation of the DNA bases (Bertoncini & Meneghini, Citation1995; Lloyd & Phillips, Citation1999; Ciccia & Elledge, Citation2010). This seems plausible especially considering that the D. melanogaster strain adopted in this study (Ok strain) is highly susceptible to ROS attack and presents low activity of antioxidant enzymes (Gaivão & Comendador, Citation1996). In the same direction, the lower or equal level of oxidative DNA damage, measured respectively as NSSFPG and NSSEndoIII parameters in flies treated with streptonigrin compared with the unchallenged ones, should not be attributed to an activation of the antioxidant defences.
Ulva rigida genoprotection potential and association with phytochemical profile
Regarding the antigenotoxic potential of U. rigida against basal non-specific DNA damage, slight and ambivalent variations were found concerning 2.5% supplementation of both alga origins, translated into a damage reduction for 2.5U1 and an increase for 2.5U2. This contradictory pattern must be interpreted cautiously taking into consideration that those subtle variations resulted from a delicate balance between genotoxic and antigenotoxic pressures. The susceptibility of this balance, especially when the genotoxic vs. antigenotoxic potential of certain foods, beverages, extracts or isolated compounds is considered, was formerly proved by several authors, when substances apparently described as beneficial showed a slight genotoxic action (Forester & Lambert, Citation2011; Ho et al., Citation2013; Leandro et al., Citation2013; Alves et al., Citation2014; Oyeyemi et al., Citation2015; Azqueta & Collins, Citation2016).
Of note, previous research revealed that those foods, beverages or compounds able to slightly reduce the DNA integrity baselines in the absence of a genotoxic challenge, frequently displayed antigenotoxic ability against a multiplicity of genotoxic agents (Leandro et al., Citation2013; Campos-Pereira et al., Citation2014). Likewise, in the current study, U. rigida showed its antigenotoxic ability against the streptonigrin-induced DNA damage, also displaying a dose-response effect in the case of aquacultured alga, which is in line with our previous research (Marques et al., Citation2018).
Furthermore, when analysing the oxidative DNA damage, the parameters GDIFPG and GDIEndoIII showed protection profiles identical to that disclosed by GDI, specifically concerning the genoprotection against streptonigrin. On the other hand, surprising details came to light regarding the DNA breaks resulting from the endonucleases activity (NSSFPG and NSSEndoIII parameters). In general, U. rigida from both origins enhanced the DNA breaks resulting from FPG endonuclease activity, either in the absence or presence of streptonigrin. This was evident only in some cases regarding EndoIII endonuclease activity. As mentioned above, genoprotection involves a complex network of processes and should be regarded as a multiphasic action. Accordingly, certain foods, beverages or isolated compounds that are usually described as antioxidants, also display pro-oxidant actions (Podmore et al., Citation1998; Yen et al., Citation2002; Lambert & Elias, Citation2010; Ho et al., Citation2013). That pro-oxidant potential has been shown to be possibly beneficial, since a mild oxidative stress may trigger cell antioxidant defences and xenobiotic-metabolizing enzymes (Halliwell, Citation2008), activating essential cell signalling pathways (Procházková et al., Citation2011), and, ultimately, prevent the development of certain diseases, such as cancer (Lambert & Elias, Citation2010; Forester & Lambert, Citation2011; Carocho & Ferreira, Citation2013). To the authors’ knowledge, no study has investigated the pro-oxidant potential of U. rigida. Nonetheless, diverse studies have indicated a pro-oxidant activity of certain phytocompounds, namely carotenoids (Astorg, Citation1997; El-Agamey et al., Citation2004), vitamin C (Podmore et al., Citation1998), polyphenols (Halliwell, Citation2008) (previously determined on U. rigida; Yildiz et al., Citation2012) and β-sitosterol (Rosenblat et al., Citation2013) (determined on U. rigida in the current study), as well as unsaturated fatty acids extracted from U. lactuca (Wang et al., Citation2013). Hence, it is reasonable to assume that U. rigida may possess a mild pro-oxidant potential, which probably contributes to its antioxidant action and, indirectly, to the antigenotoxic properties observed in the current and previous studies (Celikler et al., Citation2008, Citation2009, Citation2014; Marques et al., Citation2018). On the other hand, the pro-oxidant potential of those compounds depends on the dose (de Roos & Duthie, Citation2015), as well as on their chemical structure and metal-chelating activity, which will influence their redox properties (Yen et al., Citation2002; El-Agamey et al., Citation2004). Considering that FPG-induced DNA breaks on unchallenged individuals fed with 5% of wild-harvested U. rigida more than doubled regarding the same supplementation level of aquacultured alga, or compared with the groups exposed to streptonigrin, it is not certain that this still corresponds to a beneficial mild pro-oxidant effect as described above.
Bearing in mind algae origin-related specificities, feeding with 5% of aquacultured U. rigida was found to be the treatment which offered higher protection against the genotoxic insult inflicted by streptonigrin, pushing the DNA breaks almost to the unchallenged level, which can be consistently observed on GDI, GDIFPG and GDIEndoIII parameters. This must be related with the higher production of certain phytocompounds in an aquaculture context observed in the current study, resulting from the controlled cultivation conditions of this alga in an organic IMTA system and the genome protective properties ascribed to them. According to the literature, sterols, namely β-sitosterol and campesterol, showed anticarcinogenic (Shahzad et al., Citation2017; Sharmila & Sindhu, Citation2017), antioxidant (Yoshida & Niki, Citation2003) and antigenotoxic (Zeiger & Tice, Citation1997; Paniagua-Pérez et al., Citation2008; Sharmila & Sindhu, Citation2017) properties. In turn, sesquiterpenoids have shown antioxidant, anticarcinogenic and anti-inflammatory potential (Zhang et al., Citation2005; Chakraborty & Paulraj, Citation2010; Ghantous et al., Citation2010; Merfort, Citation2011). Natural pigments, such as chlorophyll, and pigment-related compounds previously showed antioxidant ability (Pangestuti & Kim, Citation2011). Moreover, compounds belonging to the fatty alcohols class, especially octacosanol, displayed antioxidant (Ohta et al., Citation2008) and anti-inflammatory (Guo et al., Citation2017) properties.
In general, the phytocompounds of U. rigida determined in the current study may explain the algal genoprotection properties, as well as the putative mechanisms considered, especially against an exogenous stressor such as streptonigrin. Nevertheless, consideration should be given to the existence of other compounds not determined in the current study (e.g. sulphated polysaccharides, carotenoids, polyphenols), mainly because U. rigida was tested in vivo as a whole food. Thus, the observed effects were probably due, as well, to synergisms between the algal phytocompounds. In that context, and with the aim of examining macroalgae as a functional food, further studies should be pursued, targeting a more comprehensive phytochemical analysis, as well as the unveiling of the genoprotection mechanisms triggered by U. rigida and other edible macroalgae.
Conflict of interests
No potential conflict of interest was reported by the authors.
Supplementary information
The following supplementary material is accessible via the Supplementary Content tab on the article’s online page at http://doi.org/10.1080/09670262.2020.1778796
Supplementary table 1. Drosophila melanogaster prolificacy (number of hatched individuals) registered on the preliminary study for the different levels of supplementation with wild-harvested (U1) and aqua-cultured (U2) Ulva rigida.
Author contributions
A. Marques: macroalgae harvesting, practical work, data analysis, manuscript writing; J. Ferreira: practical work, manuscript editing; H. Abreu and R. Pereira: macroalgae harvesting, identification and dehydration; D. Pinto and A. Silva: phytochemical characterization; I. Gaivão and M. Pacheco: original concept, manuscript editing.
TEJP-2019-0121-File008.docx
Download MS Word (13.3 KB)Acknowledgements
The authors would like to thank Dr Malcolm Purves (professional translator) for the manuscript proofreading and editing.
Supplemental Material
The following supplementary material is accessible via the Supplementary Content tab on the article’s online page at https://doi.org/10.1080/09670262.2020.1778796.
Additional information
Funding
References
- Abreu, M.H., Varela, D.A., Henríquez, L., Villarroel, A., Yarish, C., Sousa-Pinto, I. & Buschmann, A.H. (2009). Traditional vs. integrated multi-trophic aquaculture of Gracilaria chilensis C. J. Bird, J. McLachlan & E. C. Oliveira: productivity and physiological performance. Aquaculture, 293: 211–220.
- Abreu, M.H., Pereira, R., Yarish, C., Buschmann, A. & Sousa-Pinto, I. (2011). IMTA with Gracilaria vermiculophylla: productivity and nutrient removal performance of the seaweed in a land-based pilot scale system. Aquaculture, 312: 77–87.
- Alam, S.S., El-Kader, H.A.M.A., El-Rahim, A.H.A., Hamed, S.M. & Saber, A.A. (2016). The protective role of Ulva lactuca against genotoxic and biochemical effects induced by γ-irradiation in rats. International Journal of Pharmaceutical Sciences Review and Research, 37: 40–48.
- Alves, A.B.C.R., Santos, R.S. dos, Calil, S. de S., Niero, R., Lopes, J. da S., Perazzo, F.F., Rosa, P.C.P., Andrade, S.F., Cechinel-Filho, V. & Maistro, E.L. (2014). Genotoxic assessment of Rubus imperialis (Rosaceae) extract in vivo and its potential chemoprevention against cyclophosphamide-induced DNA damage. Journal of Ethnopharmacology, 153: 694–700.
- AOCS (2019). AOCS Lipid Chemistry, Biology, Technology & Analysis. http://lipidlibrary.aocs.org (accessed 3.11.19).
- Astorg, P. (1997). Food carotenoids and cancer prevention: an overview of current research. Trends in Food Science and Technology, 8: 406–413.
- Athukorala, Y., Kim, K.-N. & Jeon, Y.-J. (2006). Antiproliferative and antioxidant properties of an enzymatic hydrolysate from brown alga, Ecklonia cava. Food and Chemical Toxicology, 44: 1065–1074.
- Azqueta, A. & Collins, A.R. (2016). Polyphenols and DNA damage: a mixed blessing. Nutrients, 8: 1–21.
- Azqueta, A., Shaposhnikov, S. & Collins, A.R. (2009). DNA oxidation: investigating its key role in environmental mutagenesis with the comet assay. Mutation Research, 674: 101–108.
- Bertoncini, C.R. & Meneghini, R. (1995). DNA strand breaks produced by oxidative stress in mammalian cells exhibit 3’-phosphoglycolate termini. Nucleic Acids Research, 23: 2995–3002.
- Bolzán, A.D. & Bianchi, M.S. (2001). Genotoxicity of streptonigrin: a review. Mutation Research, 488: 25–37.
- Campos-Pereira, F.D., Veiga‐Menoncello, A.C.P. & Marin-Morales, M.A. (2014). DNA damage induced by diet. InToxic Effects of Chemicals in Food, Chemical and Consumer Product Safety (Severi-Aguiar, G.D.C. & Alves, A.A., editors), 43–59. Research Signpost.
- Canter-Lund, H. & Lund, J. (1995). Freshwater Algae: Their Microscopic World Explored. Biopress.
- Carmona, E.R., Creus, A. & Marcos, R. (2011). Genotoxicity testing of two lead-compounds in somatic cells of Drosophila melanogaster. Mutation Research, 724: 35–40.
- Carocho, M. & Ferreira, I.C.F.R. (2013). A review on antioxidants, prooxidants and related controversy: natural and synthetic compounds, screening and analysis methodologies and future perspectives. Food and Chemical Toxicology, 51: 15–25.
- Celikler, S., Yildiz, G., Vatan, O. & Bilaloglu, R. (2008). In vitro antigenotoxicity of Ulva rigida C. Agardh (Chlorophyceae) extract against induction of chromosome aberration, sister chromatid exchange and micronuclei by mutagenic agent MMC. Biomedical and Environmental Sciences, 21: 492–498.
- Celikler, S., Tas, S., Vatan, O., Ziyanok-Ayvalik, S., Yildiz, G. & Bilaloglu, R. (2009). Anti-hyperglycemic and antigenotoxic potential of Ulva rigida ethanolic extract in the experimental diabetes mellitus. Food and Chemical Toxicology, 47: 1837–1840.
- Celikler, S., Tas, S., Ziyanok-Ayvalik, S., Vatan, O., Yildiz, G. & Ozel, M. (2014). Protective and antigenotoxic effect of Ulva rigida C. Agardh in experimental hypothyroid. Acta Biologica Hungarica, 65: 13–26.
- Chakraborty, K. & Paulraj, R. (2010). Sesquiterpenoids with free-radical-scavenging properties from marine macroalga Ulva fasciata Delile. Food Chemistry, 122: 31–41.
- Ciccia, A. & Elledge, S.J. (2010). The DNA damage response: making it safe to play with knives. Molecular Cell, 40: 179–204. doi:10.1016/j.molcel.2010.09.019.
- Collins, A.R. (2004). The comet assay for DNA damage and repair: principles, applications, and limitations. Molecular Biotechnology, 26: 249–261.
- de Roos, B. & Duthie, G.G. (2015). Role of dietary pro-oxidants in the maintenance of health and resilience to oxidative stress. Molecular Nutrition and Food Research, 59: 1229–1248.
- DFG (2000). Carcinogenic and Anticarcinogenic Factors in Food. Senate Commission on Food Safety. Wiley‐VCH Verlag GmbH. doi: 10.1002/3527606246.
- El-Agamey, A., Lowe, G.M., McGarvey, D.J., Mortensen, A., Phillip, D.M., Truscott, T.G. & Young, A.J. (2004). Carotenoid radical chemistry and antioxidant/pro-oxidant properties. Archives of Biochemistry and Biophysics, 430: 37–48.
- European Advisory Services (EAS) (2008). Marketing Food Supplements, Fortified and Functional Foods in Europe: Legislation and Practice. ISBN: 9789080699533.
- Faustino, M.V., Seca, A.M.L., Silveira, P., Silva, A.M.S. & Pinto, D.C.G.A. (2017). Gas chromatography–mass spectrometry profile of four Calendula L. taxa: a comparative analysis. Industrial Crops and Products, 104: 91–98.
- Fernández-Bedmar, Z., Anter, J., de La Cruz-Ares, S., Muñoz-Serrano, A., Alonso-Moraga, Á. & Pérez-Guisado, J. (2011). Role of citrus juices and distinctive components in the modulation of degenerative processes: genotoxicity, antigenotoxicity, cytotoxicity, and longevity in Drosophila. Journal of Toxicology and Environmental Health, Part A, 74: 1052–1066.
- Forester, S.C. & Lambert, J.D. (2011). The role of antioxidant versus pro-oxidant effects of green tea polyphenols in cancer prevention. Molecular Nutrition and Food Research, 55: 844–854.
- Füzfai, Z., Boldizsár, I. & Molnár-Perl, I. (2008). Characteristic fragmentation patterns of the trimethylsilyl and trimethylsilyl–oxime derivatives of various saccharides as obtained by gas chromatography coupled to ion-trap mass spectrometry. Journal of Chromatography A, 1177: 183–189.
- Gaivão, I. & Comendador, M.A. (1996). The w/w+ somatic mutation and recombination test (SMART) of Drosophila melanogaster for detecting reactive oxygen species: characterization of 6 strains. Mutation Research, 360: 145–151.
- Gaivão, I., Sierra, L.M. & Comendador, M.A. (1999). The w/w+ SMART assay of Drosophila melanogaster detects the genotoxic effects of reactive oxygen species inducing compounds. Mutation Research, 440: 139–145.
- Ghantous, A., Gali-Muhtasib, H., Vuorela, H., Saliba, N.A. & Darwiche, N. (2010). What made sesquiterpene lactones reach cancer clinical trials? Drug Discovery Today, 15: 668–678.
- Golm Metabolome Database (GMD) (2019). http://gmd.mpimp-golm.mpg.de/( accessed 3. 11.19).
- Gómez-Pinchetti, J.L., Fernández, E.C., Díez, P.M. & García-Reina, G. (1998). Nitrogen availability influences the biochemical composition and photosynthesis of tank-cultivated Ulva rigida (Chlorophyta). Journal of Applied Phycology, 10: 383.
- Guilherme, S., Gaivão, I., Santos, M.A. & Pacheco, M. (2012). DNA damage in fish (Anguilla anguilla) exposed to a glyphosate-based herbicide – elucidation of organ-specificity and the role of oxidative stress. Mutation Research, 743: 1–9.
- Guiry, M.D. & Guiry, G.M. (2019). AlgaeBase. National University of Ireland, Galway. http://www.algaebase.org
- Guo, T., Lin, Q., Li, X., Nie, Y., Wang, L., Shi, L., Xu, W., Hu, T., Guo, T. & Luo, F. (2017). Octacosanol attenuates inflammation in both RAW264.7 macrophages and a mouse model of colitis. Journal of Agricultural and Food Chemistry, 65: 3647–3658.
- Halliwell, B. (2008). Are polyphenols antioxidants or pro-oxidants? What do we learn from cell culture and in vivo studies? Archives of Biochemistry and Biophysics, 476: 107–112.
- Ho, C.K., Siu-Wai, C., Siu, P.M. & Benzie, I.F. (2013). Genoprotection and genotoxicity of green tea (Camellia sinensis): are they two sides of the same redox coin? Redox Report, 18: 150–154.
- Hoek, C., Mann, D. & Jahns, H.M. (1995). Algae: An Introduction to Phycology. Cambridge University Press, Cambridge.
- Holdt, S.L. & Kraan, S. (2011). Bioactive compounds in seaweed: functional food applications and legislation. Journal of Applied Phycology, 23: 543–597.
- Hrabovski, N., Sinadinović-Fišer, S., Nikolovski, B., Sovilj, M. & Borota, O. (2012). Phytosterols in pumpkin seed oil extracted by organic solvents and supercritical CO2. European Journal of Lipid Science and Technology, 114: 1204–1211.
- Isca, V.M.S., Seca, A.M.L., Pinto, D.C.G.A., Silva, H. & Silva, A.M.S. (2014). Lipophilic profile of the edible halophyte Salicornia ramosissima. Food Chemistry, 165: 330–336.
- Johnson, I.T. & Fenwick, G.R. (2000). Dietary Anticarcinogens and Antimutagens: Chemical and Biological Aspects. The Royal Society of Chemistry, Cambridge.
- Knasmüller, S., Steinkellner, H., Majer, B.J., Nobis, E.C., Scharf, G. & Kassie, F. (2002). Search for dietary antimutagens and anticarcinogens: methodological aspects and extrapolation problems. Food and Chemical Toxicology, 40: 1051–1062.
- Lahaye, M., Gómez‐Pinchetti, J., del Rio, M.J. & Garcia‐Reina, G. (1995). Natural decoloration, composition and increase in dietary fibre content of an edible marine algae, Ulva rigida (Chlorophyta), grown under different nitrogen conditions. Journal of the Science of Food and Agriculture, 68: 99–104.
- Lambert, J.D. & Elias, R.J. (2010). The antioxidant and pro-oxidant activities of green tea polyphenols: a role in cancer prevention. Archives of Biochemistry and Biophysics, 501: 65–72.
- Leandro, L.F., Munari, C.C., Sato, V.L.F.L., Alves, J.M., de Oliveira, P.F., Mastrocola, D.F.P., Martins, S. de P.L., Moraes, T. da S., de Oliveira, A.I., Tozatti, M.G., Cunha, W.R. & Tavares, D.C. (2013). Assessment of the genotoxicity and antigenotoxicity of (+)-usnic acid in V79 cells and Swiss mice by the micronucleus and comet assays. Mutation Research, 753: 101–106.
- Lloyd, D.R. & Phillips, D.H. (1999). Oxidative DNA damage mediated by copper(II), iron(II) and nickel(II) Fenton reactions: evidence for site-specific mechanisms in the formation of double-strand breaks, 8-hydroxydeoxyguanosine and putative intrastrand cross-links. Mutation Research, 424: 23–36.
- Marinho-Soriano, E., Fonseca, P.C., Carneiro, M.A.A. & Moreira, W.S.C. (2006). Seasonal variation in the chemical composition of two tropical seaweeds. Bioresources Technology, 97: 2402–2406.
- Marques, A., Ferreira, J., Abreu, H., Pereira, R., Rego, A., Serôdio, J., Christa, G., Gaivão, I. & Pacheco, M. (2018). Searching for antigenotoxic properties of marine macroalgae dietary supplementation against endogenous and exogenous challenges. Journal of Toxicology and Environmental Health, Part A, 81: 1–18.
- Martins, I., Oliveira, J.M., Flindt, M.R. & Marques, J.C. (1999). The effect of salinity on the growth rate of the macroalgae Enteromorpha intestinalis (Chlorophyta) in the Mondego estuary (west Portugal). Acta Oecologica, 20: 259–265.
- McAvoy, K.M. & Klug, J.L. (2005). Positive and negative effects of riverine input on the estuarine green alga Ulva intestinalis (syn. Enteromorpha intestinalis) (Linneaus). Hydrobiologia, 545: 1–9.
- Merfort, I. (2011). Perspectives on sesquiterpene lactones in inflammation and cancer. Current Drug Targets, 12: 1560–1573.
- Mezghani, S., Csupor, D., Bourguiba, I., Hohmann, J., Amri, M. & Bouaziz, M. (2016). Characterization of phenolic compounds of Ulva rigida (Chlorophycae) and its antioxidant activity. European Journal of Medicinal Plants, 12: 1–9.
- Mohamed, S., Hashim, S.N. & Rahman, H.A. (2012). Seaweeds: a sustainable functional food for complementary and alternative therapy. Trends in Food Science and Technology, 23: 83–96.
- Ohta, Y., Ohashi, K., Matsura, T., Tokunaga, K., Kitagawa, A. & Yamada, K. (2008). Octacosanol attenuates disrupted hepatic reactive oxygen species metabolism associated with acute liver injury progression in rats intoxicated with carbon tetrachloride. Journal of Clinical Biochemistry and Nutrition, 42: 118–125.
- Oyeyemi, I.T., Yekeen, O.M., Odusina, P.O., Ologun, T.M., Ogbaide, O.M., Olaleye, O.I. & Bakare, A.A. (2015). Genotoxicity and antigenotoxicity study of aqueous and hydro-methanol extracts of Spondias mombin L., Nymphaea lotus L. and Luffa cylindrical L. using animal bioassays. Interdisciplinary Toxicology, 8: 184–192.
- Pangestuti, R. & Kim, S.-K. (2011). Biological activities and health benefit effects of natural pigments derived from marine algae. Journal of Functional Foods, 3: 255–266.
- Paniagua-Pérez, R., Madrigal-Bujaidar, E., Reyes-Cadena, S., Álvarez-González, I., Sánchez-Chapul, L., Pérez-Gallaga, J., Hernández, N., Flores-Mondragón, G. & Velasco, O. (2008). Cell protection induced by beta-sitosterol: inhibition of genotoxic damage, stimulation of lymphocyte production, and determination of its antioxidant capacity. Archives of Toxicology, 82: 615.
- Petersson, G. (1969). Mass spectrometry of alditols as trimethylsilyl derivatives. Tetrahedron, 25: 4437–4443.
- Pisani, P., Bray, F. & Parkin, D.M. (2002). Estimates of the world‐wide prevalence of cancer for 25 sites in the adult population. International Journal of Cancer, 97: 72–81.
- Podmore, I.D., Griffiths, H.R., Herbert, K.E., Mistry, N., Mistry, P. & Lunec, J. (1998). Vitamin C exhibits pro-oxidant properties. Nature, 392: 559.
- Procházková, D., Boušová, I. & Wilhelmová, N. (2011). Antioxidant and prooxidant properties of flavonoids. Fitoterapia, 82: 513–523.
- Rahmouni, N., Pinto, D.C.G.A., Santos, S.A.O., Beghidja, N. & Silva, A.M.S. (2018). Lipophilic composition of Scabiosa stellata L.: an underexploited plant from Batna (Algeria). Chemical Papers, 72: 753–762.
- Razboršek, M.I., Vončina, D.B., Doleček, V. & Vončina, E. (2008). Determination of oleanolic, betulinic and ursolic acid in Lamiaceae and mass spectral fragmentation of their trimethylsilylated derivatives. Chromatographia, 67: 433–440.
- Reis, P.A., Cassiano, J., Veiga, P., Rubal, M. & Sousa-Pinto, I. (2014). Fucus spiralis as monitoring tool of metal contamination in the northwest coast of Portugal under the European Water Framework Directives. Environmental Monitoring and Assessment, 186: 5447–5460.
- Rezende, V.B., Lins, A.B., Rezende, A., Spanó, M.A. & Sousa, N.C. (2013). Avaliação do efeito modulador da levedura de cerveja (Saccharomyces cerevisiae M.) sobre a genotoxicidade induzida pela doxorrubicina em Drosophila melanogaster. Revista de Biologia Neotropical, 10: 9–17.
- Rodeiro, I., Olguín, S., Santes, R., Herrera, J.A., Pérez, C.L., Mangas, R., Hernández, Y., Fernández, G., Hernández, I. & Hernández-Ojeda, S. (2015). Gas chromatography-mass spectrometry analysis of Ulva fasciata (green seaweed) extract and evaluation of its cytoprotective and antigenotoxic effects. Evidence-Based Complementary and Alternative Medicine. doi: 10.1155/2015/520598.
- Rosenblat, M., Volkova, N. & Aviram, M. (2013). Pomegranate phytosterol (β-sitosterol) and polyphenolic antioxidant (punicalagin) addition to statin, significantly protected against macrophage foam cells formation. Atherosclerosis, 226: 110–117.
- Ruiz, R.B. & Hernández, P.S. (2014). Diet and cancer: risk factors and epidemiological evidence. Maturitas, 77: 202–208.
- Sathivel, A., Balavinayagamani, Hanumantha Rao, B.R. & Devaki, T. (2014). Sulfated polysaccharide isolated from Ulva lactuca attenuates d-galactosamine induced DNA fragmentation and necrosis during liver damage in rats. Pharmaceutical Biology, 52: 498–505.
- Shahzad, N., Khan, W., MD, S., Ali, A., Saluja, S.S., Sharma, S., Al-Allaf, F.A., Abduljaleel, Z., Ibrahim, I.A.A., Abdel-Wahab, A.F., Afify, M.A. & Al-Ghamdi, S.S. (2017). Phytosterols as a natural anticancer agent: current status and future perspective. Biomedicine and Pharmacotherapy, 88: 786–794.
- Sharmila, R. & Sindhu, G. (2017). Evaluate the antigenotoxicity and anticancer role of β-sitosterol by determining oxidative DNA damage and the expression of phosphorylated mitogen-activated protein kinases, C-fos, C-jun, and endothelial growth factor receptor. Pharmacognosy Magazine, 13: 95–101.
- Shimada, S., Yokoyama, N. & Masuda, M. (2007). Ulva (Ulvophyceae, Chlorophyta) in Hokkaido, Japan. Journal of Japanese Botany, 82: 205–216.
- Sierra, L.M., Carmona, E.R., Aguado, L. & Marcos, R. (2014). The comet assay in Drosophila: neuroblast and hemocyte cells. In Genotoxicity and DNA Repair: A Practical Approach (Sierra, L.M. & Gaivão, I., editors), 483. Humana Press, Totowa, NJ.
- Sousa, N.C., de Rezende, A.A.A., da Silva, R.M.G., Guterres, Z.R., Graf, U., Kerr, W.E. & Spanó, M.A. (2009). Modulatory effects of Tabebuia impetiginosa (Lamiales, Bignoniaceae) on doxorubicin-induced somatic mutation and recombination in Drosophila melanogaster. Genetics and Molecular Biology, 32: 382–388.
- Suttiarporn, P., Chumpolsri, W., Mahatheeranont, S., Luangkamin, S., Teepsawang, S. & Leardkamolkarn, V. (2015). Structures of phytosterols and triterpenoids with potential anti-cancer activity in bran of black non-glutinous rice. Nutrients, 7: 1672–1687.
- Tabarsa, M., Rezaei, M., Ramezanpour, Z. & Waaland, J.R. (2012). Chemical compositions of the marine algae Gracilaria salicornia (Rhodophyta) and Ulva lactuca (Chlorophyta) as a potential food source. Journal of the Science of Food and Agriculture, 92: 2500–2506.
- Taboada, C., Millán, R. & Míguez, I. (2010). Composition, nutritional aspects and effect on serum parameters of marine algae Ulva rigida. Journal of the Science of Food and Agriculture, 90: 445–449.
- Tanna, B. & Mishra, A. (2018). Metabolites unravel nutraceutical potential of edible seaweeds: an emerging source of functional food. Comprehensive Reviews in Food Science and Food Safety, 17: 1613–1624.
- Valentão, P., Trindade, P., Gomes, D., de Pinho, P.G., Mouga, T. & Andrade, P.B. (2010). Codium tomentosum and Plocamium cartilagineum: chemistry and antioxidant potential. Food Chemistry, 119: 1359–1368.
- Wang, R., Paul, V.J. & Luesch, H. (2013). Seaweed extracts and unsaturated fatty acid constituents from the green alga Ulva lactuca as activators of the cytoprotective Nrf2–ARE pathway. Free Radical Biology and Medicine, 57: 141–153.
- Wells, M.L., Potin, P., Craigie, J.S., Raven, J.A., Merchant, S.S., Helliwell, K.E., Smith, A.G., Camire, M.E. & Brawley, S.H. (2017). Algae as nutritional and functional food sources: revisiting our understanding. Journal of Applied Phycology, 29: 949–982.
- Yamori, Y., Miura, A. & Taira, K. (2001). Implications from and for food cultures for cardiovascular diseases: Japanese food, particularly Okinawan diets. Asia Pacific Journal of Clinical Nutrition, 10: 144–145.
- Yen, G.-C., Duh, P.-D. & Tsai, H.-L. (2002). Antioxidant and pro-oxidant properties of ascorbic acid and gallic acid. Food Chemistry, 79: 307–313.
- Yildiz, G., Celikler, S., Vatan, O. & Dere, S. (2012). Determination of the anti-oxidative capacity and bioactive compounds in green seaweed Ulva rigida C. Agardh. International Journal of Food Properties, 15: 1182–1189.
- Yoshida, Y. & Niki, E. (2003). Antioxidant effects of phytosterol and its components. Journal of Nutritional Science and Vitaminology, 49: 277–280.
- Yuan, Y. & Walsh, N. (2006). Antioxidant and antiproliferative activities of extracts from a variety of edible seaweeds. Food and Chemical Toxicology, 44: 1144–1150.
- Zar, J. (1996). Biostatistical Analysis. Prentice Hall, Upper Saddle River, NJ.
- Zeiger, E. & Tice, R. (1997). Saw palmetto (Serenoa repens) and one of its constituent sterols: β-sitosterol. Review of Toxicological Literature. 66 pp.
- Zhang, S., Won, Y.-K., Shen, C.-N.O. & H.-M. (2005). Anti-cancer potential of sesquiterpene lactones: bioactivity and molecular mechanisms. Current Medicinal Chemistry, 5: 239–249.
- Zubia, M., Fabre, M.S., Kerjean, V., Le Lann, K., Stiger-Pouvreau, V., Fauchon, M. & Deslandes, E. (2009). Antioxidant and antitumoural activities of some Phaeophyta from Brittany coasts. Food Chemistry, 116: 693–701.