Abstract
Membrane fusion is of fundamental importance for many biological processes and has been a topic of intensive research in past decades with several models being proposed for it. Fossils had previously not been considered relevant to studies on membrane fusion. But here two different membrane fusion patterns are reported in the same well-preserved fossil plant from the Miocene (15–20 million years old) at Clarkia, Idaho, US. Scanning electron microscope, transmission electron microscope, and traditional studies reveal the vesicles in various states (even transient semi-fusion) of membrane fusion, and thus shed new light on their membrane structure and fusion during exocytoses. The new evidence suggests that vesicles in plant cells may have not only a unit membrane but also a half-unit membrane, and that a previously overlooked membrane fusion pattern exists in plant cells. This unexpected result from an unexpected material not only marks the first evidence of on-going physiological activities in fossil plants, but also raises questions on membrane fusion in recent plants.
Introduction
Membrane fusion has been a focus of intensive biological and biophysical research in past decades (Palade & Bruns Citation1968, Poole et al. Citation1970, Lucy Citation1970, Satir et al. Citation1973, Chandler & Heuser Citation1979, Scherz et al. Citation1990, Galway et al. Citation1993, Robinson et al. Citation1996, Thiel et al. Citation1998, Thiel & Battey Citation1998, Battey et al. Citation1999, Blatt et al. Citation1999, Homann & Thiel Citation1999, Blatt Citation2002, Gruner Citation2002, Yang & Huang Citation2002, Hed & Safran Citation2003, Malinin & Lentz Citation2004, Ostrowski et al. Citation2004, Giraudo et al. Citation2005, Lu et al. Citation2005, Chernomordik & Kozlov Citation2005, Ungermann & Langosch Citation2005, Anderson Citation2006, Koumandou et al. Citation2007, Wong et al. Citation2007) because it is closely related to many fundamental biological processes and medical applications, including cell signaling, cell growth, development, homeostasis, stomatal function, secretion, cell wall formation, fertilization, product transport, drug delivery, and gene therapy (van der Woude et al. Citation1971, Chandler & Heuser Citation1979, Meindl et al. Citation1992, Galway et al. Citation1993, Thiel et al. Citation1998, Battey et al. Citation1999, Homann & Thiel Citation1999, Blatt Citation2002, Yang & Huang Citation2002, Chernomordik & Kozlov Citation2005, Wong et al. Citation2007). Biological membranes, including vesicular ones, are all thought to have a double-layered structure (Kimball Citation1970, Ambrose & Easty Citation1979, Zheng Citation1996, Staehelin & Newcomb Citation2002, Chernomordik & Kozlov Citation2005) even though half-unit membranes have been reported several times in cells (Hodge et al. Citation1956, Csillik Citation1963, Cardell et al. Citation1967, Anderson et al. Citation1970, Yatsu et al. Citation1971, Konar et al. Citation1972, Yatsu & Jacks Citation1972, Gardner & Hess Citation1977, Hilhorst et al. Citation1982, de Castro & Martinez-Honduvilla Citation1984, Scherz et al. Citation1990, Somerville et al. Citation2002, Schumann et al. Citation2003). Although vesicle trafficking is of essential importance for nearly all eukaryotic cells (Blatt et al. Citation1999, Blatt Citation2002, Koumandou et al. Citation2007) and several models and modifications have been proposed for the fusion between vesicle and cytoplasmic membranes (Thiel & Battey Citation1998, Blatt et al. Citation1999, Battey et al. Citation1999, Blatt Citation2002, Chernomordik & Kozlov Citation2005, See a), none mentions the fusion between a half-unit membrane and a cytoplasmic membrane (CM).
There are an increasing number of well-preserved plant fossils with various chemical species, cytoplasm residues and organelles preserved (Taylor & Millay Citation1977, Niklas Citation1976a,Citationb& Citation1976c, Citation1981, Niklas & Giannasi Citation1977, Niklas et al. Citation1978, Citation1982, Citation1985, Golenberg et al. Citation1990, Soltis et al. Citation1992, Kim et al. Citation2004, Schönhut et al. Citation2004, Wang Citation2004,Citation2006, Citation2007a&b, Collinson et al. Citation2005, Koller et al. Citation2005, Ozerov et al. Citation2006, Gupta et al. Citation2007a& Citation2007b, Wang & Cui Citation2007). Among them, Taylor & Millay (Citation1977) used TEM (transmission electron microscope) for the first time to study the ultrastructures in cytoplasm remains of fern spores from the Pennsylvanian even though their materials did not allow them to extract too much information. Niklas et al. (1976–1985) not only extracted phytochemical species from fossil plants ranging from the Devonian to Oligocene ages, but also studied the coalified fossil materials from the Miocene in US and demonstrated the existence of chloroplasts, biomembranes, nuclei, and possible mitochondria in these fossil plants. Schönhut et al. (Citation2004) once again found many chloroplasts in Metasequoia leaves from the Eocene in Canada and discussed their preservation mechanisms and environmental implications. Wang and his colleague (2004–2007) studied the charcoalified plant materials from the Mesozoic-Cenozoic in US and China, finding well-preserved fossil cytoplasm with ultrastructures, and proposing a new fossilizing mechanism (including lightning and wild fire). They also discussed the potential physiological value of chemical information in the cytoplasm of fossils. Gupta et al. (Citation2007a & b) studied the preservation of cuticles from Miocene in France and Oligocene in Germany, found multiple chemical species (lignin, polysaccharide, protein and chitin) in fossil materials, and also mentioned the cellular structures preserved in epidermal cells in fossil conifers. Collinson et al. (Citation2005) studied the taphonomy and preservation of fossil plants in Clarkia, Idaho, US, demonstrating clearly the existence of ultrastructures including chloroplast in the Miocene fossil plants. From the same locality, Golenberg et al. (Citation1990), Soltis et al. (Citation1992) and Kim et al. (Citation2004) have independently extracted DNA sequences from fossil plant materials three times. Koller et al. (Citation2005) studied a cypress fragment enclosed in Eocene amber from Russia with the organelles preserved intact. Ozerov et al. (Citation2006) demonstrated the presence of stainable DNA in fossil plant cells in the Eocene of Russia and attributed the preservation to the presence of tannins. However, all of these works are about the static structures in plant cells, and to our best knowledge, there is no report on the physiological processes, such as membrane fusion or exocytosis, in any fossil plants yet. This paper reports membrane fusion in plant cells 15–20 Ma (million years) old. This new evidence sheds additional light on membrane fusion and the structure of the vesicle membrane, and proposes a new membrane fusion pattern hitherto unknown.
Materials and methods
One mummified coniferous cone was studied in this research. The premature cone (PB20715) was collected from the Miocene at Clarkia, Idaho, US (P33, 47°01′N, 116°25′W, Yang et al. Citation2005) in 2005. Originally, the cone was embedded in a gray siltstone, a sediment formed in a storm-influenced lake under anoxic conditions (Yang et al. Citation2005). The sediment was composed almost completely of diatom.
The fossil was recognized and photographed using a Leica MZ-16A stereomicroscope with digital camera. Tiny pieces of the organic material were sampled for microscopic observation. The fossil material was placed sequentially in 20% HCl, 40% HF, and then 20% HCl to remove inorganic minerals. In order to remove minerals completely, this processing was repeated twice more. The sample was then observed under a Leon 1530 VP SEM (scanning electron microscope) at Nanjing Institute of Geology and Palaeontology, Nanjing, China, and EDXMA (energy dispersive X-ray microanalysis) was carried out on the samples on the same SEM. Afterwards, tiny pieces of the samples were embedded in Epon 812 for thin and ultrathin sections at Nanjing Normal University, Nanjing, China. The recipe for the 20ml resin solution was 10.28 ml Epon 812, 1.24 ml DDSA, 8.48 ml MNA, and 0.34 ml DMP-30. The samples were put in acetate for 3 h, 1 h each in 50% and 67% Epon resin solutions in acetate, then a 100% resin solution overnight. Then samples were immersed in fresh pure Epon resin in a container and cured in a progressively warmer oven set at 30°C for 24 h, 45°C for 24 h, and 60°C for 24 h. Then the cured blocks were trimmed and sectioned using a Reichert-Jung Ultracut E ultramicrotome set at an interval of 2 µm using a glass knife for light microscopy and SEM (c,d), and with a Leica Ultracut R ultramicrotome set at an interval of 70 nm using a diamond knife for TEM ( and ). The thin sections were photographed using an Olympus polarizing microscope (model BX51-75J21PO standard set). The ultrathin sections were stained with lead citrate. About 85 ultrathin sections in total on 12 grids were observed under Jeol JEM-1230 electron microscope at Nanjing Institute of Geology and Palaeontology, Nanjing, China. The thin sections were processed with saturated solution of NaOH in methanol alcohol to remove the embedding resin before further SEM examination for comparison (c). The electron micrographs were saved in TIFF format, and pieced together for publication using Photoshop 7.0.
Figure 1. A mummified coniferous cone with cytoplasm preserved from the Miocene (15–20 Ma) at Clarkia, Idaho, US. Specimen number PB20715, deposited in the Palaeobotanical Collection, Nanjing Institute of Geology and Palaeontology, Nanjing, China. (a) The fossil cone still embedded in the sediments. Note the cone axis (CA) and bract-scale complexes attached (arrows). Bar = 2 mm. (b) A cross view of a bract with the SEM. Note the intact cells and their contents (arrows) in the tissue. Bar = 20 µm. (c) A view of a bract thin section with the SEM. Note the epidermal and other cells, cytoplasm (CP) filling up the lumina, possible resin duct (RD), the cleavages between cells and their cell walls, and the ruptured cuticle (arrow). Section is about 2 µm thick. Bar = 10 µm. (d) A view of a cross-section of a bract-scale with the light microscope. Note the dark-colored cytoplasm in contrast to the light-colored cell walls in this unstained section. Bar = 0.1mm. This Figure is published with the permission of Nanjing Institute of Geology and Palaeontology, Nanjing, China and is reproduced in colour in Molecular Membrane Biology online.
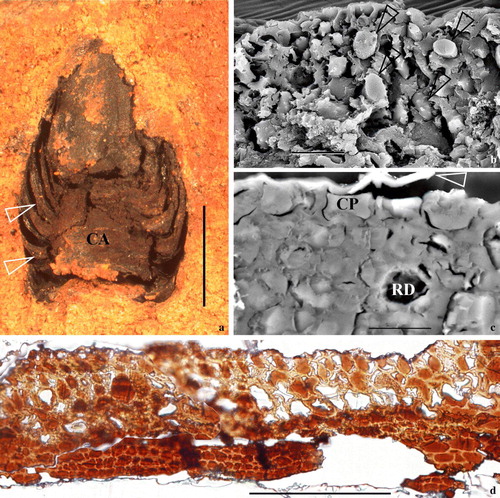
Figure 2. Vesicles and their relationships with CM in epidermal cells of a cone bract. All TEM electron micrographs. (a) A view of a cross-section of a bract. Note the faint-stained cell walls and dark-stained cytoplasm. The rectangular area is enlarged in b. Reproduced with permission from Wang (2007b). Bar = 5 µm. (b) A detailed view of an epidermal cell, enlarged from the rectangle in a. Note the dark-stained cytoplasm and faint-stained cell walls. The surface of the tissue is to the lower right. The rectangular region is shown in detail in c. Bar = 1 µm. (c) A detailed view of a portion of the cell shown in the rectangle in b. Note the relationship between the cuticle (CU), cell wall (CW), cytoplasmic membrane (CM), and cytoplasm (CP). Bar = 500 nm. (d) A detailed view of the vesicle fused to the CM, enlarged from the rectangle in c. Note that the vesicle's half-unit membrane is connected only with the inner leaflet of the CM (arrow), which becomes wavy near the fusion pore. The outer leaflet of the CM at the fusion pore is barely visible at the fusion pore. The CM is clearly two-layered. Reproduced with permission from Wang (Citation2007b). Bar = 100 nm. (e) A spherical vesicle with a half-unit membrane in the cytoplasm. Bar = 100 nm. (f) A vesicle connected with the CM through a cylindrical connection. Note the connection between vesicle membrane and the CM (black arrow), and the still-visible transverse membrane (white arrow) at the bottom of the vesicle. Bar = 100 nm. (g) The half-unit membrane of a vesicle connected with the inner leaflet of the CM. Note the connection between vesicle membrane and the inner leaflet of the CM (white arrow). Bar = 100 nm.
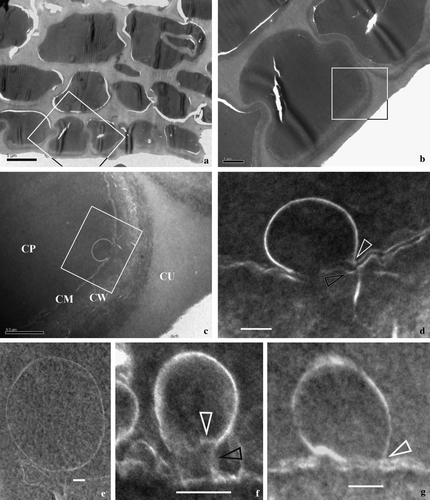
Figure 3. Vesicles’ membranes and their relationship with double-layered cytoplasmic membrane. All TEM electron micrographs. (a) A half-unit membrane of a vesicle connected to the inner leaflet of the CM. Note the connection between vesicle membrane and the inner leaflet of the CM (white arrow) in contrast to the dangling outer leaflet of CM (black arrow). Bar = 100 nm. (b) Half-unit membranes of several vesicles connected with the inner leaflet of the CM. Note the connection between vesicle membrane and the inner leaflet of the CM (white arrows) in contrast to the outer leaflet of CM (black arrow) parallel to the inner leaflet. Bar = 100 nm. (c) A half-unit membrane of another vesicle connected with the inner leaflet of the CM (white arrow). Note the dangling outer leaflet of CM (black arrow) and the drastic variation in CM thickness on each side of the vesicle. Bar = 100 nm. (d) Half-unit membranes of a vesicle connected with the CM. Note the connection between vesicle membrane and the inner leaflet of the CM (white arrows) in contrast to the dangling outer leaflet of CM (black arrow), and two more vesicles in the cell. Bar = 100 nm. (e) Several vesicles with half-unit membranes crowded along the CM. Note that the CM is highly disturbed with its double-layered structure sometimes still visible (white arrow), and that two adjacent vesicles may have their membranes closely spaced (black arrow). Bar = 100 nm. (f) A vesicle connected with the CM. The vesicle has a unit membrane (quite different from those in a–e, white arrow). The vesicle and the CM form an omega-shaped configuration with a narrow neck (black arrow). The vesicle membrane may become thicker and obscure due to oblique orientation of the membrane relative to the sectioning plane. Bar = 100 nm. (g) A typical double-layered structure of the CM. Bar = 100 nm. (h) A couple of the adjacent spherical vesicles before fusing with the CM. Bar = 200 nm. (i) Many vesicles with a half unit membrane in cytoplasm. Note the size and shape of the vesicles may vary from one to another. Bar = 200 nm. (j) More vesicles in the cytoplasm. Note the relationship between vesicles (arrows), and the varying membrane structure even within the same vesicle (lower arrow). Bar = 100 nm.
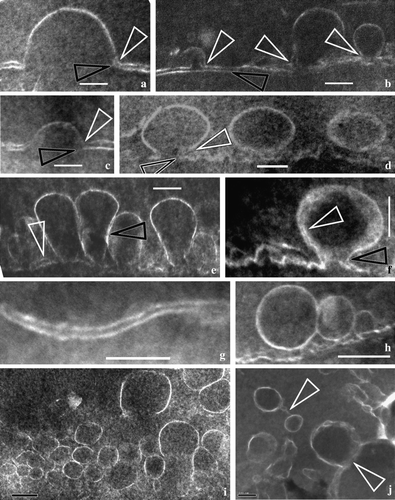
Results
The fossil studied is a three-dimensionally preserved mummified coniferous cone, about 5 mm long and 3.5 mm in diameter (a). It is composed of a central axis with bracts and scales spirally arranged on the cone axis (a). The EDXMA indicates that the elemental composition of the fossil material is mainly carbon and oxygen (C 58.77–82.15%, O 17.07–39.34%, by wt.; H is not detectable in EDXMA). The epidermal cells as well as those in the ground tissue are preserved almost intact (b–d, a–d). Cytoplasm residue is found in most cells (b–d, a–c). Under SEM, the cytoplasm appears as blocks of material separated by cell walls (b–c). A similar situation is also seen under the light microscope (d), and at this time the cells and the cell walls are distinguished by their different colors (d). Under TEM, the cytoplasm is relatively dark-stained with lead citrate, while the cell walls are faint-stained (a–c). Many vesicles are seen in the epidermal cells ( & ). A vesicle is spherical when singular in the cytoplasm and has a half-unit membrane (e, h,i, b) or a unit membrane (f, a). Vesicles may be isolated (e–g, 3d,i) or attached each other (e,h–j). There appears to be a cylindrical connection between a vesicle and the CM when the vesicle is about to fuse with the CM (f, b). The CM typically appears as two parallel light lines, corresponding to its inner and the outer leaflets (c–d,g, a–d,g, a,b), and about 12.5 nm (c) to 40 nm (f) thick, depending on their orientation and original condition (c,d,g, a–d,g). The CM may become wavy or otherwise disturbed when interrupted by one or more spherical or omega-shaped vesicles (c,d,f,g, a–f, a,b). The outer leaflet of the CM near the fusion pore either appears disturbed (c,d,f, d, b), disappears (a,c,d) or remains intact (g, b). Drastic change in CM thickness and configuration may occur in a very limited area (c). The vesicles of various shapes (spherical to omega-shaped) are defined by one (c,d,e, a–e,h-j, b) or two curved lines (f, a). The thickness of the double-layered vesicle membrane may range from 12 nm (f, white arrow) to more than 40 nm (f) in the same vesicle due to varied local orientation of membrane relative to the sectioning plane.
Discussion
Demineralizing using HF-HCl is a reliable way to remove minerals from charcoal (black carbon) that has been accepted and practiced by chemists (Poirier et al. Citation2000, Citation2002, Eusterhues et al. Citation2003). Therefore it is safe to assume that after three-time HF-HCl processings, only material of organic origin is left in this fossil material. It is apparent that the only source for the organic material in this fossil is plant tissues and their contents. This assumption is supported by the EDXMA analysis that indicates the composition of the fossil materials (C 58.77–82.15%, O 17.07–39.34%, by wt.) is similar to that of recent charcoal of Pinus stem (Baldock & Smernick Citation2002, Nguyen et al. Citation2004) and therefore confirms its plant origin. Clarkia, Idaho, US is a worldwide famous Lagerstätte for fossil plants (Yang et al. Citation2005). Niklas et al. (Citation1985) have collected their fossil materials from this locality, extracted chemical species of phytotaxonomical values, and demonstrated the existence of chloroplasts, nuclei, biomembranes and possible mitochondria in their fossils. Recently, Collinson et al. (Citation2005) collected their materials with superior ultrastructures, including those of chloroplasts and nuclei, from this locality. Furthermore, Golenberg et al. (Citation1990), Soltis et al. (Citation1992) and Kim et al. (Citation2004) have independently and successfully extracted DNA sequences from fossil plants collected at this locality for three times. All of these works point to the superior preservation of fossil plants and the potential existence of ultrastructures in fossil cytoplasm in the cone reported here. The fact that there is no shrinkage of the cells in the cone tissues suggests that the cells are preserved almost in their life condition. Considering all of this, it is not surprising to find fine structures in this fossil cone even though physiological processes such as membrane fusion are somewhat beyond expectation.
Unlike those of recent material that has to undergo artificial fixing where artifacts may be introduced, the fossil material here does not need artificial killing or fixing and therefore eliminates the possibility of artificial errors due to killing/fixing. This is not hard to conceive because if the fossil cytoplasm was not fixed before being fossilized, we could see nothing as it would have disappeared long before considering 15–20 Ma have elapsed (Yang et al. Citation2005). So although we do not know exactly what kinds of natural factors were involved in the fossilization, we are not concerned with the problems of fixing and its concomitant artifacts in this case.
The material of the fossil is of charcoal, which is very stable and inert to most chemicals. This explains why charcoal preserves well (Patterson et al. Citation1987), and also eliminates the possibility of artifacts otherwise possibly introduced during the embedding and staining. Although it is true that the fact reported here is provocative, there are ways to test its faithfulness: (i) by independent research on other fossil materials, and (ii) by comparison between different portions of this fossil material. For the first case, whatever the future studies will indicate, now we have to be patient and wait for further works. Whoever first finds this phenomenon must make it is possible for other people to be able to test the correctness of the findings. For the second case, not all cells in the tissue demonstrate exocytosis and membrane fusion. Since the whole tissue has undergone the exact same processing, the patterns of the differentiated electron density visualized under TEM, namely the TEM micrographs, should be correlated with the original heterogeneous patterns of different constituents and/or features in the fossil material. These micrographs are the grounds for interpreting the ultrastructures in this fossil plant.
Vesicles play an essential role in communication and cargo transport in plant cells (van der Woude et al. Citation1971, Takeo et al. Citation1973, Meindl et al. Citation1992, Hohl et al. Citation1996, Thiel et al. Citation1998, Battey et al. Citation1999, Homann & Thiel Citation1999, Weise et al. Citation2000, Blatt Citation2002). Exocytosis occurs when the CM and the vesicle form an omega-shaped configuration, a fusion pore is formed between them, and the vesicle contents are released into the extracellular space (Thiel & Battey Citation1998, Battey et al. Citation1999, Weise et al. Citation2000). At this time, the cells are characterized by invagination of CM (Takeo et al. Citation1973, Esau Citation1977, Chandler & Heuser Citation1979, Buvat Citation1989). In this fossil plant material, the above dynamic process cannot be seen because the fossil materials and their electron micrographs are static. However, the electron micrographs in c,d, f, g and a–f closely resemble the omega shapes of vesicles in recent plants (Figures 8–9, Takeo et al. Citation1973, Figure 11, Chandler & Heuser Citation1979, Figure 6, Chandler & Heuser Citation1981, , Heuser & Reese Citation1981, a, Rao & Dave Citation1983, and , Thiel & Battey Citation1998, , Battey et al. Citation1999, a, Marsh & McMahon Citation1999, Figure 5, Weise et al. Citation2000, , Blatt Citation2002, b,c, Higgins & McMahon Citation2002, c,f, Stefanowska et al. Citation2002, a–d, Jeremic et al. Citation2003, Figure 5c, Jena Citation2005a), thus the various shapes of many vesicles (c,d,f,g, 3a–f) altogether imply various stages of their continuous fusing with the CM. By the way, there is no information on the origin of these vesicles even though there are other biomembranes in cytoplasm probably related to these vesicles (Supplementary figure – Online version only).
Figure 4. Diagram showing two different membrane fusion patterns. (a) A vesicle with a unit membrane and its fusion with double-layered cytoplasmic membrane. Note that both the inner and the outer leaflets of the CM are connected with their counterparts in the vesicle membrane. (b) A vesicle with a half-unit membrane and its fusion with double-layered CM. Note that only the inner leaflet of the CM is connected with the vesicle membrane while the outer leaflet of the CM is slightly distorted due to the impact of the vesicle content. This is a new pattern proposed based on the observation on cells in this fossil material.

Normally, as in recent plant cells, the CM appears as two smooth parallel lines around cytoplasm (c,d,g, a–c,g, a,b). Most membrane fusions in the cone occur between a vesicle's half-unit membrane and CM's inner leaflet, while the corresponding outer leaflet of the CM either remains intact (g, b, leftmost white arrow), or appears disturbed (c,d, d). The disturbed outer leaflet suggests that it is under pressure or impact from the vesicle contents. The vesicles and their contents appear to cause great changes in CM profile and thickness (d, a–f). The CM may be as thin as 12.5 nm (c), very close to that in recent materials (9–11 nm, Morré Citation1990), while it could be up to 40 nm thick when highly disturbed (f). This variation scope appears wide at the first glance; however, a similar drastic variation has been reported for CM, ranging from 5 nm up to 18 nm, in recent cells (Korn Citation1966). Considering that the CMs in this fossil material are highly disturbed and that those in recent materials (Korn Citation1966) are just in resting status, the drastic variation in CM thickness appears less surprising. And most of the thicknesses of the CMs in this fossil fall in between the two extremities (d,g, a–e,g), depending on orientation and other factors (Korn Citation1966). The drastic variation in CM thickness from 12.5 nm to 31 nm within 300 nm (the left and right of the fused vesicle in c) implies that the increased thickness of the CM is due more to the original membrane disruption rather than an oblique sectioning and that the image (c) more or less reflects the true status of the CM even though the counterpart of such a drastic variation has not been seen in recent cells yet.
Membrane fusion is a process ubiquitous and fundamental to eukaryotic cells (Gruner Citation2002, Yang & Huang Citation2002, Jahn et al. Citation2003, Tamm et al. Citation2003, Ostrowski et al. Citation2004, Koumandou et al. Citation2007, Wong et al. Citation2007), and it is closely related to the structures of the membranes involved in. Although vesicle trafficking has been intensively studied, little is known about the membrane of secretory vesicles (Battey et al. Citation1999). On one hand, it is believed that vesicle membranes have a structure similar to that of the CM (Buvat Citation1989), and it is generalized that all biological membranes are unit membranes with a double-layered structures (Holum Citation1978, Staehelin & Newcomb Citation2002). Thus it is not surprising that almost all of the models of membrane fusion concern fusion between two unit membranes (Palade & Bruns Citation1968, Lucy Citation1970, Poole et al. Citation1970, Thiel & Battey Citation1998, Blatt et al. Citation1999, Blatt Citation2002, Tamm et al. Citation2003, Tuma & Hubbard Citation2003, Jena Citation2005a&Citationb, Chernomordik & Kozlov Citation2005, Wong et al. Citation2007, a). On the other hand, although half-unit membranes, composed of a monomolecular layer of radially-oriented phospholipid elements (Hodge et al. Citation1956, Csillik Citation1963, Cardell et al. Citation1967, Ambrose & Easty Citation1979, Hilhorst et al. Citation1982, Scherz et al. Citation1990), have been intensively studied by biochemists (Fromherz & Rüppel Citation1985, Moscho et al. Citation1996, Oliveira et al. Citation1998, Citation2005, Brockman Citation1999, Deleu et al. Citation2005, Rosetti et al. Citation2005), in living cells they only rarely are reported (for example, mouse liver and muscle, mature cells and young apical cells of Nitella cristata, root tips of germinating wheat, and leaves of Zea mays, Hodge et al. Citation1956; Rana esculenta and Mus rattus, Csillik Citation1963; rat, Cardell et al. Citation1967; Triticum aestivum, Anderson et al Citation1970; Allium cepa, Brassica capitata, Gossypium hirsutum, Yatsu et al. Citation1971; Ranunculus sceleratus, Konar et al. Citation1972; Arachis hypogaea, Yatsu & Jacks Citation1972; Tilletia caries, Gardner & Hess Citation1977; Desulfovibrio vulgaris, Hilhorst et al. Citation1982; Pinus pinea, de Castro & Martinez-Honduvilla Citation1984; Rhodospirillum rubrum, Scherz et al. Citation1990; Zea mays, Somerville et al. Citation2002; Arabidopsis, Schumann et al. Citation2003). It is believed that a half-unit membrane provides a good package for storage and transport of photoproducts, enzymes (Hodge et al. Citation1956, Csillik Citation1963, Cardell et al. Citation1967, Yatsu et al. Citation1971, Yatsu & Jacks Citation1972, Hilhorst et al. Citation1982, Scherz et al. Citation1990, Somerville et al. Citation2002), and hydrophobic drugs (Savić et al. Citation2006). Among them, Anderson et al. (Citation1970, c) and Yatsu et al. (Citation1971, Figure 6a) demonstrate clearly a half-unit membrane in contrast to a unit membrane in the same pictures. However, despite these reports of half-unit membranes, they have been ignored in membrane fusion research and none of the membrane fusion models mention fusion with a half-unit membrane.
In this research, transcytoses (probably all exocytoses) observed in the fossil cone consistently demonstrate fusions between a half-unit membrane of a vesicle and a unit membrane of CM (except in f). Many of the vesicles in the epidermal cells of the cone have a single-lined profile and fuse only with the inner leaflet of the CM (c,g, a–e, b). This strongly suggests the existence of half-unit membranes and a membrane fusion pattern ignored hitherto in plant cells. An unfavored interpretation for this phenomenon is that the single-lined profile of the vesicle actually stands for a unit membrane, thus there is no hassle of the so-called half-unit membrane. However, it follows that each cell has two cytoplasmic membranes around since two parallel lines are seen around the cytoplasm (c,g, a–e) and that the vesicle in f also has two unit-membranes around. Apparently, these deductions, especially the first one, are hard to accept for most botanists. However, if people adopted this idea, a plastid, nucleus or mitochondrion (the only organelles with two unit membranes around) transferred across the CM would be a phenomenon of great scientific importance. Due to the difficulty to accept two cytoplasmic membranes around a single cells and the non-existence of a plastid/nucleus/mitochondrion transferred across the CM, this alternative is discarded hereafter. On the contrary, the half-unit membrane interpretation is in good agreement with experimental results, in which vesicles can be formed on a lipid monolayer (Higgins & McMahon Citation2002). The CM's biochemical properties and the way it fuses with a vesicle membrane (c,d,g, a–e) suggest that the hydrophilic ends of the phospholipid elements on the vesicle membrane point to the exterior of the vesicle, and the hydrophobic ends to the interior. This type of membrane appears convenient for membrane fusion, vesicle membrane recycling, transport of hydrophobic product, and also is ideal for the kiss-and-run type of exocytosis in plant cells since there is no need to interact with the outer leaflet of the CM, in which the process is otherwise envisaged as a difficulty by modern biologists (Higgins & McMahon Citation2002). Interestingly, in the same plant (even possibly in the same cell) there are fusions between two unit membranes (f) as well as between a half-unit membrane and a unit membrane, and both types of membranes are distinct under the TEM (c,d,f,g, a–f). This implies that two membrane fusion patterns truthfully exist simultaneously even though one of them has been overlooked or unsubstantiated for a long time, and that the contents in these two kinds of vesicles should be of different biochemical properties.
A unit membrane of the vesicle appears as a light-colored curved belt of more or less uniform width (f), and its double-layered structure may still be visible sometimes (f white arrow). The thinner portion of the double-layered vesicle membrane is only 12 nm thick (f, arrow), while the thicker one in f is up to 40 nm thick (probably due to the local oblique orientation). This drastic change is understandable since the local orientation of the vesicle membrane, which is close to spherical in form, relative to the sectioning plane may vary from point to point.
Interestingly, the good preservation of this fossil plant allows the transient membrane-fusion intermediate structure (Chernomordik & Kozlov Citation2005, Wong et al. Citation2007) to be observed herein (f). It appears that there is a cylindrical connection established between the half-unit membrane of the vesicle and the CM before they fuse (f, black arrow) while the vesicle has not completely lost its spherical form (f, white arrow). This is in good agreement with models of membrane fusion (Blatt et al. Citation1999, Blatt Citation2002, Gruner Citation2002, Yang & Huang Citation2002, Jahn et al. Citation2003, Tamm et al. Citation2003, Tuma & Hubbard Citation2003, Jena Citation2005a&Citationb, Chernomordik & Kozlov Citation2005, Wong et al. Citation2007) even though the actual structure has never before been directly observed in recent cells under TEM.
As more advanced technologies become available for application recently, more and more subcellular secrets of fossil plants are revealed. Schönhut et al. (Citation2004) have revealed the well-preserved chloroplasts in cells of Eocene Metasequoia leaves from Canada, and Ozerov et al. (Citation2006) have observed stainable DNA in cells of Eocene plants from Russia. Both of the groups agree with Niklas et al. (Citation1978, Citation1985) on the preservation mechanism: tannins preserve the subcellular structures. Koller et al. (Citation2005) add another alternative for preservation by pointing out the preservation potential by amber for cellular details in an Eocene plant from Russia. It is important to note that the ultrastructures observed in Schönhut et al. and Koller et al.'s materials are especially similar to those in this paper, in terms of membrane structures and negative-staining-like appearance. In addition, Wang & Cui (Citation2007) and Wang (Citation2004, Citation2006, Citation2007a) & b) have proposed that some natural phenomena such as lightning, wild fire and high temperature may contribute to the exceptional preservation of ultrastructures in fossil cytoplasm. In spite of so many alternatives for preservation of cellular details, it remains true that little is known of the preservation mechanism of the fine ultrastructures in this fossil. However, this only means that people do not understand it much, and does not mean that the preservation mechanism does not exist. It does not mean that the unknown mechanism does not work, either. Similar situation exists in biological study on recent material. Microwave fixation is a good way to fix biological samples but its mechanism is not completely understood (Login & Dvorak Citation1994). However, this not-understanding does not stop people from using it in biological and medical research. Actually, all ‘correct’ artificial fixing and processing must have more or less changed the original status in plant cells. It is also right to assume that changes have occurred in fossil cytoplasm. Since the quality of the images in this fossil material are comparable to their recent counterparts and those in fossil plants (Niklas et al. Citation1978, Schönhut et al. Citation2004, Koller et al. Citation2005), it is not a bad idea to accept their existence for the time being and then try to figure out the mechanism later. The information in this paper implies that that there might be unknown bypasses for biological sample preparation. This challenges scientists working on recent materials and provides an initiative for the further understanding of nature, which is the only and reliable source of our knowledge.
The presence of an unknown membrane fusion pattern in this fossil plant has two possible mutually exclusive implications. (i) It is a pattern only seen in fossil plants: therefore its presence in this Miocene plant and absence in recent plants suggest that plants have dropped this pattern during evolution. If so, this would be the first time that we have understood plant evolution at this physiological level. (ii) This membrane fusion pattern also exists in recent plants but people simply have not observed it in recent plants yet. We favor the latter alternative because (i) the age of the fossil is 15–20 Ma, which appears too short for such a drastic variation to occur; and (ii) there have been, though rare, reports of half-unit membranes in recent plants (Hodge et al. Citation1956, Csillik Citation1963, Cardell et al. Citation1967, Anderson et al Citation1970, Yatsu et al. Citation1971, Konar et al. Citation1972, Yatsu & Jacks Citation1972, Gardner & Hess Citation1977, Hilhorst et al. Citation1982, de Castro & Martinez-Honduvilla Citation1984, Scherz et al. Citation1990, Somerville et al. Citation2002, Schumann et al. Citation2003) and some of them contain secretory contents, and thus they (half-unit membranes) have to fuse with CM (unit membranes) somehow somewhere sooner or later. Theoretically, this type of membrane fusion is inevitable for plant cells. It appears not impossible for scientists to find this type of membrane fusion in recent plants some time later.
Acknowledgements
We thank Dr Zhiyan Zhou for his support during this research, Drs Jinxing Lin, Chuanming Zhou, Yongdong Wang, Yi Wang, Yuandong Zhang, Ms Chunzhao Wang, Mr Yongqiang Mao, Mr Erjun Zhuo, Ms. Cuiling He, Ms Fangming Xu, Drs William Schopf, Margaret Collinson, Gaetan Guignard and Johanna Van Konijnenburg-Van Cittert for their help during this research, Dr Douglas McKinnon and Ms Margaret Joyner for help on English, two anonymous reviewers for help with the manuscript. This research is supported by Jiangsu Planned Project for Postdoctoral Research Funds, China Postdoctoral Science Foundation (No.2005037746), K. C. Wong Post-doctoral Fellowships, State Key Laboratory of Palaeobiology and Stratigraphy (Nanjing Institute of Geology and Palaeontology, CAS), National Natural Science Foundation of China Programs (No. 40632010 and No.J0630967). This paper is a contribution to IGCP 506.
References
- Ambrose, EJ, Easty, DM. 1979. Cell biology. 2nd ed. Middlesex: Thomas Nelson and Sons Ltd. p 562.
- Anderson JD, Baker JE, Worthington EK. Ultrastructural changes of embryos in wheat infected with storage fungi. Plant Physiol 1970; 46: 857–859
- Anderson LL. Discovery of the ‘porosome’; the universal secretory machinery in cells. J Cell Mol Med 2006; 10: 126–131
- Baldock JA, Smernik RJ. Chemical composition and bioavailability of thermally altered Pinus resinosa (Red pine) wood. Org Geochem 2002; 33: 1093–1109
- Battey NH, James NC, Greenland AJ, Brownlee C. Exocytosis and endocytosis. Plant Cell 1999; 11: 643–660
- Blatt MR. Toward understanding vesicle traffic and the guard cell model. New Phytol 2002; 153: 405–413
- Blatt MR, Leyman B, Geelen D. Molecular events of vesicle trafficking and control by SNARE proteins in plants. New Phytol 1999; 144: 389–418
- Brockman H. Lipid monolayers: why use half a membrane to characterize protein-membrane interactions?. Curr Opin Struct Biol 1999; 9: 438–443
- Buvat R. Ontogeny, cell differentiation, and structure of vascular plants. Springer-Verlag, Berlin 1989; 581
- Cardell RRJ, Badenhausen S, Porter KR. Intestinal triglyceride absorption in the rat: electron microscopical study. J Cell Biol 1967; 34: 123–155
- De Castro MFG, Martinez-Honduvilla CJ. Ultrastructural changes in naturally aged Pinus pinea seeds. Physiol Plant 1984; 62: 581–588
- Chandler DE, Heuser J. Membrane fusion during secretion: cortical granule exocytosis in sex urchin eggs as studied by quick-freezing and freeze-fracture. J Cell Biol 1979; 83: 91–108
- Chandler DE, Heuser JE. Arrest of membrane fusion events in mast cells by quick- freezing. J Cell Biol 1981; 86: 666–674
- Chernomordik LV, Kozlov MM. Membrane hemifusion: crossing a chasm in two leaps. Cell 2005; 123: 375–382
- Collinson, ME, Rember, W, Finch, P, Brain, APR, Gupta, NS, Pancost, RD. 2005. Morphological, anatomaical, ultrstructural and macromolecular preservation of leaves from the Miocene of Clarkia, Idaho, USA [abstract]. 15th Goldschmidt Conference, 20–25 May 2005; Moscow, Idaho. Cambridge: Cambridge Publications. p A341.
- Csillik B. Submicroscopic organization of the postsynaptic membrane in the myoneural junction: a polarization optical study. J Cell Biol 1963; 17: 571–586
- Deleu M, Paquot M, Nylander T. Fengycin interaction with lipid monolayers at the air-aqueous interface-implications for the effect of Fengycin on biological membranes. J Coll Interface Sci 2005; 283: 358–365
- Esau K. Anatomy of seed plants2nd edn. John Wiley & Sons, New York 1977; 550
- Eusterhues K, Rumpel C, Kleber M, Koegel-Knabner I. Stabilisation of soil organic matter by interactions with minerals as revealed by mineral dissolution and oxidative degradation. Org Geochem 2003; 34: 1591–1600
- Fromherz P, Rüppel D. Lipid vesicle formation: the transition from open disks to closed shells. FEBS Lett 1985; 179: 155–159
- Galway ME, Rennie PJ, Fowke LC. Ultrastructure of the endocytotic pathway in glutaraldehyde-fixed and high-pressure frozen/freeze-substituted protoplasts of white spruce (Picea glauca). J Cell Sci 1993; 106: 847–858
- Gardner JS, Hess WM. Ultrastructure of lipid bodies in Tilletia caries teliospores. J Bacteriol 1977; 131: 662–671
- Giraudo CG, Hu C, You D, Slovic AM, Mosharov EV, Sulzer D, Melia TJ, Rothman JE. SNAREs can promote complete fusion and hemifusion as alternative outcomes. J Cell Biol 2005; 170: 249–260
- Golenberg EM, Giannasi DE, Clegg MT, Smiley CJ, Durbin M, Henderson D, Zurawski G. Chloroplast DNA sequence from a Miocene Magnolia species. Nature 1990; 344: 656–658
- Gruner SM. Membrane fusion: caught in the act. Science 2002; 297: 1817–1818
- Gupta NS, Briggs DEG, Collinson ME, Evershed RP, Michels R, Jack KS, Pancost RD. Evidence for the in situ polymerisation of labile aliphatic organic compounds during the preservation of fossil leaves: implications for organic matter preservation. Org Geochem 2007a; 38: 499–522
- Gupta NS, Briggs DEG, Collinson ME, Evershed RP, Michels R, Pancost RD. Molecular preservation of plant and insect cuticles from the Oligocene Enspel Formation, Germany: evidence against derivation of aliphatic polymer from sediment. Org Geochem 2007b; 38: 404–418
- Hed G, Safran SA. Initiation and dynamics of hemifusion in lipid bilayers. Biophys J 2003; 85: 381–389
- Heuser JE, Reese TS. Structure changes after transmitter release at the frog neuromuscular junction. J Cell Biol 1981; 88: 564–580
- Higgins MK, McMahon HT. Snap-shots of clathrin-mediated endocytosis. Trends Biochem Sci 2002; 27: 257–263
- Hilhorst R, Laane C, Veeger C. Photosensitized production of hydrogen by hydrogenase in reversed micelles. Proc Natl Acad Sci USA 1982; 79: 3927–3930
- Hodge AJ, Branster M, Martin EM, Morton RK, McLean JD, Mercer FV. Effects of the physical environment on some lipoprotein layer systems and observations on their morphogenesis. J Cell Biol 1956; 2: 221–228
- Hohl I, Robinson DG, Chrispeels MJ, Hinz G. Transport of storage proteins to the vacuole is mediated by vesicles without a clathrin coat. J Cell Sci 1996; 109: 2539–2550
- Holum JR. Organic and biological chemistry. John Wiley & Sons, New York 1978; 494
- Homann U, Thiel G. Unitary exocytotic and endocytotic events in guard-cell protoplasts during osmotically driven volume changes. FEBS Lett 1999; 360: 495–499
- Jahn R, Lang T, Südhof TC. Membrane fusion. Cell 2003; 112: 519–533
- Jena BP. Cell secretion and membrane fusion. Dom Animal Endocrin 2005a; 29: 145–165
- Jena BP. Molecular machinery and mechanism of cell secretion. Exp Biol Med 2005b; 230: 307–319
- Jeremic A, Kelly M, Cho S-J, Stromer MH, Jena BP. Reconstituted fusion pore. Biophys J 2003; 85: 2035–2043
- Kim S, Soltis DE, Soltis PS, Suh Y. DNA sequences from Miocene fossils: an ndhF sequence of Magnolia latahensis (Magnoliaceae) and an rbcL sequence of Persea pseudocarolinesis (Lauraceae). Am J Bot 2004; 91: 615–620
- Kimball JW. Cell biology2nd edn. Addison-Wesley Publishing Company, Reading 1970; 415
- Koller B, Schmitt JM, Tischendorf G. Cellular fine structures and histochemical reactions in the tissue of a cypress twig preserved in Baltic amber. Proc Roy Soc B 2005; 272: 121–126
- Konar RN, Thomas E, Street HE. Origin and structure of embryoids arising from epidermal cells of the stem of Ranunculus sceleratus L. J Cell Biol 1972; 11: 77–93
- Korn ED. Structure of biological membranes. Science 1966; 153: 1491–1498
- Koumandou VL, Dacks JB, Coulson RMR, Field MC. Control systems for membrane fusion in the ancestral eukaryote. BMC Evol Biol 2007; 7: 29
- Login GR, Dvorak AM. Methods of microwave fixation for microscopy. Gustav Fischer Verlag, Stuttgart 1994; 127
- Lu X, Zhang F, McNew JA, Shin Y-K. Membrane fusion induced by neuronal SNAREs transits through hemifusion. J Biol Chem 2005; 280: 30538–30541
- Lucy JA. The fusion of biological membranes. Nature 1970; 227: 815–817
- Malinin VS, Lentz BR. Energetics of vesicle fusion intermediates: comparison of calculations with observed effects of osmotic and curvature stresses. Biophys J 2004; 86: 2951–2964
- Marsh M, McMahon HT. The structural era of endocytosis. Science 1999; 285: 215–220
- Meindl U, Lancelle S, Helper PK. Vesicle production and fusion during lobe formation in Micrasterias visualized by high-pressure freeze fixation. Protoplasma 1992; 170: 104–114
- Morré DJ. Plasma membrane cytochemistry. The plant plasma membrane, structure, function and molecular biology, C Larsson, IM Moller. Springer-Verlag, Berlin 1990; 76–92
- Moscho A, Orwar O, Chiu DT, Modi BP, Zare RN. Rapid preparation of giant unilamellar vesicles. Proc Natl Acad Sci USA 1996; 93: 11443–11447
- Nguyen TH, Brown RA, Ball WP. An evaluation of thermal resistance as a measure of black carbon content in diesel soot, wood char, and sediment. Org Geochem 2004; 35: 217–234
- Niklas KJ. The chemotaxonomy of Parka decipiens from the lower Old Red Sandstone, Scotland (U.K.). Rev Palaeobot Palynol 1976a; 21: 205–217
- Niklas KJ. Morphological and chemical examination of Courvoisiella ctenomorpha gen. et sp., a siphonous alga from the upper Devonian, West Virginia. USA. Rev Palaeobot Palynol 1976b; 21: 187–203
- Niklas KJ. Chemical examinations of some non-vascular Paleozoic plants. Brittonia 1976c; 28: 113–137
- Niklas KJ. Paleophytochemistry: implications concerning plant evolution. Paleobiol 1981; 7: 1–3
- Niklas KJ, Brown RM, Santos R, Vian B. Ultrastructure and cytochemistry of Miocene angiosperm leaf tissues. Proc Natl Acad Sci USA 1978; 75: 3263–3267
- Niklas KJ, Giannasi DE. Flavonoids and other chemicals constituents of fossil Miocene Zelkova (Ulmaceae). Science 1977; 196: 877–878
- Niklas KJ, Brown RMJr., Santos R. Ultrastructural states of preservation in Clarkia angiosperm leaf tissues: Implications on modes of preservation. Late Cenozoic history of the Pacific Northwest, CJ Smiley. American Association for the Advancement of Science, Pacific Division, San Francisco 1985; 143–159
- Niklas KJ, Tiffney BH, Leopold AC. Preservation of unsaturated fatty acids in Palaeogene angiosperm fruits and seeds. Nature 1982; 296: 63–64
- Oliveira RG, Calderón RO, Maggio B. Surface behavior of myelin monolayers. Biochim Biophys Acta 1998; 1370: 127–137
- Oliveira RG, Tanaka M, Maggio B. Many length scales surface fractality in monomolecular films of whole myelin lipids and proteins. J Struct Biol 2005; 149: 158–169
- Ostrowski SG, Van Bell CT, Winograd N, Ewing AG. Mass spectrometric imaging of highly curved membranes during Tetrahymena mating. Science 2004; 305: 71–73
- Ozerov IA, Zhinkina NA, Efimov AM, Machs EM, Rodionov AV. Feulgen-positive staining of cell nuclei in fossilized leaf and fruit tissues of the lower Eocene Myrtaceae. Bot J Linn Soc 2006; 150: 315–321
- Palade GE, Bruns RR. Structural modulation of plasmalemmal vesicles. J Cell Biol 1968; 37: 633–649
- Patterson WAI, Edwards KJ, Maguire DJ. Microscopic charcoal as a fossil indicator of fire. Quaternary Sci Rev 1987; 6: 3–23
- Poirier N, Derenne S, Balesdent J, Rouzaud J-N, Mariotti A, Largeau C. Abundance and composition of the refractory organic fraction of an ancient, tropical soil (Pointe Noire, Congo). Org Geochem 2002; 33: 383–391
- Poirier N, Derenne S, Rouzaud J-N, Largeau C, Mariottia A, Balesdent J, Maquet J. Chemical structure and sources of the macromolecular, resistant, organic fraction isolated from a forest soil (Lacadée, south-west France). Org Geochem 2000; 31: 813–827
- Poole AR, Howell JI, Lucy JA. Lysolecithin and cell fusion. Nature 1970; 227: 810–813
- Rao KS, Dave YS. Ultrastructure of active and dormant cambial cells in teak (Tectona grandis L. f). New Phytol 1983; 93: 447–456
- Robinson MS, Watts C, Zerial M. Membrane dynamics in endocytosis. Cell 1996; 84: 13–21
- Rosetti CM, Oliveira RG, Maggio B. The Folch-Lees proteolipid induces phase coexistence and transverse reorganization of lateral domains in myelin monolayers. Biochim Biophys Acta 2005; 1668: 75–86
- Satir B, Schooley C, Satir P. Membrane fusion in a model system: mucocyst secretion in Tetrahymena. J Cell Biol 1973; 56: 153–176
- Savić R, Azzam T, Eisenberg A, Maysinger D. Assessment of the integrity of poly(caprolactone)-b-poly(ethylene oxide) micelles under biological conditions: a fluorogenic-based approach. Langmuir 2006; 22: 3570–3578
- Scherz A, Rosenbach-Belkin V, Fisher JRE. Distribution and self-organization of photosynthetic pigments in micelles: implication for the assembly of light-harvesting complexes and reaction centers in the photosynthetic membrane. Proc Natl Acad Sci USA 1990; 87: 5430–5434
- Schönhut K, Vann DR, LePage BA. Cytological and ultrastructural preservations in Eocene Metasequoia leaves from the Canadian High Arctic. Am J Bot 2004; 91: 816–824
- Schumann U, Wanner G, Veenhuis M, Schmid M, Gietel C. AthPEX10, a nuclear gene essential for peroxisome and storage organelle formation during Arabidopsis embryogenesis. Proc Natl Acad Sci USA 2003; 100: 9626–9631
- Soltis PS, Soltis DE, Smiley CJ. An rbcL sequence from a Miocene Taxodium (bald cypress). Proc Natl Acad Sci USA 1992; 89: 449–451
- Somerville C, Browse J, Jaworski JG, Ohlrogge JB. Lipids. Biochemistry and molecular biology of plants, BB Buchanan, W Gruissem, RL Jones. American Society of Plant Biologists (Physiologists), Rockville 2002; 369–428
- Staehelin LA, Newcomb EH. Membrane structure and membraneous organelles. Biochemistry and molecular biology of plants, BB Buchanan, W Gruissem, RL Jones. American Society of Plant Biologists (Physiologists), Rockville 2002; 1–51
- Stefanowska M, Kuras M, Kacperska A. Low temperature-induced modifications in cell ultrastructure and localization of phenolics in winter oilseed rape (Brassica napus L. var. oleifera L.) leaves. Ann Bot 2002; 90: 637–645
- Takeo K, Uesaka I, Uehira K, Nishiura M. Fine structure of Cryptococcus neoformans grown in vitro as observed by freeze-etching. J Bacteriol 1973; 113: 1442–1448
- Tamm LK, Crane J, Kiessling V. Membrane fusion: a structural perspective on the interplay of lipids and proteins. Curr Opin Struct Biol 2003; 13: 453–466
- Taylor TN, Millay MA. Structurally preserved fossil cell contents. Trans Am Microsc Soc 1977; 96: 390–393
- Thiel G, Battey N. Exocytosis in plants. Plant Mol Biol 1998; 38: 111–125
- Thiel G, Kreft M, Zorec R. Unitary exocytotic and endocytotic events in Zea mays L. coleoptile protoplasts. Plant J 1998; 13: 117–120
- Tuma PL, Hubbard AL. Transcytosis: crossing cellular barriers. Physiol Rev 2003; 83: 871–932
- Ungermann C, Langosch D. Functions of SNAREs in intracellular membrane fusion and lipid bilayer mixing. J Cell Sci 2005; 118: 3819–3828
- Van der Woude WJ, Morré DJ, Bracker CE. Isolation and characterization of secretory vesicles in germinated pollen of Lilium longiflorum. J Cell Sci 1971; 8: 331–351
- Wang X. Plant cytoplasm preserved by lightning. Tissue Cell 2004; 36: 351–360
- Wang X. A chemical signal possibly related to physiology in fossil cells detected by energy dispersive X-ray microanalysis. Tissue Cell 2006; 38: 43–51
- Wang X. High temperature as a mechanism for plant cytoplasm preservation in fossils. Acta Geol Sin 2007a; 81: 183–193
- Wang X. Vivid fossils. Evol Life 2007b; 1: 20–22
- Wang X, Cui J. The first observation of plant fossil cells in China. Acta Geol Sin 2007; 81: 16–22
- Weise R, Kreft M, Zorec R, Homann U, Thiel G. Transient and permanent fusion of vesicles in Zea mays coleoptile protoplasts measured in the cell-attached configuration. J Membr Biol 2000; 174: 15–20
- Wong JL, Koppel DE, Cowan AE, Wessel GM. Membrane hemifusion is a stable intermediate of exocytosis. Dev Cell 2007; 12: 653–659
- Yang L, Huang HW. Observation of a membrane fusion intermediate structure. Science 2002; 297: 1877–1879
- Yang H, Huang Y, Leng Q, LePage BA, Williams CJ. Biomolecular preservation of Tertiary Metasequoia fossil Lagerstätten revealed by comparative pyrolysis analysis. Rev Palaeobot Palynol 2005; 134: 237–256
- Yatsu LY, Jacks TJ. Spherosome membranes. Plant Physiol 1972; 49: 937–943
- Yatsu LY, Jacks TJ, Hensarling TP. Isolation of spherosomes (oleosomes) from onion, cabbage, and cottonseed tissues. Plant Physiol 1971; 48: 675–682
- Zheng, G-C. 1996. Cell biology. 2nd ed. Beijing: Higher Education Publisher. p 633 ( in Chinese).