Abstract
The system IMINO transporter plays an essential role in the transport of proline and hydroxyproline in the intestine and kidney. Its molecular correlate has been identified and named SIT1 or IMINO (SLC6A20). Initial characterization of the transporter showed it to be Na+ and Cl−-dependent, but the stoichiometry remained unresolved. Using homology modeling along the structure of the bacterial leucine transporter LeuT, we identified two highly conserved Na+-binding sites and a putative Cl−-binding site. Mutation of all residues in the two proposed Na+-binding sites revealed that most of them were essential for uptake and completely inactivated the transporter. However, mutants A22V (Na+-binding site 1) and mutants S20A, S20G, S20G/G405S (Na+-binding site 2) were partially active and characterized further. Flux studies suggested that mutations of Na+-binding site 1 caused a decrease of the Na+-K0.5, whereas mutations of site 2 increased the K0.5. Mutation of Na+-binding site 1 also changed the ion selectivity of the IMINO transporter. IMINO actively translocates 36Cl− demonstrating that the proposed chloride binding site is used in the transporter. Accumulation experiments and flux measurements at different holding potentials showed that the transporter can work as a 2Na+/1Cl−-proline cotransporter. The proposed homology model allows to study mutations in IMINO associated with iminoglycinuria.
Introduction
The solute carrier family 6 of mammalian genomes is commonly known as the neurotransmitter transporter family. It comprises four branches, namely the monoamine transporter branch, the GABA transporter branch and the amino acid transporter branches (I) and (II) Citation[1]. In addition to neurotransmitters, members of this family mediate the uptake of metabolites such as taurine, creatine and a variety of amino acids. The family is highly significant for the physiology of mammalian organisms as illustrated by disorders associated with the malfunction of these transporters such as attention deficit hyperactivity disorder, hyperekplexia, creatine deficiency syndrome, obesity and Hartnup disorder Citation[2], Citation[3]. Transporters related to the SLC6 family have been identified in many organisms such as amphibia, insects, worms and bacteria Citation[4]. A high-resolution structure of a bacterial member of this superfamily has been resolved Citation[5]. The structure of the leucine transporter from Aquifex aeolicus (LeuT) is characterized by 12 transmembrane helices, four of which are intimately involved in ion and substrate translocation. The structure encloses a substrate binding site and two Na+ binding sites in the center. Helix 1 and 6 in particular line the translocation pore. In these two helices the secondary structure is interrupted in the center, allowing the contact of residues in this area of the protein with the two Na+-ions and the substrate leucine. Further contacts to substrate and Na+-ions are provided by helix 7 and 8. In addition to the contacts with the protein, the carboxylgroup of the substrate leucine forms one coordinating bond for Na+-binding site 1. Although the overall sequence similarity of LeuT with the mammalian transporters is less than 20%, a reliable alignment is straight-forward because highly-conserved residues can be found throughout the first 11 helices Citation[5], Citation[6]. This allows homology modeling of the mammalian transporters along the structure of the bacterial transporter. A number of studies using this approach have been published and appear to confirm the validity of the models (e.g., Citation[7–10]). As a result it appears likely that the overall protein fold of the mammalian transporters is similar to that of the bacterial transporter. Homology modeling has also revealed the presence of a chloride binding site in mammalian members of the SLC6 family Citation[11], Citation[12]. This site is filled by a glutamate residue in LeuT, but a cavity without a negatively charged side-chain is found in the mammalian transporters of the SLC6 family.
Although it was initially hypothesized that all members of the SLC6 family cotransport their substrates together with 2Na+ ions and 1Cl− ion, further studies showed that the stoichiometry in this family is in fact quite variable Citation[13], Citation[14]. The serotonin transporter, for example, cotransports 1Na+ and 1Cl− together with the substrate. The net translocation of one charge is compensated by the antiport of 1K+-ion Citation[13]. The two glycine transporters in the SLC6 family also show different stoichiometries: GlyT1 shows the canonical 2Na+ and 1Cl− cotransport, whereas GlyT2 cotransports 3Na+ and 1Cl−Citation[15]. The amino acid transporters B0AT1 and B0AT2, by contrast, are chloride-independent and cotransport only 1Na+Citation[16].
The initial characterization of the IMINO transporter showed it to be Na+ and Cl−-dependent Citation[17], Citation[18]. In contrast to other members of the SLC6 family, the apparent Km of chloride is very low being in the order of 1–2 mM. Na+ activation and Cl− activation of proline uptake resulted in hyperbolic relationships, suggesting the binding of 1Na+ and 1Cl− to the transporter. To reconcile these data with the inward currents observed during substrate transport we proposed that Cl− either binds to the IMINO transporter and is not cotransported, or that an electroneutral Cl−-exchange takes place similar to that proposed for the GABA transporter GAT1 Citation[19]. Physiological studies in brush-border membrane vesicles, which defined the IMINO transport activity, by contrast, gave evidence for a 2Na+-proline cotransport Citation[20]. Subsequent studies revealed the chloride-dependence of the IMINO transport activity Citation[21], Citation[22]. The IMINO transporter has recently been shown to be mutated in some cases of the inherited aminoaciduria iminoglycinuria Citation[23].
To clarify the mechanism of the transporter and to generate a valid homology model to analyse mutations occurring in iminoglycinuria, we investigated the functionality of the putative Na+-binding sites in the IMINO transporter and further investigated its mechanism. Our results suggest the presence of two functional Na+-binding sites and demonstrate Cl− cotransport, which is in agreement with the presence of a functional chloride binding site in the transporter.
Experimental
Oocytes and injections
Oocyte isolation and maintenance have been described in detail elsewhere Citation[24]. For expression, the IMINOB (slc6a20a) plasmid Citation[17] was linearized with NotI and transcribed in vitro using the T7 mMessage mMachine Kit (Ambion, Austin Texas, USA). In the mouse genome two IMINO genes are found, whereas in humans only one gene is found at the syntenic chromosomal locus. IMINOB indicates the transporter, which is strongly expressed in brain, kidney and intestine and corresponds to the human IMINO transporter SLC6A20. Human IMINO was isolated as described Citation[23]. Oocytes were each injected with 20 ng of cRNA encoding mouse IMINOB or human IMINO. Site-directed mutagenesis was carried out using the Stratagene QuikChangeII kit following the instructions of the manufacturer. The primers used for mutagenesis were synthesized according to the recommendations of the kit.
Flux studies
Oocyte transport measurements were carried out 3–6 days after injection as described in detail recently Citation[24]. Oocyte ringer ND96 (96 mM NaCl, 2 mM KCl, 1.8 mM CaCl2, 1 mM MgCl2, 5 mM N-(2-Hydroxyethyl)piperazine-1-ethanesulfonic acid (HEPES) titrated with NaOH to pH 7.4) was used in all transport assays unless indicated otherwise. N-methyl-D-glucamine chloride (NMDG-Cl) was used to replace NaCl, where indicated. Uptake of [14C]proline increased linearly with time for more than 30 min. As a result, uptake was determined using an incubation period of 10-20 min. Radiochemicals were purchased from Amersham Pharmacia Biotech (Castle Hill, Australia). To determine the ratio of Cl− to proline uptake, double-labelling experiments were carried out. Oocytes were incubated with 36Cl− (final concentration 10 mM) and [14C]proline (final concentration 1 mM) for 25 min. Subsequently, oocytes were dissolved and the amount of [14C]proline and 36Cl− uptake was determined by dual label scintillation counting. The 36Cl− preparation contained substantial amounts of Na+, due to titration of the H36Cl.
For the accumulation experiments, [14C]proline uptake was followed for up to 5h. Thin layer chromatography analysis of oocyte extracts showed that 95% of the total radioactivity was found after this time in the spot corresponding to a proline standard (data not shown). Accumulation experiments with overnight incubation showed significant accumulation also in non-injected control oocytes and were not used. The accumulation experiments were performed in buffers of different ionic composition. Buffer 1: 30 mM Na+/10 mM Cl− (70 mM NMDG-Pi (pH 7.4), 20 mM Na-gluconate, 10 mM NaCl, 0.2 mM KCl, 0.18 mM CaCl2, 0.1 mM MgCl2, 0.5 mM HEPES; titrated with NaOH to pH 7.4); buffer 2: 30 mM Na+/100 mM Cl− (70 mM NMDG-Cl (pH 7.4), 30 mM NaCl, 0.6 mM KCl, 0.6 mM CaCl2, 0.3 mM MgCl2, 1.5 mM HEPES; titrated with NaOH to pH 7.4); buffer 3: 100 mM NaCl (96 mM NaCl, 2 mM KCl, 1.8 mM CaCl2, 1 mM MgCl2, 5 mM HEPES; titrated with NaOH to pH 7.4). For each accumulation experiment 25 µM [14C]proline was added to the buffer followed by a 5h incubation period. Subsequently, the radioactivity in the supernatant and in the oocytes was determined. To calculate the accumulation, a volume ratio of 100 µl supernatant to 400 nl oocyte volume was used Citation[25]. Theoretical accumulation was calculated using electrochemical equations for ion gradients Citation[26], Citation[27]; intracellular ion concentrations (Na+=8 mM, Cl−=40 mM) were taken from Citation[28].
Construction of fusion proteins and surface expression
The cDNA of the IMINOB transporter was excised with EcoRI and subcloned in to pEGFPc3 (Clontech, Mountain View, California, USA). Constructs which showed the correct orientation were isolated and the cDNA of the EGFP-IMINOB transporter was amplified by PCR using the following primers: sense 5′ CCTAATACGACTCACTATAGGGCCACCATGGTGAGCAAG 3′ and antisense 5′ TCAGTTATCTAGATCCGGTGGATCCCGGGG 3′. The sense primer contained a T7 promoter (underlined) to allow in vitro transcription of the resulting PCR product. After amplification for 30 cycles the PCR product was purified and used for in vitro transcription. The cRNA was extended by polyadenylation using a poly(A)tailing kit (Ambion, Austin, Texas, USA). After purification the cRNA was injected into oocytes (25 ng) and surface expression was analysed after five days of incubation using a Biorad Radiance 2000 inverted confocal microscope. The EGFP-IMINO fusion protein was functionally active showing 44±7% transport activity of the wild-type transporter. We have previously shown that the laser light does not penetrate far into the oocyte because of its egg yolk content Citation[29]. As a result membrane proteins residing in the endoplasmic reticulum or Golgi apparatus are not visible. Only proteins expressed at the surface are excited by the laser light. The fluorescence intensity is therefore proportional to the surface expression Citation[29].
Electrophysiological recordings
Amino acid induced currents were analyzed by two-electrode voltage clamp recording. The recordings were performed with microelectrodes connected to a Geneclamp 500B electronic amplifier in Canberra (Axon Instruments, Union City, CA, USA) or to a TEC-03 amplifier in Freising (npi electronic, Tamm, Germany). The output signal was amplified ten times and filtered at 50 Hz. Data were sampled at 3Hz using a Digidata 1322A and pCLAMP or Cellworks software (Axon Instruments, Union City, CA, USA and npi electronic, Tamm, Germany). Once a stable membrane potential was reached under current clamp conditions, the amplifier was switched to voltage clamp mode holding the oocytes at −50 mV. Oocytes were superfused with ND96 containing different substrates as indicated. A complete change of the bath to a new solution was accomplished in about 10 sec. Current-voltage relationships were measured immediately before and 20–30 sec after substrate application in the potential range of −160 to +20 mV (20 mV steps). The current generated by the transporter at a given membrane potential was calculated as the difference of the currents measured in the presence and the absence of substrate.
Uptake of [14C]proline under voltage-clamp conditions was performed in the bath (volume 200 µl) of the electrophysiological setup. Superfusion was stopped for the time of the experiment. Uptake was initiated by addition of 20 µl [14C]proline (1 mM) to the bath. After 10 min, oocytes were washed by resuming superfusion. After removal of the microelectrodes oocytes were immediately transferred into scintillation vials.
Modelling
The I-TASSER server Citation[30] was used to produce a homology model of the IMINO transporter. The model was checked using the WHAT IF program Citation[31]. The model contained the canonical transmembrane helices and cavities that matched predicted binding sites for sodium ions and proline. These entities were built into the model on the basis of Na+ and leucine binding to LeuT. A further cavity, not found in LeuT was observed that was lined with hydrogen bond donors. This was interpreted as the chloride binding site. A chloride ion was placed in this space in accordance with recent results Citation[11], Citation[12].
Molecular Dynamics Simulation
The structural stability of the model was tested by simulation in a physically realistic membrane environment. The IMINO model including bound ions and proline was placed in a 1-palmitoyl-2-oleoyl-sn-glycero-3-phosphoethanolamine (POPE) membrane patch 96 Å to a side. Lipid molecules overlapping with the protein were removed. Additional water molecules were placed above and below the membrane so as to ensure complete solvation of the protein. Sodium and chloride ions (0.1 M) were introduced into the extramembrane space. Histidine residues were protonated in accordance with their local hydrogen-bonding environment. The complete system had overall dimensions of 96×96×80 Å and comprised 61,109 atoms. All simulations were computed with molecular dynamics simulation software NAMD Citation[32]. The CHARMM-27 (Chemistry at Harvard Macromolecular Mechanics) forcefield for lipids was used for POPE groups Citation[33]. The CHARMM-22 forcefield with cross-term map correction Citation[34], Citation[35] was used for protein. The system was maintained at a pressure of 1 atmosphere using the Nosé-Hoover Langevin piston algorithm. The system was subjected to 10,000 steps of energy minimization to reduce unfavorable contacts prior to 10 ns of Langevin dynamics at 368 K. A switching function was used to smoothly truncate non-bonded interactions to zero from 10–12 Å. Multiple timestep integration was used with bonded, non-bonded and long-range interactions calculated every 1, 2, and 4 fs, respectively. The Particle Mesh Ewald method was used for the calculation of long-range electrostatic forces. During the first 1 ns the protein non-hydrogen atoms were restrained to their initial positions with a force constant of 1 kcal/Å2, allowing the surrounding lipid and water molecules to adjust to the protein. The stability of the proline and ion binding sites, and the transmembrane helices were monitored throughout the simulation.
Results
Homology model and simulation of the IMINO transporter
To study Na+ binding and transport in the IMINO transporter we generated a homology model using the LeuT transporter as a template. The model reproduces the overall topology of the LeuT transporter and is consistent with the related serotonin transporter model produced by Forrest et al. Citation[11]. No serious structural anomalies were identified by the WHAT IF server. The IMINO sequence has several insertions relative to the LeuT transporter, which cannot be modeled reliably. However, these are extracellular or intracellular loops which are not involved in the transport mechanism. The transmembrane helices are well conserved and we therefore expect these regions of the model to be fairly accurate (A). The alignment of the primary sequence of the IMINO transporter with LeuT is shown in .
Figure 1. Homology model of the mouse IMINO transporter. The I-TASSER server was used to produce a homology model of the IMINO transporter. (A) Depicted is the backbone of the peptide-chain, secondary structures are emphasized. (B) The model contained the canonical transmembrane helices and cavities that matched predicted binding sites for sodium ions and proline. These entities were built into the model on the basis of Na+ and leucine binding to LeuT. Na+-binding site 1 is highly conserved, while Na+-binding site 2 shows less conservation. A further cavity, not found in LeuT, was observed that was lined with hydrogen bond donors. This was interpreted as the chloride binding site. A chloride ion was placed in this space.
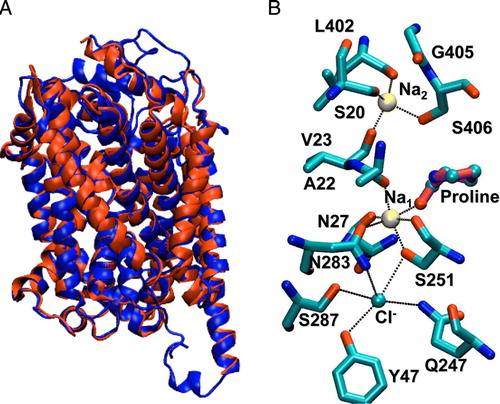
Figure 2. Structural alignment of IMINO and LeuT. The homology model was generated as described in the methods section. The resulting sequence alignment is shown. Fully conserved residues are indicated by an asterisk and similar residues are indicated by a dot. Residues on a grey background are involved in Na+1-binding. Residues on a black background are involved in Na+2-binding. Bold letters indicate residues involved in Cl− binding. Transmembrane helices are underlined and numbered.
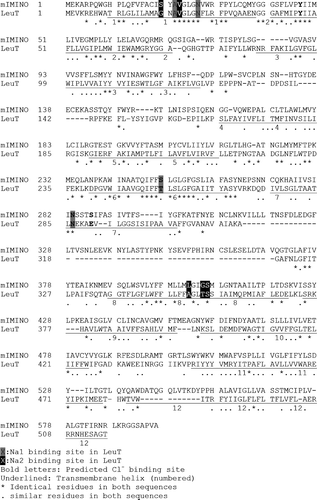
There are two Na+ binding sites in the LeuT transporter and as a result the homology model also contains two putative binding sites (B). In the IMINO transporter the predicted residues forming Na+ binding site 1 are highly conserved (LeuT residues given in square brackets, atoms involved are given in round brackets): Ala22 (O) [LeuT: Ala22 (O)], Asn27 (O▵), [LeuT: Asn27 (O▵)], Ser251 (O and Oγ), [LeuT: Thr254 (O and Oγ)], Asn283 (O▵?) [LeuT: Asn286 (O▵?)] and the substrate proline [LeuT: leucine]. The putative Na2-binding site is less conserved involving residues Ser20 (O) [LeuT: Gly20 (O)], Val23 (O), [LeuT: Val23 (O)], Leu402 (O), [LeuT: Ala351 (O)], Gly405 (no contact) [LeuT: Thr354 (Oγ)]? and Ser406 (Oγ) [LeuT: Ser355 (Oγ)]. Thus Na+ binding site 1 is completely conserved apart from a conservative threonine to serine exchange. In the less conserved putative Na+ binding site 2 there is a notable swap of two residues, combined with a conservative replacement: Ser20 of IMINO is located at the equivalent position to Gly20 of LeuT; and Gly405 of IMINO corresponds to Thr354 of LeuT. As in the LeuT transporter, this model suggests that Na1 is hexagonally coordinated, whereas the putative Na2 shows tetrahedral coordination. Both Na+ binding sites could therefore be functional in the IMINO transporter.
A further feature of the homology model is the presence of a cavity adjacent to the Na1 binding site. This has been shown to form a chloride-binding site in the GABA transporter GAT1 Citation[12] and the serotonin transporter SERT Citation[11]. In our model it is lined by five residues that can donate hydrogen bonds to a negatively charged entity at this site. These are Tyr47 (Oε-H), Gln247 (Nε-H), Ser251 (Oγ-H), (Oγ251 also interacts with Na1), Asn283 (N▵-H) and Ser287 (Oγ-H). The interactions of the transporter with bound ions and substrate proline as proposed above were maintained throughout the time course of the simulation. Furthermore, the transmembrane helices maintained their secondary structure and topological arrangement throughout the simulation. During the first 5 ns of the simulation, the root mean square deviation of the model increased to about 4.5 Å – mainly due to the fluctuations of the surface loops – after which it stabilized at this value.
Functional properties of Na+-binding site mutants
In order to test the role of the two proposed Na+ binding sites in the IMINO transporter we mutated all residues involved. Conservative exchanges were made when the side-chain was involved in Na+-binding, whereas positive or negative charges were introduced in the side-chain when the backbone contacted the Na+-ion. These extra charges introduce an additional electrostatic interaction, causing repulsion or attraction of Na+-ions. To address the differences of Na+ binding site 2 between LeuT and IMINO, we also generated mutations S20G and S20G/G405S. The single mutation reverts position 20 to the corresponding sequence of LeuT. The double mutation reverts the swap of these two residues between LeuT and IMINO, but retains the serine instead of a threonine in LeuT. Not unexpectedly, most replacements resulted in inactive transport proteins (A, 3C), indicating that the selected residues are critical for transporter function. Some of these mutations, however, caused loss of function due to a lack of surface expression (), such as V23E and G405A. Nevertheless, we found mutations that were partially active and showed significant surface expression in each putative binding site. Mutation S20G, for instance, had similar activity as the wild type. The mutants A22V, S20A and the double mutant S20G/G405S showed 40–50% transport activity (at a substrate concentration of 100 µM) (A, 3C). Electrophysiological recordings performed with representative mutants from both binding sites confirmed the flux data, but the currents of S20A and A22V (measured at a substrate concentration of 1 mM) did not exceed 20% of those of the wild type. Small currents were also observed for the replacements S287A and S406A (B, 3D). Consistent with a critical role of Ser251 and Ser287 in chloride binding and/or protein stability, the two mutants showed very little activity and reduced surface expression. All partially active mutants were Na+-dependent (B, 3D), as evidenced by a loss of transport activity when NaCl was replaced by NMDG-Cl.
Figure 3. Proline uptake and currents of wild type and IMINO mutants. (A) and (C): Oocytes were injected with cRNA (20 ng) encoding wild type or mutant IMINO transporters. After an incubation period of 4 days, uptake of 100 µM [14C]proline was measured over an incubation period of 20 min. Each bar represents the mean±SD of 10 oocytes; the experiment was repeated three times with similar results. Wild-type activity ranged from 140–180 pmol/20 min per oocyte. (B) and (D): After an incubation period of five days oocytes were superfused with 1mM proline in the presence or absence (NMDG-Cl) of Na+ and the resulting currents were recorded (n=7 for each mutant, the experiment was performed with three independent batches of oocytes, wild-type currents were in the range of 50–150 nA).
![Figure 3. Proline uptake and currents of wild type and IMINO mutants. (A) and (C): Oocytes were injected with cRNA (20 ng) encoding wild type or mutant IMINO transporters. After an incubation period of 4 days, uptake of 100 µM [14C]proline was measured over an incubation period of 20 min. Each bar represents the mean±SD of 10 oocytes; the experiment was repeated three times with similar results. Wild-type activity ranged from 140–180 pmol/20 min per oocyte. (B) and (D): After an incubation period of five days oocytes were superfused with 1mM proline in the presence or absence (NMDG-Cl) of Na+ and the resulting currents were recorded (n=7 for each mutant, the experiment was performed with three independent batches of oocytes, wild-type currents were in the range of 50–150 nA).](/cms/asset/ee9cd9d4-4484-417d-b04a-0650f4a095eb/imbc_a_415175_f0003_b.gif)
Figure 4. Surface expression of IMINO mutants. IMINO wild type and its site-specific mutants were cloned into an N-terminal EGFP fusion vector. Subsequently, all constructs were amplified by PCR, transcribed in vitro and expressed in oocytes. Surface localization was analysed by confocal microscopy after five days of expression. The laser light does not penetrate the oocyte; as a result only transporters at the surface are excited and show fluorescence (n=7 for each mutant, the experiment was performed with 2–3 independent batches of oocytes).
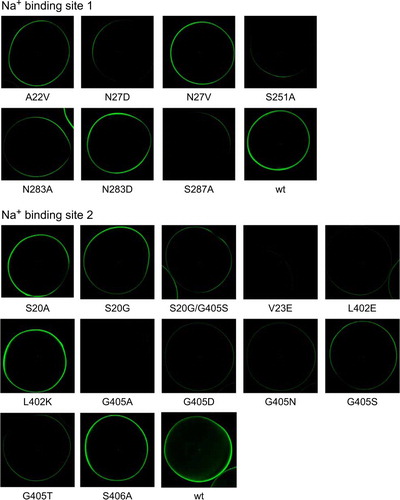
We reasoned that a mutation of the putative Na+-binding sites should alter the affinity for Na+ or change ion specificity. Previously, we found that Na+ activation of proline transport occurred with an apparent K0.5-value of 22±2 mM Citation[17]. The resulting curves had a hyperbolic shape indicating the presence of one Na+ binding site. Repetition of these experiments with more experimental points at low Na+ concentration revealed a sigmoidal shape with a K0.5 of 11±0.1 mM (Hill coefficient n=2.0) (). Mutation of Na+ binding site 1 (A22V) reduced the sigmoidity (n=1.3) and resulted in a lower apparent K0.5 for Na+ of 3.2±0.7 mM ( and ). Maximum currents were about 13% of the wild type. Mutation of Na+ binding site 2 (S20G and S20G/G405S), by contrast, increased the K0.5 of Na+ from 13±3 mM (wt) to 28±10 (S20G) and 44±5 mM (S20G/G405S) when NaCl was replaced by LiCl and slightly less when NaCl was replaced NMDG-Cl (). This result suggests that both Na+-binding sites determine the Na+-K0.5 in the IMINO transporter. The conservative replacement S20A resulted in little change of the Na+ activation of proline transport, suggesting that the side chain of serine 20 is unlikely to coordinate the Na+-ion. This was confirmed by molecular dynamics simulations of the homology model in the presence of proline, the two Na+-ions and the Cl−-ion. B shows the equilibrated structure of the IMINO model in which the side chain of serine 20 points away from Na+-binding site 2 (the hydroxylgroup points towards the label [L402]).
Figure 5. Activation of proline-induced currents by sodium ions. Oocytes were injected with wild type, A20V and S20A IMINO cRNA. After an incubation period of five days oocytes were superfused with 0.5 mM proline in the presence of Na+ ranging from 1–30 mM and the resulting currents were recorded. The left scale is used for wild-type currents, the right scale for the two mutated transporters. Each datapoint represents the mean±SEM of n=7 oocytes. The experiment was repeated with two independent batches of oocytes.
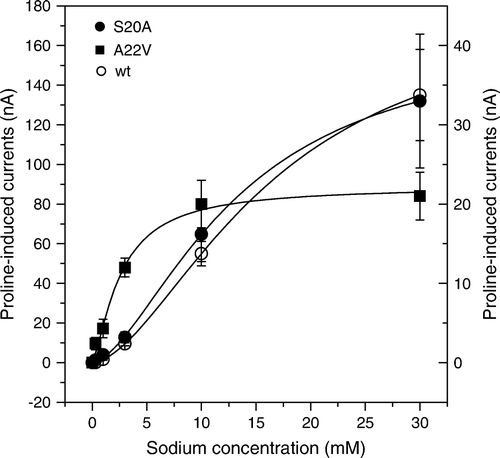
Table I. Changes of the K0.5 for Na+ associated with mutations in Na+-binding sites. Oocytes were injected with cRNA (20 ng) encoding the IMINO transporter. After an incubation period of 4 days, uptake of 100 µM [14C]proline was measured over an incubation period of 15 min at different Na+ concentrations. LiCl or NMDG-Cl was used to replace Na+ as indicated in the table. The number of independent experiments (e) used to derive the K0.5 is indicated.
The functional impairment of mutants A22V and S20A suggested that they could provide further insight into ion binding. In the wild-type, proline-induced currents were almost entirely Na+-dependent ( and ). When Na+ was replaced by Li+ small proline-induced currents were observed in hyperpolarized oocytes. Slightly bigger currents were observed when Cl− was replaced by gluconate (). The wild-type IMINO transporter showed inward-currents of 5–10 nA when Na+ was replaced by Li+, equivalent to about 5% of the currents observed in the presence of NaCl (A). In contrast to recent observations with GABA transporter GAT1 Citation[7], no big differences of the Na+-K0.5 were observed regardless whether Na+ was replaced by NMDG+ or Li+ (). The Hill-coefficient, however, was close to 2 when NMDG-Cl was used as a replacement for NaCl but only 1 when LiCl was used. In oocytes expressing the S20A mutation all currents were reduced but the ratio of the currents under the three ionic conditions was similar (B, 6C). This confirmed that the side-chain of S20 did not participate in ion binding. Mutation of alanine 22 to valine (A22V), by contrast, changed the ratio of the currents. The currents in LiCl and NMDG-Cl were similar or higher than in the wild type, whereas the current in NaCl was strongly reduced (D). Thus it appears that cation selectivity is reduced in the A22V mutation. Analysis of the current-voltage relationship showed that currents did not reverse in the range analyzed (up to +20 mV), extrapolation of the curve in the presence of NaCl suggests that reversal could occur above +50 mV. This indicates that the observed inward currents are carried by an ion with a strongly positive reversal potential, i.e., Na+-ions.
Figure 6. Current-voltage relationships for IMINO wild type and mutants A20V and S20A. Current-voltage relationships were recorded in Na+-containing ND96 buffer or in modified ND96 in which Na+ was replaced by LiCl or Na-gluconate (NaGlc) in oocytes expressing wild-type IMINO (A) or its mutants S20A (C) and A22V (D). To compare substrate-induced currents in the wild type and its mutants, currents at a holding potential of −50 mV are depicted on an interrupted scale (B). All measurements show the mean of three experiments with n > 8 oocytes each.
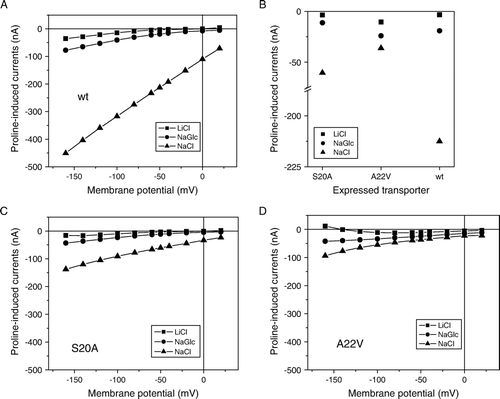
Expression of IMINO in oocytes results in a general depolarization of the oocyte Citation[18], caused by a ‘leak’ current. These tonic currents became apparent when NaCl was replaced by NMDG-Cl and their size is directly proportional to the Na+ concentration difference (A). The leak currents were blocked by mutations in both Na+-binding sites, suggesting that they are an integral part of the IMINO transport mechanism (B). For instance N27V (Na+-binding site 1) and G405S (Na+-binding site 2) showed significant surface expression (), but did not display leak currents. Both mutants, however, showed residual transport activity. Thus mutations in both Na+ binding sites can block the Na+ leak current.
Figure 7. Na+-dependent leak currents in the IMINO transporter. Oocytes were injected with wild type or mutant IMINO cRNA (20 ng). After an incubation period of five days oocytes were superfused with ND96 (96 mM Na+) or modified ND96 in which NaCl was replaced by NMDG-Cl in the absence of substrate. The difference of the holding current is depicted. (A) Outward current as a function of the difference of the Na+-concentration in the bath (n=19). (B) Comparison of the outward current between wild-type, mutants and non-injected oocytes (n=7).
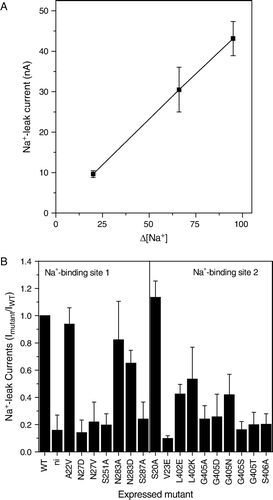
Transport mechanism
The modeling suggested the presence of a conserved chloride-binding site in the transporter. To find out whether this chloride binding site has a structural role or actively translocates chloride, we determined the uptake of 36Cl− in the absence and presence of [14C]proline. Addition of saturating amounts of proline stimulated 36Cl− uptake into oocytes expressing the IMINO transporter. On average 1.98±0.3 mol Cl− (n=70 oocytes, each dual label) were cotransported with each mol proline, demonstrating active uptake of chloride during the transport process (A). In the nominal absence of Na+, chloride transport was strongly reduced. The remaining activity could be explained by contaminating Na+-ions in the radioactive preparation (A). The transport inactive mutant N27V displayed minimal uptake of 36Cl−, demonstrating that Cl− uptake was not mediated by oocyte endogenous pathways (A).
Figure 8. Chloride cotransport and electrogenicity of the IMINO transporter. Oocytes were injected with 20 ng IMINO cRNA. (A) After an incubation period of five days uptake of 36Cl− (grey bars) and [14C]proline (black bars) was measured over a period of 30 min in the presence (100 mM) and the nominal absence (low) of Na+ (NMDG replacement) and the amount of both isotopes was determined in individual oocytes by dual-label scintillation counting. The 36Cl− preparation contained significant amounts of Na+, which can be estimated from the difference of 36Cl− and [14C]proline uptake in the wild type versus the N27V mutant. (B) Uptake of 100 µM [14C]proline was performed under voltage-clamp and current clamp (cc) conditions over a period of 10 min and the amount of accumulated amino acid was determined by liquid scintillation counting.
![Figure 8. Chloride cotransport and electrogenicity of the IMINO transporter. Oocytes were injected with 20 ng IMINO cRNA. (A) After an incubation period of five days uptake of 36Cl− (grey bars) and [14C]proline (black bars) was measured over a period of 30 min in the presence (100 mM) and the nominal absence (low) of Na+ (NMDG replacement) and the amount of both isotopes was determined in individual oocytes by dual-label scintillation counting. The 36Cl− preparation contained significant amounts of Na+, which can be estimated from the difference of 36Cl− and [14C]proline uptake in the wild type versus the N27V mutant. (B) Uptake of 100 µM [14C]proline was performed under voltage-clamp and current clamp (cc) conditions over a period of 10 min and the amount of accumulated amino acid was determined by liquid scintillation counting.](/cms/asset/010b4662-90dd-4dcd-97ef-236d283f202d/imbc_a_415175_f0008_b.gif)
The transport of close to 2 mol equivalents chloride for each substrate molecule, points to an electroneutral transport mechanism. Previously, we reported a charge/substrate flux ratio of 1:1 Citation[17]. To further investigate whether the transporter is electrogenic or electroneutral, we measured the uptake of [14C]proline under voltage-clamp condition at three different holding potentials (B). Proline uptake increased about five-fold from −30mV to −60mV but then remained at this level when the membrane potential was set to −90mV. Oocytes that were not held at a specific voltage showed uptake similar to the −30 mV level.
The results of the structure-function analysis suggested that IMINO has two functional Na+-binding sites and a functional Cl− -ion binding site. To further evaluate the coupling between ion and substrate transport, we performed accumulation studies in Xenopus oocytes (). We considered five possible models for proline uptake by the IMINO transporter: Model 1 (1Na+-1Cl− symport), Model 2 (1Na+ symport-Cl− exchange), Model 3 (2Na+-1Cl− symport), Model 4 (2Na+-2Cl− symport), and Model 5 (2Na+ symport-Cl− exchange). Due to the size of the oocytes, we determined proline accumulation in 5-h incubation periods, during which endogenous uptake and metabolism can be neglected Citation[36]. Models 2 and 5 (both involving chloride exchange) did not predict the observed changes to proline accumulation in response to alterations of the chloride concentration. Model 1 and 2 were refuted because experimentally determined accumulation exceeded predictions under all three ionic conditions. Model 4 was inconsistent with the experimental data, because it did not predict accumulation > 1 at 30 mM Na+ and 10 mM Cl−. Only Model 3 predicted accumulation ratios that were in agreement with or above the experimental data under all ionic conditions. Mutants S20A and A22V showed similar accumulation ratios as the wild type ().
Table II. Accumulation of [14C]proline in oocytes expressing the mouse IMINO transporter. Oocytes were injected with cRNA (20 ng) encoding the IMINO transporter. After an incubation period of 4 days, uptake of 25 M [14C]proline was measured over an incubation period of 5 hours. Each experiment (columns wt, S20A and A22V) shows the mean observed accumulation of n=10 oocytes each in ‘e’ experimental repeats. These values are compared to accumulation ratios predicted by models 1–5. Cotransported Cl− are preceded by a /, Cl− undergoing exchange are shown in brackets followed by X.
Modeling of a mutation occurring in iminoglycinuria
Recently, we identified a mutation in the human IMINO transporter (T199M) that causes iminoglycinuria and impairs the transport Citation[23]. In order to understand the mutation, we used the homology model to predict the position of this residue (A). The residue T199 is located on the outside of the protein in helix 5 and is in close contact with M401 in helix 8. Helix 8 contains critical residues involved in Na+-binding and it appears likely that its interface to helix 5 changes during transport. In order to test whether the location of residue T199 is correctly predicted by the model, we mutated residue M401 to threonine which should remove stereochemical constraints introduced by the bulkier methionine residue. In agreement with this notion, the double mutation regained significant activity, particularly when expressed for up to five days (B).
Figure 9. Modelling of the Iminoglycinuria associated mutation T199M. (A) Depiction of the spatial arrangement of T199 and M401 in the IMINO transporter. The side-chain of T199 is in close contact with M401. M401 is located in helix 8 and is close to Na+2 (magenta) and the substrate proline (orange). The Na+1 is shown in blue. (B) Oocytes were injected with cRNA (20 ng) encoding wild type or mutant IMINO transporters. After an incubation period of 3 and 5 days, uptake of 100 µM [14C]proline was measured over an incubation period of 10 min. Each bar represents the mean±SD of 10 oocytes, the experiment was repeated three times with similar results.
![Figure 9. Modelling of the Iminoglycinuria associated mutation T199M. (A) Depiction of the spatial arrangement of T199 and M401 in the IMINO transporter. The side-chain of T199 is in close contact with M401. M401 is located in helix 8 and is close to Na+2 (magenta) and the substrate proline (orange). The Na+1 is shown in blue. (B) Oocytes were injected with cRNA (20 ng) encoding wild type or mutant IMINO transporters. After an incubation period of 3 and 5 days, uptake of 100 µM [14C]proline was measured over an incubation period of 10 min. Each bar represents the mean±SD of 10 oocytes, the experiment was repeated three times with similar results.](/cms/asset/d08ff5a2-5611-48ad-9717-371069933c41/imbc_a_415175_f0009_b.jpg)
Discussion
Previously, we and others found that substrate translocation in the IMINO transporter is accompanied by inward currents and that the transporter is Na+ and Cl− -dependent Citation[17], Citation[18]. These initial studies could not resolve three main questions, which are addressed in this study: (i) What is the Na+ cotransport stoichiometry of the IMINO transporter? (ii) Does IMINO mediate chloride translocation or is it activated by chloride binding?, and (iii) Is LeuT a useful template to analyse mutations occurring in the IMINO transporter?
Evidence from a variety of methods points to a mechanism involving active cotransport of 2Na+-ions and 1Cl− together with the substrate. First, Na+-activation curves are sigmoidal. Second mutation of residues in both predicted Na+-binding sites alter Na+-activation or ion selectivity. Mutations in binding site 1 reduced the K0.5 for Na+ of the transporter, while mutations in Na+-binding site 2 increased the K0.5. Thus both binding sites appear to have different affinities, which is consistent with the observed sigmoidal Na+-dependence. Third, we could detect a putative binding site for Cl− in the structure of IMINO that is close to Na+ binding site 1. All residues around the Cl− binding site are well conserved and critical for protein function and folding. Active substrate-dependent uptake of 36Cl− unambiguously demonstrated the use of this site.
To further substantiate the structure-function data we used accumulation experiments to test five possible mechanisms: (Model 1) 1Na+-1Cl− cotransport, (Model 2) 1Na+ symport-Cl− exchange, (Model 3) 2Na+-1Cl− symport, (Model 4) a 2Na+-2Cl− symport, (Model 5) 2Na+ symport-Cl− exchange. The experiments are in agreement with model (3) under all conditions. Models (1) and (2) are ruled out, because accumulation above predicted levels would be thermodynamically impossible. Model (2) and (5) are not consistent with the data, for they do not predict a change of accumulation in response to changes of the chloride concentration. A hybrid model in which chloride uptake occurs as exchange at least some of the time, cannot be ruled out, however. At high substrate-motive forces the experimental accumulation stayed well below the theoretical maximum predicted by model (3). It appears likely that substrate will accumulate close to the membrane during transport, resulting in a high proportion of exchange reaction. As a result net uptake can cease quickly and theoretical accumulation will be reached very slowly as demonstrated in the case of the lac permease and the glycine transporter GlyT1B Citation[37], Citation[38]. It appears possible that Cl− -ions participate in this exchange reaction, explaining why the flux experiments showed an uptake of two 36Cl− per proline molecule. It also explains why substrate-induced currents of some mutants were smaller than expected from the uptake of labelled proline. The accumulation appeared unchanged in the mutants suggesting that they have the same cotransport stoichiometry.
Similar to other branches of the SLC6 family the stoichiometries in the amino acid transporter branch (II) are variable. Both B0AT1 and B0AT2 appear to have only 1 functional Na+-binding site Citation[16], Citation[39–41], whereas IMINO conforms to the consensus stoichiometry in this family, which is a 2Na+/1Cl− cotransport Citation[42]. Cotransport of chloride might allow compensation for the electrostatic repulsion of the two Na+ ions. Accordingly, the chloride-independent members of the SLC6 family B0AT1 and B0AT2 have retained a less conserved chloride-binding site, which lacks two hydroxyl-groups in each case.
We also investigated whether mutation of Na+-binding site 2 would allow occupation by Li+ as observed in the GABA transporter GAT1 Citation[7]. Neither S20G, nor S20G/G405S tolerated Li+ as a replacement for Na+, supporting that an aspartate residue was required at position 405 for Li+ binding. The G405D mutation was found to be completely inactive and therefore could not be analyzed. The partial rescue of the G405S mutation by the additional exchange S20G is consistent with the proposed structure of Na+-binding site 2 of the IMINO transporter. The difference of the Hill-coefficient between NMDG-Cl replacement and LiCl replacement suggests an interaction between Li+-ions and the transporter. This interaction is likely to occur at Na+ -binding site 1 because it was observed in the wild type and in Na+ -binding site 2 mutations. In contrast to LeuT, the proposed Na+-binding site 2 shows tetrahedral coordination in the IMINO transporter model. This suggests that this binding site is less stable than in LeuT. It should be pointed out, however, that ATB0,+ also has only four coordination bonds and is thought to cotransport 2Na+ with the substrates Citation[43].
Mutation of residues in both Na+-binding sites significantly reduced substrate-independent leak currents associated with expression of the IMINO transporter. It thus appears likely that these currents are mediated by the transporter itself and are not generated by upregulation of oocyte endogenous conductances.
The mechanism of the IMINO transporter as outlined in this publication is in agreement with earlier studies in brush-border membrane vesicles and brain synaptosomes Citation[22], Citation[44]. This confirms that the cloned IMINO transporter is indeed the molecular correlate of the system IMINO defined functionally in the apical membrane of renal and intestinal epithelial cells. Recently, we identified a mutation in the human IMINO transporter (T199M) associated with the rare disorder iminoglycinuria (OMIM 242600) Citation[23]. The mutation changed the maximum velocity and increased the Hill-coefficient of Na+ activation. The homology model places T199 in helix 5 in contact with residue M401 in helix 8. This contact could explain the effect of the mutation on Na+ activation, as helix 5 is not directly involved in Na+ transport. The successful application of the homology model to Na+ translocation and mutant analysis demonstrates that it will help to understand mutations causing human disorders.
Acknowledgements
This work is supported by grants from the Australian Research Council (ARC DP0877897) and the National Health and Medical Research Council (NHMRC 402730). The authors thank Sonja Kowalczuk and Stephen Fairweather for experimental support. Declaration of interest: The authors report no conflicts of interest. The authors alone are responsible for the content and writing of the paper.
References
- Bröer S. The SLC6 orphans are forming a family of amino acid transporters. Neurochem Int 2006; 48(6–7)559–567
- Hahn MK, Blakely RD. The functional impact of SLC6 transporter genetic variation. Annu Rev Pharmacol Toxicol 2007; 47: 401–441
- Bröer S. Apical transporters for neutral amino acids: physiology and pathophysiology. Physiology (Bethesda) 2008; 23: 95–103
- Boudko DY, Kohn AB, Meleshkevitch EA, Dasher MK, Seron TJ, Stevens BR, Harvey WR. Ancestry and progeny of nutrient amino acid transporters. Proc Natl Acad Sci USA 2005; 102(5)1360–1365
- Yamashita A, Singh SK, Kawate T, Jin Y, Gouaux E. Crystal structure of a bacterial homologue of Na(+)/Cl(−)-dependent neurotransmitter transporters. Nature 2005; 437: 215–223
- Beuming T, Shi L, Javitch JA, Weinstein H. A comprehensive structure-based alignment of prokaryotic and eukaryotic neurotransmitter/Na+ symporters (NSS) aids in the use of the LeuT structure to probe NSS structure and function. Mol Pharmacol 2006; 70(5)1630–1642
- Zhou Y, Zomot E, Kanner BI. Identification of a lithium interaction site in the gamma-aminobutyric acid (GABA) transporter GAT-1. J Biol Chem 2006; 281(31)22092–22099
- Vandenberg RJ, Shaddick K, Ju P. Molecular basis for substrate discrimination by glycine transporters. J Biol Chem 2007; 282(19)14447–14453
- Zhang YW, Rudnick G. The cytoplasmic substrate permeation pathway of serotonin transporter. J Biol Chem 2006; 281(47)36213–36220
- Forrest LR, Zhang YW, Jacobs MT, Gesmonde J, Xie L, Honig BH, Rudnick G. Mechanism for alternating access in neurotransmitter transporters. Proc Natl Acad Sci USA 2008; 105(30)10338–10343
- Forrest LR, Tavoulari S, Zhang YW, Rudnick G, Honig B. Identification of a chloride ion binding site in Na + /Cl -dependent transporters. Proc Natl Acad Sci USA 2007; 104(31)12761–12766
- Zomot E, Bendahan A, Quick M, Zhao Y, Javitch JA, Kanner BI. Mechanism of chloride interaction with neurotransmitter:sodium symporters. Nature 2007; 449(7163)726–730
- Rudnick G. Bioenergetics of neurotransmitter transport. J Bioenerg Biomembr 1998; 30(2)173–185
- Bröer S. Adaptation of plasma membrane amino acid transport mechanisms to physiological demands. Pflugers Arch 2002; 444(4)457–466
- Supplisson S, Roux MJ. Why glycine transporters have different stoichiometries. FEBS Lett 2002; 529(1)93–101
- Bröer A, Tietze N, Kowalczuk S, Chubb S, Munzinger M, Bak LK, Bröer S. The orphan transporter v7-3 (slc6a15) is a Na + -dependent neutral amino acid transporter (B0AT2). Biochem J 2006; 393(Pt 1)421–430
- Kowalczuk S, Bröer A, Munzinger M, Tietze N, Klingel K, Bröer S. Molecular cloning of the mouse IMINO system: an Na + - and Cl − -dependent proline transporter. Biochem J 2005; 386(Pt 3)417–422
- Takanaga H, Mackenzie B, Suzuki Y, Hediger MA. Identification of Mammalian Proline Transporter SIT1 (SLC6A20) with Characteristics of Classical System Imino. J Biol Chem 2005; 280(10)8974–8984
- Loo DD, Eskandari S, Boorer KJ, Sarkar HK, Wright EM. Role of Cl− in electrogenic Na + -coupled cotransporters GAT1 and SGLT1. J Biol Chem 2000; 275(48)37414–37422
- Stevens BR, Wright EM. Kinetics of the intestinal brush border proline (Imino) carrier. J Biol Chem 1987; 262(14)6546–6551
- Chesney RW, Zelikovic I, Budreau A, Randle D. Chloride and membrane potential dependence of sodium ion-proline symport. J Am Soc Nephrol 1991; 2(4)885–893
- Kanner BI, Sharon I. Active transport of L-proline by membrane vesicles isolated from rat brain. Biochim Biophys Acta 1980; 600(1)185–194
- Bröer S, Bailey CG, Kowalczuk S, Ng C, Vanslambrouck JM, Rodgers H, Auray-Blais C, Cavanaugh JA, Bröer A, Rasko JE. Iminoglycinuria and hyperglycinuria are discrete human phenotypes resulting from complex mutations in proline and glycine transporters. J Clin Invest 2008; 118(12)3881–3892
- Bröer S. Xenopus laevis Oocytes. Methods Mol Biol 2003; 227: 245–258
- Stegen C, Matskevich I, Wagner CA, Paulmichl M, Lang F, Bröer S. Swelling-induced taurine release without chloride channel activity in Xenopus laevis oocytes expressing anion channels and transporters. Biochim Biophys Acta 2000; 1467(1)91–100
- Van Winkle LJ. Biomembrane transport. Academic Press, San Diego 1999
- Attwell D, Barbour B, Szatkowski M. Nonvesicular release of neurotransmitter. Neuron 1993; 11(3)401–407
- Weber W. Ion currents of Xenopus laevis oocytes: state of the art. Biochim Biophys Acta 1999; 1421(2)213–233
- Chubb S, Kingsland AL, Bröer A, Bröer S. Mutation of the 4F2 heavy-chain carboxy terminus causes y+ LAT2 light-chain dysfunction. Mol Membr Biol 2006; 23(3)255–267
- Zhang Y. Template-based modeling and free modeling by I-TASSER in CASP7. Proteins 2007; 69(Suppl. 8)108–117
- Vriend, G. 1990. WHAT IF: a molecular modeling and drug design program. J Mol Graph, 8(1)52–6, 29.
- Phillips JC, Braun R, Wang W, Gumbart J, Tajkhorshid E, Villa E, Chipot C, Skeel RD, Kale L, Schulten K. Scalable molecular dynamics with NAMD. J Comput Chem 2005; 26(16)1781–1802
- Feller SE, Gawrisch K, MacKerell AD, Jr. Polyunsaturated fatty acids in lipid bilayers: intrinsic and environmental contributions to their unique physical properties. J Am Chem Soc 2002; 124(2)318–326
- Mackerell AD, Jr. Empirical force fields for biological macromolecules: overview and issues. J Comput Chem 2004; 25(13)1584–1604
- Guvench O, MacKerell AD, Jr. Comparison of protein force fields for molecular dynamics simulations. Methods Mol Biol 2008; 443: 63–88
- Bröer S, Bröer A, Hamprecht B. Expression of Na + -independent isoleucine transport activity from rat brain in Xenopus laevis oocytes. Biochim Biophys Acta 1994; 1192(1)95–100
- Wright JK, Dornmair K, Mitaku S, Moroy T, Neuhaus JM, Seckler R, Vogel H, Weigel U, Jahnig F, Overath P. Lactose: H+ carrier of Escherichia coli: kinetic mechanism, purification, and structure. Ann NY Acad Sci 1985; 456: 326–341
- Aubrey KR, Vandenberg RJ, Clements JD. Dynamics of forward and reverse transport by the glial glycine transporter, glyt1b. Biophys J 2005; 89(3)1657–1668
- Bohmer C, Bröer A, Munzinger M, Kowalczuk S, Rasko JE, Lang F, Bröer S. Characterization of mouse amino acid transporter B0AT1 (slc6a19). Biochem J 2005; 389(Pt 3)745–751
- Camargo SM, Makrides V, Virkki LV, Forster IC, Verrey F. Steady-state kinetic characterization of the mouse B(0)AT1 sodium-dependent neutral amino acid transporter. Pflugers Arch 2005; 451(2)338–348
- Takanaga H, Mackenzie B, Peng JB, Hediger MA. Characterization of a branched-chain amino-acid transporter SBAT1 (SLC6A15) that is expressed in human brain. Biochem Biophys Res Commun 2005; 337(3)892–900
- Nelson N. The family of Na + /Cl- neurotransmitter transporters. J Neurochem 1998; 71(5)1785–1803
- Sloan JL, Mager S. Cloning and functional expression of a human Na(+) and Cl(−)-dependent neutral and cationic amino acid transporter B(0 + ). J Biol Chem 1999; 274(34)23740–23745
- Stevens BR, Wright EM. Kinetic model of the brush-border proline/sodium (IMINO) cotransporter. Ann NY Acad Sci 1985; 456: 115–117