ABSTRACT
Ionic liquids (ILs), both as pure lubricants and as lubricant additives, have been demonstrated extensively to exhibit excellent tribological performance in terms of friction and wear reduction in the boundary lubrication (BL) regime. Because engineering contacts experience boundary and mixed as well as full film lubrication depending on operating conditions, it is crucial to examine whether lubrication regimes other the BL regime can also benefit from the use of ILs. The objective of this work is to investigate the tribological performance of IL additives in the mixed lubrication (ML) and the elastohydrodynamic lubrication (EHL) regimes. Polyethylene glycol (PEG) was used as the base fluid. ILs were synthesized in situ by dissolving lithium bis(trifluoromethanesulfonyl) imide (LiTFSI) in PEG. Friction and film thickness measurements were employed to investigate the effectiveness of IL additives at room temperature, 60°C, and 80°C at various loads and slide–roll ratios (SRRs). The effect of IL additives on the rheological behavior of PEG was also investigated. The EHL film thickness increases with increasing IL concentration. EHL friction is, however, only mildly affected by IL additives. In the ML regime, IL additives can reduce friction and metal wear compared to pure PEG in mild conditions. It is conjectured that IL forms sacrificial layers and protects the rubbing surfaces.
Introduction
Room temperature ionic liquids (ILs) are molten salts with low melting temperatures (below 100°C; Earle and Seddon (Citation1)). They consist of bulky, asymmetric cations and anions. As a result, they are liquids at ambient conditions. The unique properties of ILs, including negligible volatility and flammability, high thermal stability, high polarity, and low melting point (Wasserceid and Welton (Citation2)), make them excellent lubricants for extreme operating conditions. Indeed, ILs were first tested as space lubricants in 2001. It was found that three imidazolium tetrafluoroborate–based ILs exhibit generally lower friction and produce less wear than conventional space lubricants (Ye, et al. (Citation3)). Since then, research on the use of IL lubricants has blossomed. Pure ILs have demonstrated excellent tribological performance compared to conventional lubricants in numerous studies. Ye, et al. (Citation3), Lu, et al. (Citation4), Mu, et al. (Citation5), and Liu, et al. (Citation6) have designed and synthesized various ILs and investigated their tribological properties as pure lubricants using steel–steel and steel–aluminum contacts under oscillatory sliding conditions. Their ILs show superior antiwear performance and load-carrying capacity compared to conventional high-temperature lubricants X-1P and perfluoropolyether (PFPE). Jiménez and Bermúdez (Citation7)–(9) studied the use of L106, L108, ClB, and imidazolium ILs with different alkyl chain lengths as lubricants of titanium–steel and steel–aluminum contacts under a wide range of temperatures with a pin-on-disk tribometer. In all cases, ILs showed lower friction and wear than mineral oils and synthetic ester propylene glycol dioleate. Qu, et al. (Citation10), (Citation11) also demonstrated that ILs have better lubricity than engine oils (15W40 and 0W-10) and polyalphaolefin base oil, with a reduction in friction and wear of steel–aluminum and steel–cast iron contacts.
Though pure ILs are excellent lubricants, they are relatively expensive. They can also be corrosive (Hosseini, et al. (Citation12)), and their synthesis is often complex. As such, recent work on ILs has focused on their effectiveness as lubricant additives. ILs were firstly studied as additives in water for lubricating silicon nitride ceramics. Using a pin-on-disc reciprocating tribometer, it was shown that adding 2-methyl-[C1C2im] BF4/PF6 into water improves the load-carrying capacity and lowers the friction coefficient (Phillips and Zabinski (Citation13)). Cai, et al. (Citation14), (Citation15)) showed that the addition of 1 wt% of IL in polyethylene glycol (PEG) could effectively reduce friction and wear of sliding pairs. Similar positive effects were also present when ILs were added into polyalphaolefin (Yu, et al. (Citation16); Han, et al. (Citation17)), engine oil (Pejaković, et al. (Citation18); Jiménez, et al. (Citation19)), gear oil (Fernandes, et al. (Citation20)), safflower oil (Khemchandani, et al. (Citation21)), and glycerol (Pejaković, et al. (Citation22)).
Most work on IL lubricants have focused on the boundary lubrication (BL) regime (Ye, et al. (Citation3); Lu, et al. (Citation4); Mu, et al. (Citation5); Liu, et al. (Citation6); Jiménez and Bermúdez (Citation7)–(9); Qu, et al. (Citation10), (Citation11); Hosseini, et al. (Citation12); Phillips and Zabinski (Citation13); Cai, et al. (Citation14), (Citation15); Yu, et al. (Citation16); Han, et al. (Citation17); Pejaković, et al. (Citation18), (Citation22); Jiménez, et al. (Citation19); Fernandes, et al. (Citation20); Khemchandani, et al. (Citation21)). Many engineering components, including modern engines, however, have been designed to minimize the contribution of the BL regime. Yet, few studies have investigated the friction behavior of ILs as pure lubricant or lubricant additives in mixed or full-film lubrication (hydrodynamic lubrication, HL) regimes (Mordukhovich, et al. (Citation23); Xiao, et al. (Citation24); Nomura, et al. (Citation25); Gananath, et al. (Citation26)). Note that the lubrication mechanisms of IL additives are likely to depend on operating conditions. As such, the performance of IL additives in mixed lubrication (ML) and elastohydrodynamic lubrication (EHL) regimes may differ from their performance in the BL regime. This is because in the BL regime, surface interactions dominate the friction properties of the contact. In ML and EHL regimes, however, shearing of the lubricant film is the main contributor to frictional losses (Tung and Michael (Citation27)). If IL additives are to be used in ML and EHL regimes, studies will need to be conducted in these regimes, which is the focus of this work.
In recent years, widespread attention has been devoted to binary ILs based on lithium bis(trifluoromethanesulfonyl) imide (LiTFSI) because of their applications in electrochemical devices, such as electric double-layer capacitors, fuel cells, dye-sensitized solar cells, and lithium ion batteries (Hu, et al. (Citation28); Chen, et al. (Citation29)). Song, et al. (Citation30), (Citation31) reported that IL additives can be formed in situ in PEG by mixing small molecules of 2-oxazolidinone, triglyme, or tetraglyme with bis(trifluoromethanesulfonyl). This novel IL preparation method is simple and clean. It also improves solubility of ILs in base fluids. Lubricants produced with this novel in situ method can effectively decrease friction and wear in the boundary lubrication regime. In this work, binary ILs based on LiTFSI were prepared in PEG using an in situ synthesis method, similar to the process in Song, et al. (Citation30). The effectiveness of IL additives in the ML and EHL regimes is investigated and IL additive lubrication mechanisms in different lubrication conditions are discussed.
Materials and methods
In situ synthesis of ionic liquids
In this study, polyethylene glycol PEG-400 (PEG), with average molecular weight of 400 g mol−1, was used as the base fluid. Metal salt LiTFSI was purchased and used as received. Their molecular structures are shown in . IL additives are synthesized in situ based on the formation of complexes between lithium salts and organic compounds with an acylamino group when they are mixed at a specific molar ratio (Yoshida, et al. (Citation32)), giving rise to complexes with physicochemical properties similar to those of conventional ILs. Based on this principle, an LiTFSI-based IL, [Li(glyme)]TFSI, was prepared as an in situ coordinating lubricant additive or in situ ionic liquid additive by dissolving LITFSI in PEG (see ).
PEG-based lubricants with 0, 1, 3, and 5 wt% of LiTFSI were used in this study. They were denoted as PEG-0, PEG-1, PEG-3, and PEG-5, respectively. These model lubricants were prepared by adding the right amount of LiTFSI into PEG at room temperature. The mixtures were mixed with a magnetic stirrer until homogeneous solutions were formed (about 24 h). They were tested within 72 h after their preparations. Note that solutions with up to 20 wt% IL were prepared and all solutions were clear and transparent and remained so for at least 4 weeks, suggesting that the test solutions were stable for at least 4 weeks. Fourier transform infrared spectra confirmed that the association of Li ions and PEG occurred when LiTFSI was added into PEG, suggesting that IL additives formed in situ.
Refractive indices of model lubricants were measured by an Abbe refractometer at room condition and were 1.46 for all lubricants. Dynamic viscosities (see ) and densities of lubricants (see b) were obtained with a viscometer at room temperature, 60°C, and 80°C. Bulk rheology of model lubricants at room temperature, 60°C, and 80°C was characterized using a rheometer with a standard concentric cylinder geometry in flow sweep mode. Viscosity and shear stress were evaluated using a stepped ramp of shear rates from 0.01 to 10,000 s−1. All lubricants are Newtonian and their bulk viscosities remain constant within the range of shear rates tested. As shown in , the viscosities of all four lubricants decrease with temperature. The viscosity of PEG at room temperature increases with increasing concentration of LiTFSI. The effect of IL additives on viscosities of lubricants is, however, very small at elevated temperatures. The properties of the model lubricants are summarized in .
Friction and film thickness measurements
A mini-traction machine was employed to conduct friction tests. The detailed method is described elsewhere (Fujita and Spikes (Citation33)). Briefly, lubricants are entrained into a ball-on-disc rolling–sliding contact. Both the ball and disc were made of AISI 52100 steel. They were purchased from PCS and cleaned with isopropanol before use. The ball had a nominal diameter of 19.05 mm, surface hardness of 64 Rc, and maximum arithmetic average roughness (Ra) of 25 nm. The disc was 46 mm in diameter with a hardness of 62.5 Rc and Ra of 10 nm (given by the supplier). Note that a circular wear track with a diameter of 42 mm was formed during the friction test. Mechanical properties of the balls and discs are provided in . Friction measurements were used to obtain Stribeck curves for all model lubricants. The tests were conducted at loads of 15 and 20 N (average Hertzian pressure of 0.31 and 0.41 GPa, respectively) and slide-to-roll ratios (SRRs) of 5, 15, and 50%, at room temperature, 60°C, and 80°C. All tests started at an entrainment velocity () of 3.5 m/s, which gradually decreased to 0.007 m/s. This covers the range from the hydrodynamic to the boundary lubrication regimes. The dwell time at each speed was 6 s. Each set of rubbing surfaces, lubricated by a particular lubricant, experienced all of the above-described conditions. At least three tests were conducted for each lubricant. After the test, the discs were lightly rinsed with isopropanol to remove remaining lubricants. The wear tracks on these discs were then examined with a white light vertical scanning interferometer. The arithmetic average surface roughness (Ra) was measured at three locations on each wear track.
Table 1. Mechanical properties of the rubbing surfaces.
The lubricant film thickness under various test conditions was monitored using white light interferometry (Kaneta and Nishikawa (Citation34)) with an EHL ball-on-disc rig. Details of the test method can be found in Johnston, et al. (Citation35)). A steel ball (the same type as the one used for friction measurements) was loaded against a flat glass disc (diameter 100 mm). The disc was coated with approximately 20 nm of chromium and then 500 nm of silica (root mean square surface roughness 2 nm) to increase its reflectivity. During tests, white light was shined onto the contact. The interference fringes formed by the light reflected from the chromium layer of the disc and the surface of the steel ball were captured via a CCD camera. Lubricant film thickness was extracted from these fringe patterns. Tests were conducted at loads of 15 and 20 N, under SRR = 0 (pure rolling), 15 and 50%. Tests started at 2.5 m/s, which gradually decreased to 0.1 m/s by the end of the test at room temperature, from 3 to 1 m/s at 60°C, and from 3 to 2 m/s at 80°C. The range of entrainment speed was chosen to ensure that the tribological contacts were in the elastohydrodynamic lubrication regime. It increased with temperature as the viscosity of lubricant was reduced. Each test condition lasted 7 s. Each measurement was carried out on a fresh disk track and a fresh stainless steel ball. In all cases, the EHL regime was achieved with a film thickness below 1 μm.
Results and discussion
Stribeck curves are used to identify conditions at which a lubricated contact experiences the full-film hydrodynamic to the mixed and then to the boundary lubrication regimes as a function of load and velocity (). At a fixed load, low corresponds to the BL regime and generally gives high and constant friction due to insufficient lubricant film buildup. Increasing
results in a reduction in the friction coefficient μ (the ML regime). μ reaches a minimum when a full fluid film is formed (EHL regime). μ then increases with
as the lubricant film thickness increases (hydrodynamic lubrication) due to the viscous loss.
Figure 3. Effect of temperature on Stribeck curves for PEG-0 (neat PEG, solid symbols) and PEG-3 (PEG with 3 wt% IL additives, open symbols) at an applied load of 15 N and SRR = 5%.
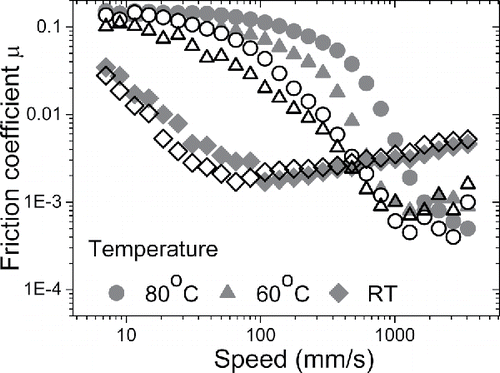
Stribeck curves obtained for PEG (PEG-0, solid symbols) and PEG with 3 wt% IL (PEG-3, open symbols) are presented in at a load of 15 N and SRR = 5% for room temperature, 60°C, and 80°C, respectively. An increase in temperature shifts the Stribeck curve to the right, with higher necessary to achieve full-film lubrication. A greater temperature effect is observed when the test temperature is raised from room temperature (diamonds, ) to 60°C (triangles, ). With PEG-0 having a larger shift than PEG-3, increasing the temperature further to 80°C (circles, ) has only a small and negligible effect. This is consistent with the results in , which show that bulk viscosities of our model lubricants are reduced substantially when the temperature is increased from room temperature (stars) to 60°C (circles), after which the change in viscosity is relatively small (squares). Note that the reduction in viscosity with temperature reduces the friction losses in the full-film lubrication regime and hence decreases the minimum friction coefficient obtained within the range of entrainment speeds tested ().
For all lubricants, increasing SRR shifts the Stribeck curves up except at the boundary lubrication regime, where maximum friction coefficient remains unaffected (see ). This is expected because increasing SRR—that is, increasing sliding speed—gives rise to greater viscous dissipation. Increasing load has a minimal effect (not shown) because lubricant film thickness is not strongly affected by the applied load (not shown).
The remainder of the article is divided into two parts. The performance of IL additives in the EHL regime is first explored, followed by a description of the effectiveness of the IL additives in the ML regime.
Effectiveness of IL additives in the EHL regime
Effect on friction
EHL friction (and HL friction) is most strongly affected by the applied SRR (see ). As discussed, increasing SRR increases friction in the EHL regime due to increased viscous dissipation. Keeping the SRR constant, the use of the IL additives in PEG shifts the Stribeck curve slightly to the left at room temperature (). As such, the tribosystem transitions from a mixed lubrication to a full-film lubrication regime at slightly lower entrainment speed with increasing IL concentration. The friction coefficient of the system is reduced marginally in the ML regime, and the minimum friction coefficient and friction coefficients for full-film lubrication increase very slightly as IL is added. This is due to an increase in lubricant viscosity and thus lubricant film thickness. The critical entrainment speed
for pure PEG where
is achieved is about 100 mm/s (solid vertical lines) at room temperature. The amount of shift of the Stribeck curve very weakly depends on the concentration of IL additives in PEG and the applied SRR. At room temperature and SRR = 50%, an addition of 5 wt% IL (PEG-5) gives rise to the most significant change in
where it drops from 100 mm/s to about 40 mm/s (dash vertical line, ). Note that changing the applied load does not change the effect of IL additives in room temperature. This may be due to the limited range of loads tested in this work.
Figure 5. Effect of IL additive concentration on Stribeck curves of PEG and PEG with IL additives. The solid and dashed vertical lines approximate UEHL for PEG-0 and PEG-5, respectively.
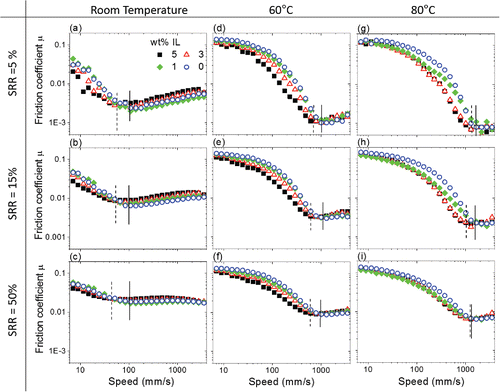
At 60 and 80°C, is relatively constant regardless of IL concentration, applied load, and SRR (see –5i). The use of IL additives again shifts
slightly to the left although its effect diminishes with increasing temperature. This is consistent with results in , which show that the bulk viscosity of PEG is more sensitive to temperature than that due to the addition of IL. In fact, the higher the temperature, the smaller the effect of IL concentration on bulk viscosity. For the same reason, though the range of entrainment speed used at higher temperature in this study is insufficient to investigate the performance of these lubricants in the HL regime, it is unlikely that IL concentration would have a strong effect in the HL regime.
Effect on film thickness
Film thickness measurements have been conducted to study the influence of IL additives in the EHL regime. The tribological contacts observed with interferometry show a classical horseshoe geometry (not shown), confirming that the EHL regime was achieved in these conditions. presents measured central film thickness plotted against the entrainment speed
in a log-log scale for the four test lubricants. All test lubricants show an approximately linear relationship between
and
for the range of
tested. The film thickness obtained for PEG-0 at 20 N and pure rolling condition (SRR = 0) is about 70 nm at 0.1 m/s and increases to 540 nm at 2.5 m/s at room temperature (). When IL additives are used, thicker films are observed. Film thickness increases with the concentration of IL additives in PEG, which agrees with the viscosity measurements of the mixture (). The effect of the IL additives on the film thickness is similar for all test temperatures, with increasing temperature reducing lubricant film thickness due to a decrease in lubricant viscosity (b and ). The lubricant film thickness in a contact is not affected by the applied SRR (not shown). At an applied load of 20 N, the applied shear rate based on measured film thickness vary between
and
and
and
at SRR = 15 and 50%, respectively. The negligible effect of SRR on measured film thickness suggests that shear heating and shear thinning at the applied shear rate range are negligible in our test conditions. Surface temperature and lubricant temperatures can be predicted based on models developed in Jaeger (Citation36) and Hirst and Moore (Citation37) and are 0.62 and 0.59°C, respectively, in the case of SRR = 50% and
= 2.5 m/s. These estimates support that shear heating is not significant.
Figure 6. Comparisons between measured (symbols) and predicted (lines) film thickness at an applied load of 20 N and SRR = 0. Prediction is performed using the pressure–viscosity coefficient (α) of PEG-0.
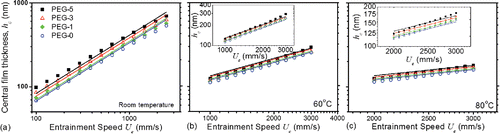
The measured central film thickness (symbols, ) is compared with the predicted central film thickness
(lines, ) based on the Hamrock-Dowson formula for an EHL contact (Hamrock and Dowson (Citation38)) as shown in Eq. [1]:
[1] where
is the radius of the ball,
is the viscosity of the lubricant at the inlet of the contact (see ),
is the reduced modulus (see ),
is the applied load, and
is the pressure–viscosity coefficient. For a given test condition and geometry, the estimation of
only depends on
and
.
measured at corresponding speed
, on the other hand, can be used to estimate
using Eq. [1]. This is done by fitting
with Eq. [1] with
being the only fitting parameter. For PEG-0, estimated
depends on temperature and is about 6.6, 8.9, and 7.9
at room temperature, 60°C, and 80°C, respectively (see ). Using the
values for PEG-0, the measured and predicted film thickness values for PEG with the IL additives only agree in some cases (). This is because the IL additives have changed the values of
of the lubricants and its effect is temperature dependent. At room temperature,
of our model lubricants increases with IL concentration (stars, ). At elevated temperature (circles and squares in for 60 and 80°C, respectively), adding IL decreases
. The drop is, however, small. In addition, the use of IL additive reduces the effect of temperature on
. Because the pressure–viscosity coefficient of a fluid is highly dependent on the molecular structure of the oil (Larsson, et al. (Citation39); Aderin, et al. (Citation40)), the use of additives to alter viscosities and possibly the pressure–viscosity coefficient of lubricants is common. For ionic liquids, it has been shown that in general they have lower pressure–viscosity coefficients than hydrocarbon-based lubricants (Pensado, et al. (Citation41)). It is then reasonable to expect the pressure–viscosity coefficient to drop with the use of IL additives. Indeed, at a fixed concentration of IL in PEG,
reduces when the temperature increases from 60 to 80°C. This is consistent with Pensado, et al. (Citation42), who found that
of pure IL decreases with temperature.
Table 2. Parameters used to predict the lubricant film thickness based on Hamrock-Dowson formula.
Effectiveness of IL additives in the ML regime
Friction reduction
The benefit of IL additives at 60 and 80°C is observed in the ML regime (see ). At 60°C, IL additives can effectively reduce friction in the ML regime, especially with low SRR. At SRR = 5%, adding 1 wt% IL additives (diamonds) results in some friction reduction (), and a further increase in the IL concentration (squares, ) substantially reduces . A similar effect of IL concentration on
is observed at higher SRR and higher load, although the magnitude is less.
The effect of IL additives on at 80°C is qualitatively similar to that observed at 60°C although the effect is weaker at higher SRR. At all SRRs, the friction coefficient decreases when 1 wt% of IL additives is used but only a marginal effect is observed for further increases in IL concentrations.
Wear reduction
Wear tracks on the steel discs lubricated by each of the four model lubricants were observed to investigate the effect of the IL additive concentration on steel wear. presents images and cross-section profiles of the wear tracks. There was no significant material removal at the wear tracks. Though the surface roughness of all worn surfaces (see Ra in ) is higher than that of the pristine steel discs (e, Ra = 8.1 nm), the higher the IL concentration of the lubricant, the smoother the wear tracks. Indeed, the roughness of the worn surface lubricated by PEG-5 (, Ra = 11.4 nm) is very close to that of the pristine disc. This confirms that IL additives are effective in reducing steel wear.
Discussion
Bulk viscosity enhancement with IL additives
When IL is added to PEG, the viscosity of the lubricant increases because pure IL is more viscous than PEG. The increase in viscosity due to IL addition would depend on the interactions between IL additives and the base oil (Davoodnia, et al. (Citation43)), IL molecular structures (Nazari, et al. (Citation44)), and ion sizes (Greaves, et al. (Citation45)). For a single-phase fluid composed of multiple liquid components, the viscosity of the blend is a function of the individual viscosities of each component and the blend ratio, as defined by the Refutas equation (Maples (Citation46)). Yu, et al. (Citation16) found that the measured viscosities of blends of phosphonium-based ionic liquids in both polyalphaolefin base oils and engine oil agree well with the Refutas equation. In our study, the bulk viscosities of our PEG-IL blends, as shown in , are also consistent with the Refutas equation. This suggests that once our IL additives are formed in PEG, there are no other specific interactions between the LiTFSI and PEG in ambient conditions other than the formation of in situ IL additives.
The measured EHL central film thickness lubricated by PEG with IL additives is higher than the predictions based on the Hamrock-Dowson equation using the measured bulk viscosities and the pressure–viscosity coefficient of PEG-0, especially when the IL concentration is high. Our results show that IL additives changed the pressure–viscosity coefficient
of PEG. The effect of IL additives on the pressure–viscosity coefficient is temperature dependent, with
increasing substantially with IL concentration at room temperature, whereas
decreases slightly with IL addition at 60 and 80°C. Therefore, the effectiveness of ILs as viscosity modifiers and their effects on lubricant film thickness and friction in the EHL regime are temperature dependent. In addition, note that the local IL arrangement near interfaces (see next section) can increase the local viscosity near the surfaces compared to that of the bulk lubricant and contributes to enhanced film thickness.
The reduction in pressure–viscosity coefficients due to the addition of IL into PEG at elevation temperate could limit the increase in viscous friction loss at high pressure and reduce subsurface stresses and pressure spikes that play an important role in wear and failure of gear elements and bearing.
Weak effect of IL additives on EHL friction
ILs are known to participate in a variety of attractive interactions involving both weak, nonspecific isotropic forces (e.g., van der Waals, Parsegian (Citation47); dispersion forces, Mahanty and Ninham (Citation48)) and strong (Coulombic), specific anisotropic forces (e.g., hydrogen bonding, Jeffrey (Citation49); electron pair donor/acceptor interactions). This allows ILs to adsorb on surfaces and locally rearrange themselves, as shown by atomic force microscopy studies (Segura, et al. (Citation50)). The nature of the adsorbed layer on the metal substrate will influence the effectiveness of a particular IL as lubricants/lubricant additives. The IL adsorbed layer formed on gold surface in 0.1 wt% 1-hexyl-3-methyl-imidazolium bis(trifluoromethanesulfonyl)imide (HMIM NTf2) in ethanol has been determined by ellipsometry to have an average layer thickness of 0.15 nm, which was smaller than the size of an IL ion pair, due to poor affinity of IL to the gold surface (Beattie, et al. (Citation51)). Such small layer thickness suggests that the IL layer is likely be to a submonolayer. Barnhill, et al. (Citation52), on the other hand, suggested that when a metal surface is lubricated by an IL, a layer-structured IL film may form by physical adsorption, with anions tending to adsorb onto the naturally positively charged metal surface to form a monolayer. Cations then form a second layer due to Coulombic forces and, sometimes, weak hydrogen bonds. Note that IL consists of bulky, asymmetric ions. Unlike commonly used surfactants, adsorbed IL layers preserve their fluidity (Cheng, et al. (Citation53)). These local arrangements also alter the distribution of solvent molecules at a solid–IL interface. The adsorbed ILs and the near-surface self-assembled IL structures determine friction at solid–lubricant interfaces (Smith, et al. (Citation54), (Citation55)). Hence, multilayer films may build up that create low-traction interfaces (slip) under sliding, which may help reduce friction. Indeed, surface force apparatus experiments have shown that IL ions form layers on mica surfaces, with each layer having a different friction coefficient and the shear plane can form at the alkyl domain (monolayer arrangement) or ionic domain (bilayer arrangement; Werzer, et al. (Citation56)). IL arrangements on surfaces have also been confirmed recently with molecular dynamic simulations (Wells and Southcombe (Citation57); Grew and Cameron (Citation58)).
We conjecture that when PEG with IL additives is used, adsorbed IL layers are formed on rubbing surfaces. Overall, the effect of IL additives on friction reduction in the EHL regime studied in this work is relatively weak. Note that in the EHL regime, the two rubbing surfaces are separated by the lubricant film. Without any surface contact, it is difficult for the adsorbed layers to be transformed into tribofilms. Thus, it is likely that the structure of this adsorbed film is similar to those observed in surface force apparatus studies (Perkin, et al. (Citation59)). The effect of the adsorbed film has been shown to be short-ranged and repulsive (Hayes, et al. (Citation60)). Hence, the repulsion forces can only be experienced by rubbing surfaces when they are close to each other. Because lubricant rheology rather than surface effects dominate in the EHL regime, it is not surprising that the effect of IL addition on the lubricity of PEG is related to the ability of IL to increase the viscosity of PEG. An increase in lubricant film thickness as IL is added is observed. The weak dependence of on SRR is expected as film thickness remains constant with SRR in our test conditions.
IL additive effects on the ML regime
It is generally accepted that the effect of IL chemistry on their lubrication performance in the BL and ML regimes can be ascribed to the generation of physically adsorbed layers (see previous section) and complex chemical reactions of anions with fresh surfaces (Zhou, et al. (Citation61)). The adsorbed layers can provide slip planes that have much lower shear strength than bare metal–on–bare metal (or metal oxide–on–metal oxide) contacts (Zhou, et al. (Citation61); see also previous section). IL can be very reactive under the right conditions, such as high temperature and high shear stress encountered in a tribological contact. When the two contact surfaces rub against each other, anions of IL may react with metal to form tribofilms. The formation of a tribofilm is crucial to the protection of rubbing surfaces (Qu, et al. (Citation10), (Citation11); Cai, et al. (Citation14); Han, et al. (Citation17); Pejaković, et al. (Citation18)). The nature of the film may be affected by the applied SRR. Arora and Cann (Citation62) suggested that at low SRRs, an adsorbed double-layer mechanism predominates and under some conditions this is augmented by a thicker physically deposited film. For high sliding contacts where damage to the surface occurs, a chemical film is formed. The thickness of the tribofilms ranges from 10 to 200 nm and depends on IL chemistry and test conditions (Qu, et al. (Citation11), (Citation63)). Though the IL tribofilm may be very thin, it can still be sufficient to effectively protect rubbing surfaces from wear.
In this study, the amount of steel wear is reduced by the use of IL additives. Yet the friction coefficients at low entrainment speeds (the BL regime) remain constant. Both the topography of the wear tracks () and surface chemical analysis with energy-dispersive X-ray spectroscopy (not shown) shows no conclusive evidence of an IL tribofilm. This suggests that the reduction in surface wear is likely due to the IL surface films acting as sacrificial layers. As the surface film is worn during rubbing, new IL layers are formed on the rubbing surfaces quickly and the process repeats. It would be insightful to have information about the properties of this surface sacrificial layer. Indeed, Landauer, et al. (Citation64) used nanoindentation tests to correlate the relationship between surface film properties and its antiwear performance. This was, however, not pursued in this study because our surface film is likely to be very thin.
Though an IL surface film generally reduces wear, it can desorb or melt at some specific situations. Such situations include high temperature, which means that their friction-reducing properties are lost above a certain critical situation and often depend on the concentration of IL additives present and the chemistry of the IL (Gabler, et al. (Citation65); Sharma, et al. (Citation66)). This may explain why the IL additives are not as effective in improving the lubrication of PEG at 80°C and high SRR.
Note that in the mixed lubrication regime, a reduction in surface wear is observed when IL additives are used. The effectiveness is enhanced with increasing IL concentration in PEG (). In the ML regime, actual solid–solid contacts occur only at a fraction of the nominal contact, while the rest of the rubbing surfaces are separated by lubricants. Thus, the ability to generate a lubricant film, which is governed by the viscosity of the lubricant, will play an important role. This might also explain why temperature has a significant effect on the effectiveness of IL additives in the mixed lubrication regime (see –5i) as lubricant viscosity is sharply reduced with temperature. At 80°C, the benefit of IL additives is only observed with 1 wt% addition, and a further increase in IL concentration has a marginal effect. Similarly, IL additives have a stronger effect under low SRR conditions in the ML regime. Because a surface-adsorbed double-layer mechanism predominates at low SRR ratios as suggested by Cases, et al. (Citation67), the lower applied shear stress with low SRR may allow the double layer to remain on the surface and prevent metal–metal contacts more effectively. The performance of the IL additive also has a very slight load dependence, with IL additives performing better at low loads. At low load, there might be fewer asperity contacts; hence, the contact can benefit more readily from the increased lubricant viscosity due to the use of IL additives.
Conclusion
In this work, we explored the effectiveness of ionic liquids as lubricant additives for ML and EHL regimes. It is demonstrated that LiTFSI as a lubricant additive in PEG can reduce friction and wear in the mixed lubrication regime and increase the lubricant film thickness in the EHL regime. The effect of IL additives on the tribological performance of PEG depends on a few of parameters, such as temperature, SRR, and IL concentration. Experimental results suggest that high temperature reduces the effectiveness of IL additives. This is because an increase in test temperature substantially reduces lubricant viscosity. Thus, at lower temperature, higher lubricant film thickness is achieved in the EHL regime. The increase in viscosity due to the addition of LiTFSI into PEG obeys the Refutas equation. Yet the measured film thickness of PEG with IL additives in the EHL regime does not match the predictions based on the Hamrock-Dowson equation using the pressure–viscosity coefficient of PEG. This is because the viscosity–pressure coefficient of the lubricant depends on IL concentration and temperature.
In the mixed lubrication regime, increasing IL concentration reduces the friction and wear and is likely due to the formation of an adsorbed layer/tribofilm. In addition, it is found that IL additives work better at lower SRR. Load has little effect on the performance of IL additives. In the mixed lubrication regime, the increased viscosity may allow the physically adsorbed layers and tribofilm to generate more easily and maintain integrity on the rubbing surface, thus improving the tribological performance of the lubricant. The fact that IL additives are less effective at 80°C and high SRR compared to 60°C and low SRR suggests that the adsorbed layer/tribofilm is mechanically weak and is working as a sacrificial layer.
Graphic Abstract
Download ()Acknowledgments
The authors gratefully acknowledge Dr. Jie Zhang, Jon Dench, and the Tribology Group of Imperial College London for fruitful discussion and technical support.
Additional information
Funding
References
- Earle, M. J. and Seddon, K. R. (2000), “Ionic Liquid–Green Solvents for the Future: Pure and Apply Chemistry,” Tribology Transactions, 72, pp 1391–1398.
- Wassercheid, P. and Welton, T. (2008), Ionic Liquids in Synthesis, Wiley: Weinheim, Germany.
- Ye, C., Liu, W., Chen, Y., and Yu, L. (2001), “Room-Temperature Ionic Liquids: A Novel Versatile Lubricant,” Chemical Communications, 21, pp 2244–2245.
- Lu, Q., Wang, H., Ye, C., Liu, W., and Xue, Q. (2004), “Room Temperature Ionic Liquid 1-Ethyl-3-hexylimidazolium-bis (Trifluoromethylsulfonyl)-imide as Lubricant for Steel–Steel Contact,” Tribology International, 37, pp 547–552.
- Mu, Z., Zhou, F., Zhang, S., Liang, Y., and Liu, W. (2005), “Effect of the Functional Groups in Ionic Liquid Molecules on the Friction and Wear Behavior of Aluminum Alloy in Lubricated Aluminum-on-Steel Contact,” Tribology International, 38, pp 725–731.
- Liu, X., Zhou, F., Liang, Y., and Liu, W. (2006), “Tribological Performance of Phosphonium Based Ionic Liquids for an Aluminum-on-Steel System and Opinions on Lubrication Mechanism,” Wear, 261, pp 1174–1179.
- Jiménez, A. E. and Bermúdez, M. D. (2007), “Ionic Liquids as Lubricants for Steel–Aluminum Contacts at Low and Elevated Temperatures,” Tribology Letters, 26, pp 53–60.
- Jiménez, A. E. and Bermúdez, M. D. (2009), “Ionic Liquids as Lubricants of Titanium–Steel Contact,” Tribology Letters, 33, pp 111–126.
- Jiménez, A. E. and Bermúdez, M. D. (2010), “Ionic Liquids as Lubricants of Titanium–Steel Contact—Part 3: Ti6Al4V Lubricated with Imidazolium Ionic Liquids with Different Alkyl Chain Lengths,” Tribology Letters, 40, pp 237–246.
- Qu, J., Blau, P. J., Dai, S., Meyer, H. M., III, and Truhan, J. J. (2009), “Tribological Characteristics of Aluminum Alloys Sliding against Steel Lubricated by Ammonium and Imidazolium Ionic Liquids,” Wear, 267, pp 1226–1231.
- Qu, J., Blau, P. J., Dai, S., Luo, H., and Meyer, H. M., III (2009), “Ionic Liquids as Novel Lubricants and Additives for Diesel Engine Applications,” Tribology Letters, 35, pp 181–189.
- Hosseini, M. G., Arshadi, M. R., Shahrabi, T., and Ghorbani, M. (2003), “Synergistic Influence of Benzoate Ions on Inhibition of Corrosion of Mild Steel in 0.5m Sulphuric Acid by Benzotriazole,” IJE Transactions B: Applications, 16(3), pp 255–264.
- Phillips, B. S. and Zabinski, J.S. (2004), “Ionic Liquid Lubrication Effects on Ceramics in a Water Environment,” Tribology Letters, 17(3), pp 533–541.
- Cai, M., Liang, Y., Yao, M., Zhou, F., and Liu, W. (2010), “Imidazolium Ionic Liquids as Antiwear and Antioxidant Additive in Poly(ethylene glycol) for Steel/Steel Contacts,” ACS Applied Materials and Interfaces, 2, pp 870–876.
- Cai, M., Liang, Y., Zhou, F., and Liu, W. (2013), “A Novel Imidazolium Salt with Antioxidation and Anticorrosion Dual Functionalities as the Additive in Poly(ethylene glycol) for Steel/Steel Contacts,” Wear, 306, pp 197–208.
- Yu, B., Bansal, D. G., Qu, J., Sun, X., Dai, S., Blau, P. J., Bunting, B. G., Mordukhovich, G., and Smolenski, D. J. (2012), “Oil-Miscible and Non-Corrosive Phosphonium-Based Ionic Liquids as Candidate Lubricant Additives,” Wear, 289, pp 58–64.
- Han, Y., Qiao, D., Zhang, L., and Feng, D. (2015), “Study of Tribological Performance and Mechanism of Phosphonate Ionic Liquids for Steel/Aluminum Contact,” Tribology International, 84, pp 71–80.
- Pejaković, V., Kronberger, M., Mahrova, M., Tojo, E., and Kalin, M. (2016), “Pyrrolidinium Sulfate and Ammonium Sulfate Ionic Liquids as Lubricant Additives for Steel/Steel Contact Lubrication,” Proceedings of the Institution of Mechanical Engineers – Part J: Journal of Engineering Tribology, 226, pp 923–932.
- Jiménez, A. E., Bermúdez, M. D., Carrión, F. J., and Martínez-Nicolás, G. M. (2006), “Room Temperature Ionic Liquids as Lubricant Additives in Steel–Aluminium Contacts: Influence of Sliding Velocity, Normal Load and Temperature,” Wear, 261, pp 347–359.
- Fernandes, C. M. C. G., Battez, A. Z., González, R., Monge, R., Viesca, J. L., García, A., Martins, R. C., and Seabra, J. H. O. (2015), “Torque Loss and Wear of FZG Gears Lubricated with Wind Turbine Gear Oils Using an Ionic Liquid as Additive,” Tribology International, 90, pp 306–314.
- Khemchandani, B., Somers, A., Howlett, P., Jaiswal, A. K., Sayanna, E., and Forsyth, M. (2014), “A Biocompatible Ionic Liquid as an Antiwear Additive for Biodegradable Lubricants,” Tribology International, 77, pp 171–177.
- Pejaković, V., Tomastik, C., and https://www.sciencedirect.com/science/article/pii/S0301679X16000463 – !Kalin, N. D. (2016), “Influence of Concentration and Anion Alkyl Chain Length on Tribological Properties of Imidazolium Sulfate Ionic Liquids as Additives to Glycerol in Steel–Steel Contact Lubrication,” Tribology International, 97, pp 234–243.
- Mordukhovich, G., Qu, J., Howe, J. Y., Bair, S., Yu, B., Luo, H., Smolenski, D. J., Blau, P. J., Bunting, B. G., and Dai, S. (2013), “A Low-Viscosity Ionic Liquid Demonstrating Superior Lubricating Performance from Mixed to Boundary Lubrication,” Wear, 301, pp 740–746.
- Xiao, H., Guo, D., Liu, S., Pan, G., and Lu, X. (2011), “Film Thickness of Ionic Liquids under High Contact Pressures as a Function of Alkyl Chain Length,” Tribology Letters, 41, pp 471–477.
- Nomura, A., Okayasu, K., Ohno, K., Fukuda, T., and Tsujii, Y. (2012), “Lubrication Mechanism of Concentrated Polymer Brushes in Solvents: Effect of Solvent Viscosity,” Polymer Chemistry, 3, pp 148–153.
- Gananath, D., Thakre, S. C., Sharma, S. P., and Tyagi, H. M. R. (2016), “A Theoretical Study of Ionic Liquid Lubricated μ-EHL Line Contacts Considering Surface Texture,” Tribology International, 94, pp 39–51.
- Tung, S. C. and Michael, L. M. (2004), “Automotive Tribology Overview of Current Advances and Challenges for the Future,” Tribology International, 37, pp 517–536.
- Hu, Y., Li, H., Huang, X., and Chen, L. (2004), “Novel Room Temperature Molten Salt Electrolyte Based on LiTFSI and Acetamide for Lithium Batteries,” Electrochemical Communications, 6, pp 28–32.
- Chen, R., Wu, F., Li, L., Qiu, X., and Chen, S. (2007), “The Structure–Activity Relationship Studies of Binary Room Temperature Complex Electrolytes Based on LiTFSI and Organic Compounds with Acylamino Group,” Vibrational Spectroscopy, 44, pp 297–307.
- Song, Z., Fan, M., Liang, Y., Zhou, F., and Liu, W. (2013), “Lithium-Based Ionic Liquids: In situ–Formed Lubricant Additive Only by Blending,” Tribology Letters, 49, pp 127–133.
- Song, Z., Liang, Y., Fan, M., Zhou, F., and Liu, W. (2014), “Lithium-Based Ionic Liquids Functionalized by Symtriazine and Cyclotriphosphazene as High Temperature Lubricants,” Tribology International, 70, pp 136–141.
- Yoshida, T. T., Hachida, K., and Tsuchiya, T. M. (2010), “Physicochemical Properties of Glyme–Li Salt Complexes as a New Family of Room-Temperature Ionic Liquids,” Chemical Letters, 39, pp 753–755.
- Fujita, H. and Spikes, H. A. (2004), “The Formation of Zinc Dithiophosphate Antiwear Films,” Proceedings of the Institution of Mechanical Engineers – Part J: Journal of Engineering Tribology, 218, pp 265–277.
- Kaneta, M. S. T. and Nishikawa, H. (1992), “Optical Interferometric Observations of the Effects of a Bump on Point Contact EHL,” Journal of Tribology, 114, pp 779–784.
- Johnston, G. J., Wayte, R., and Spikes, H. A. (1991), “The Measurement and Study of Very Thin Lubricant Films in Concentrated Contacts,” Tribology Transactions, 34, pp 187–194.
- Jaeger, J. C. (1942), “Moving Sources of Heat and the Temperature at Sliding Contacts,” Proceedings of the Royal Society of New South Wales, 76, pp 203–224.
- Hirst, W. and Moore, A. J. (1980), “The Effect of Temperature on Traction in Elastohydrodynamic Lubrication,” Philosophical Transactions of the Royal Society of London A, 298, pp 183–208.
- Hamrock, B. J. and Dowson, D. (1977), “Isothermal Elastohydrodynamic Lubrication of Point Contacts: Part III—Fully Flooded Results,” Journal of Lubrication Technology, 99(2), pp 264–275.
- Larsson, R., Kassfeldt, E., Byheden, A., and Norrby, T. (2001), “Base Fluid Parameters for Elastohydrodynamic Lubrication and Friction Calculations and their Influence on Lubrication Capability,” Journal of Synthetic Lubrication, 81, pp 83–198.
- Aderin, M., Johnston, G. J., and Spikes, H. A. (1992), “The Elastohydrodynamic Properties of Some Advanced Non Hydrocarbon-Based Lubricants,” Lubrication Engineering, 48, pp 633–638.
- Pensado, A. S., Comunas, M. J. P., and Fernández, J. (2008), “The Pressure–Viscosity Coefficient of Several Ionic Liquids,” Tribology Letters, 31, pp 107–118.
- Paredes, X., Fandiño, O., Pensado, A. S., Comuñas, M. J. P., and Fernández, J. (2012), “Pressure–Viscosity Coefficients for Polyalkylene Glycol Oils and Other Ester or Ionic Lubricants,” Tribology Letters, 45, pp 89–100.
- Davoodnia, A., Heravi, M. M., Safavi-Rad, Z.., and Tavakoli-Hoseini, N. (2010), “Green, One-Pot, Solvent-Free Synthesis of 1,2,4,5-Tetrasubstituted Imidazoles Using a Brønsted Acidic Ionic Liquid as Novel and Reusable Catalyst,” Synthetic Communications, 40(17), pp 2588–2597.
- Nazari, S., Ghandi, K., Cameron, S. B., and Johnson, M. B. (2013), “Physicochemical Properties of Imidazo Pyridine Protic Ionic Liquids,” Journal of Materials Chemistry A, 1(38), pp 11570–11579.
- Greaves, T. L., Weerawardena, A., Fong, C., Krodkiewsk, I. A., and Drummond, C. J. (2000), “Protic Ionic Liquids: Solvents with Tunable Phase Behavior and Physicochemical Properties,” The Journal of Physical Chemistry B, 110(45), pp 22479–22487.
- Maples, R. E. (2008), Petroleum Refinery Process Economics, 2nd ed., Pennwell Books. PennWell Corp: Minden, NV, U.S.A.
- Parsegian, V. A. (2005), Vander Waals Forces: A Handbook for Biologists, Chemists, Engineers and Physicists, Cambridge University Press: New York.
- Mahanty, J. and Ninham, B. W. (1976), Dispersion Forces, Academic Press: London.
- Jeffrey, G. A. A. (1997), Introduction to Hydrogen Bonding, Oxford University Press: New York.
- Segura, J. J., Elbourne, A., Wanless, E. J., Warr, G. G., Voïtchovsky, K., and Atkin, R. (2013), “Adsorbed and Near Surface Structure of Ionic Liquids at a Solid Interface,” Physical Chemistry, 15, pp 3320–3328.
- Beattie, D. A., Harmer-Bassell, S. L., Ho, T. T. M., Krasowska, M., Ralston, J., Sellapperumage, P. M. F., and Wasik, P. (2015), “Spectroscopic Study of Ionic Liquid Adsorption from Solution onto Gold,” Physical Chemistry Chemical Physics, 17, pp 4199–4209.
- Barnhill, W. C., Qu, J., Luo, H., Meyer, H. M., III, Ma, C., Chi, M., and Papke, B. L. (2014), “Phosphonium–Organophosphate Ionic Liquids as Lubricant Additives: Effects of Cation Structure on Physicochemical and Tribological Characteristics,” Applied Materials & Interfaces, 6, pp 22585–22593.
- Cheng, H., Dienemann, J. N., Stock, P., Merola, C., Chen, Y., and Valtiner, M. (2016), “The Effect of Water and Confinement on Self-Assembly of Imidazolium Based Ionic Liquids at Mica Interfaces,” Nature, Scientific Reports, 6, pp 30058.
- Smith, A. M., Parkes, M. A., and Perkin, S. (2014), “Molecular Friction Mechanisms across Nanofilms of a Bilayer-Forming Ionic Liquid,” Journal of Physical Chemistry Letters, 5(22), pp 4032–4037.
- Smith, A. M., Lovelock, K. R., and Gosvami, N. N. (2013), “Quantized Friction across Ionic Liquid Thin Films,” Physical Chemistry Chemical Physics, 15(37), pp 15317–15320.
- Werzer, O., Cranston, E. D., Warr, G. G., Atkin, R., and Rutland, M. W. (2012), “Ionic Liquid Nanotribology: Mica–Silica Interactions in Ethylammonium Nitrate,” Physical Chemistry Chemical Physics, 14(15), pp 5147–5152.
- Wells, H. M. and Southcombe, J. E. (1920), “The Theory and Practice of Lubrication: The ‘Germ’ Process,” Journal of the Society of Chemistry London, 39, pp 51T–60T.
- Grew, W. J. S. and Cameron, A. (1972), “Thermodynamics of Boundary Lubrication and Scuffing,” Proceedings of the Royal Society of London A, 327, pp 47–59.
- Perkin, S., Albrecht, T., and Klein, J. (2010), “Layering and Shear Properties of an Ionic Liquid, 1-Ethyl-3-methylimidazolium Ethylsulfate, Confined to Nano-Films between Mica Surfaces,” Physical Chemistry Chemical Physics, 12(6), pp 1243–1247.
- Hayes, R., Borisenko, N., Tam, M. K., Howlett, P. C., Endres, F., and Atkin, R. (2011), “Double Layer Structure of Ionic Liquids at the Au(111) Electrode Interface: An Atomic Force Microscopy Investigation,” Journal of Physical Chemistry C, 115(14), pp 6855–6863.
- Zhou, F., Liang, Y., and Liu, W. (2009), “Ionic Liquid Lubricants: Designed Chemistry for Engineering Applications,” Chemical Society Reviews, 38(9), pp 2590–2599.
- Arora, H. and Cann, P. M. (2010), “Lubricant Film Formation Properties of Alkyl Imidazolium Tetrafluoroborate and Hexafluorophosphate Ionic Liquids,” Tribology International, 43, pp 1908–1916.
- Qu, J., Luo, H., Chi, M., Ma, C., Blau, P. J., Dai, S., and Viola, M. B. (2014), “Comparison of an Oil-Miscible Ionic Liquid and ZDDP as a Lubricant Anti-Wear Additive,” Tribology International, 71, pp 88–97.
- Landauer, A. K., Barnhil, W. C., and Qu, J. (2016), “Correlating Mechanical Properties and Anti-Wear Performance of Tribofilms Formed by Ionic Liquids, ZDDP and Their Combinations,” Wear, 354–355, pp 78–82.
- Gabler, C., Dörr, N., and Allmaier, G. (2014), “Influence of Cationic Moieties on the Tribolayer Constitution Shown for Bis(trifluoro-methylsulfonyl) Imide Based Ionic Liquids Studied by X-ray Photoelectron Spectroscopy,” Tribology International, 80, pp 90–97.
- Sharma, V., Dörr, N., and Aswath, P. B. (2016), “Chemical–Mechanical Properties of Tribofilms and Their Relationship to Ionic Liquid Chemistry,” RSC Advances, 6, pp 22341–22356.
- Cases, J. M., Poirier, J. E., and Canet, D. (1985), “Adsorption l'Interface Solide-Solution Aqueuse des Tensioactifs Ioniques” (Adsorption Solid Interface-Aqueous Solution of Ionic Surfactants) Cases, J.M. (Ed.), Solid–Liquid Interactions in Porous Media, pp 335–370, Technip Paris: Paris.