ABSTRACT
Zastepa A, Watson SB, Kling H, Kotak B. 2017. Spatial and temporal patterns in microcystin toxins in Lake of the Woods surface waters. Lake Reserve Manag. 33:433–443.
Lake of the Woods (LOW) is a complex system, with limited exchange between its multiple basins and strong spatial variance in physicochemical conditions and susceptibility to cyanobacterial blooms (cHABs). Nutrient input from tributaries has contributed to a highly productive southern basin with widespread cHABs, which are also present in some northern sub-basins. To date, there is no systematic lake-wide assessment of spatial and temporal patterns of microcystins (MCs) in relation to cyanobacterial taxa along physicochemical gradients. To achieve this, surface waters were sampled June to September in 2006, 2008–2010, and 2014–2015 offshore, inshore, and along developed shorelines. Offshore MC measured by ELISA and PPIA was consistently low despite high chlorophyll-a (Chl-a) (e.g. 0.7 µg MC-LR equivalents/L; 126 µg/L Chl-a), with only two cases exceeding the 20 µg MC-LR equivalents/L World Health Organization recreational guideline. LC-MS/MS analysis of MC congeners (2014–2015) showed MC-LR dominating, with MC-LA also present. A strong positive correlation of MC with NO3 (P < 0.005) was observed but not with other nutrients, water transparency, or mixing. The toxicity of phytoplankton biomass was positively correlated with dissolved P and inversely with particulate N:P (P < 0.02, P < 0.001 respectively), suggesting lower toxicity under P-deficiency. Shorelines contained much higher MC concentrations with more than 25% above 20 µg MC-LR equivalents/L in 2006 and 2009 (maximum ∼ 600 µg MC-LR equivalents/L). Several potential producers of MCs were suspected, including Dolichospermum and Microcystis. Cyanobacteria identified in LOW are known elsewhere as producers of other toxins including neurotoxins, but these have not yet been assessed in LOW.
Annual cyanobacterial blooms (cHABs) in Lake of the Woods (LOW, Canada-USA) are generally thought to originate in the large, shallow, polymictic southern basin, which receives 75% of the lake's total phosphorus (TP) external loading via the Rainy River (Hargan et al. Citation2011). Water flows northward into a series of deeper interconnected basins and it is thought that blooms are transported with it. However, there is no direct evidence supporting this concept, while some authors suggest that blooms may also initiate in the north (Watson and Kling Citation2017). Notably, spatio-temporal differences in physicochemical conditions and limited inter-basin exchange in LOW are associated with changes in cHABs composition, severity and toxicity.
Among the toxins produced by cyanobacteria, microcystins (MCs) are the most resilient and commonly reported in north temperate waterbodies (Chorus Citation2001). These compounds are small, cyclic peptides, produced by both planktonic and benthic cyanobacteria. They are protein phosphatase inhibitors and toxic to many organisms. In humans, they primarily target liver cells, and with acute exposure can cause massive hemorrhaging and death. They have multiple other non-fatal effects with subacute exposure including flu-like symptoms, and vomiting and diarrhea; furthermore they are known carcinogens which increase the risk of cancer with chronic exposure (Uneo et al. Citation1996, Zhou et al. Citation2002). Although commonly expressed as MC-LR equivalents (summed concentration of all congeners), there are over 100 known MC congeners with varying toxicity, environmental fate, and bioavailability (Niedermeyer Citation2014, Zastepa et al. Citation2014).
MCs have been reported in large eutrophic lakes of the Canadian prairies (Kotak and Zurawell Citation2007, Kotak et al. Citation2011) and the Canadian Shield (Chen et al. Citation2007, Citation2009) but their occurrence is not well characterized. These earlier studies have reported generally low MC concentrations in LOW, but there is some indication that areas of the lake may develop extreme levels; for example a very high concentration of total MCs (>1200 µg MC-LR equivalents/L) was measured in a cyanobacterial bloom sample from Lily Pad Bay (49.7144°N, 94.5503°W) in 1999 (Kling and Hebert unpublished data), although we note that the early ELISA-based methods of detection used in this study were susceptible to cross-reactivity with matrix components, especially in bloom material. A 2004 study measured particulate (particle adsorbed and intracellular) MC-LR equivalents in surface waters at offshore sites throughout the lake, and reported low levels of MCs but a general increase in these toxins over the summer (Chen et al. Citation2007). In later lake-wide summer surveys in 2006 and 2007, Chen et al. (Citation2009) again detected only very low surface water concentrations (<0.69 µg MC-LR equivalents/L) of particulate MC which were well below Health Canada drinking water and recreational guidelines of 1.5 µg MC-LR equivalents/L and 20 µg MC-LR equivalents/L, respectively. These authors found no clear south-north gradient in MC levels and no significant variance between the two years. Both of these earlier studies measured only particulate MC, and while most MCs are retained in cells during active growth, extracellular release can occur at senescence or cell rupture, for example at the end of a bloom or during chemical treatment, resulting in high dissolved concentrations (Zastepa et al. Citation2014).
Species of Microcystis, Dolichospermum, Planktothrix and Pseudanabaena identified in LOW (Watson and Kling Citation2017) are among the cyanobacteria reported elsewhere as MC producers (Chorus Citation2001) and at least some of these are possible sources of these toxins in LOW (Watson and Kling Citation2017). Three recent reports (DeSellas et al. Citation2009, Oblak Citation2009, Environment Canada 2012) stressed the need to fully assess the risks associated with cyanobacterial toxins due to the importance of LOW to recreational users, fisheries, and as a drinking water source. It is clear from the early studies that there is some potential for localized areas of high risk, which to date, remains unassessed. In the current study, we addressed this issue by conducting surveys as well as compiling data from others of total, particulate and dissolved MC toxin concentrations at offshore and inshore sites. This investigation encompassed over nine years of measurements. This work combined two sampling initiatives, i) across-lake collection from a vessel and ii) inshore sampling by cottagers recruited by the Lake of the Woods District Property Owners Association (LOWDPOA). Offshore sub-surface samples were collected in 2006, 2008–2010, and 2014–2015 by lake-wide surveys for toxin analysis in the particulate and dissolved phase. Since cyanobacterial blooms can often accumulate along the inshore areas and beaches, these were also targeted to measure the potential range of spatial and temporal variability in particulate and dissolved MCs. Combining results from this study with data from surveys by Chen et al. (Citation2007 and Citation2009), we evaluated patterns of MCs in relation to physical and chemical conditions of the lake and cyanobacterial taxa potentially capable of their production.
Materials and methods
Lake description
LOW has a surface area of approximately 3850 km2 located within a catchment area of approximately 70,030 km2 (excluding the lake area; Schupp and Macins Citation1977). It is a morphologically and hydrologically complex water body with variable depth (mean ∼8 m, max. ∼66 m). Water flows northward from the Rainy River through several interconnected basins to the Winnipeg River.
Offshore MC measurements at designated stations
Lake segments or zones were based on discrete patterns in water exchange and in total phosphorus (TP) and Chl-a measured in previous studies by Environment and Climate Change Canada (Zhang et al. Citation2012, Watson and Kling Citation2017). These zones (, S1-S6) were used in this study for comparative purposes. Zones S1 and S2 include the large, shallow, polymictic southern basins while zones S3 to S6 make up smaller, deeper, thermally stratified northern basins.
Figure 1. Map of Lake of the Woods (Canada and US) with each of the six ECCC-designated lake zones identified. Dots represent sampling locations offshore in 2006 (brown), 2008–2010 (orange), and 2014–2015 (orange) and nearshore in 2006 (yellow) and 2009 (green).
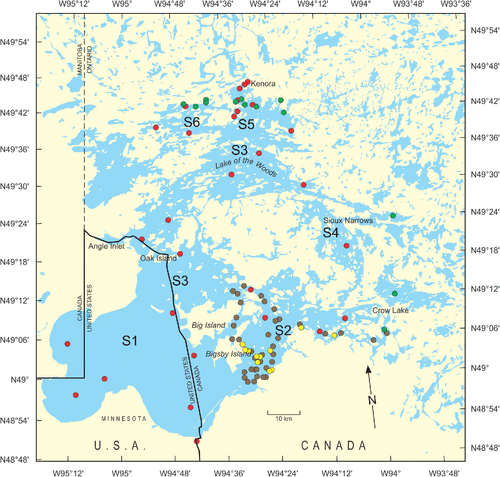
Offshore sampling was conducted in 2006 (n = 62), 2008 (n = 41), 2009 (n = 63), 2010 (n = 22), 2014 (n = 12), and 2015 (n = 16) between June and September (). Whole water samples were collected at a depth of 1 m at all sites by Niskin water sampler, except in 2006 where 10 cm surface grabs were collected. Net samples were collected at select sites by vertical tows of a 20 µm plankton net through the top ∼4 m of the water column. Total MCs were measured from whole water samples, which were frozen at −20 C in 15 mL aliquots in low retention polypropylene tubes until analysis. To obtain the particulate fraction, whole water and net samples were filtered onto GF/C filters and frozen at −20 C until analysis. For analysis by ELISA (Abraxis, PA, US), samples were thawed, tip-sonicated and syringe filtered through 0.45 µm nitrocellulose filters. The manufacturer-specified method detection limit is 0.05 µg MC-LR equivalents/L MC-LR equivalent. Samples were also analysed for toxicity using a protein phosphatase inhibition assay (PPIA) using the method described by Carmichael and An (Citation1999). PPIA measures a broader spectrum of protein phosphatase-based toxicity associated with both MCs and other cyanobacterial peptides. These data were combined with those reported by Chen et al. (Citation2009) for 2006–2007 to evaluate broad patterns in MC concentrations and water quality across LOW.
Nearshore and along shoreline sampling and analysis of phytoplankton blooms for MCs
Nearshore/shoreline sampling was conducted by volunteers in 2006 (n = 55) and 2009 (n = 63) between July and September (). In 2006 and 2009, whole water samples were collected for total MCs i.e. cell-bound and adsorbed on suspended particles (i.e. particulate), and dissolved. In 2006, whole water samples were either collected as an integrated sample from the surface to the euphotic depth as part of the Ontario Lake Partners Program (15 volunteers in total) or as surface samples (collected by AlgalTox International). MC was extracted from whole water samples by ultrasonication. Cell debris was removed by filtering the sonicated sample through 0.45 um nitrocellulose filters and the resulting supernatant was analyzed by PPIA. A total of 18 cottage/camp owners participated in the 2009 study as a result of a request for volunteer involvement published through the LOWDPOA. In June and July of 2009, participants did not observe phytoplankton blooms at their cottages/camps. However, warmer weather in August and September was accompanied by an increase in phytoplankton blooms and the majority of the samples were collected in these latter two months. Sample coolers with sample collection and storage procedures, all necessary sampling equipment (e.g. sampling bottles), and forms for taking field notes was sent to participants. During phytoplankton accumulations, the participants collected water samples from their shoreline or dock area.
Selected shoreline samples with visibly high biomass were subsampled, diluted (to facilitate counting) and preserved with Lugol's iodine for analysis of cyanobacterial species composition and biomass following the standard Utermöhl technique (e.g. Watson and Kling, Citation2017). Cyanobacteria were identified to species when possible and abundance expressed as cells/mL. Biomass (biovolume mg/m3) was estimated for each species by first measuring the dimensions of a subset of the cells for each species and using a volume formula that most closely matched geometric shape for each species. The volume was then converted to biomass using a standard volume-mass formula (Rott et al. Citation1981).
MC congener analysis
Analysis of MC congeners in 2014 samples was conducted using the LC-MS/MS method described in Zastepa et al. (Citation2014) and included the following congeners: MC-RR, -YR, -LR, -7dmLR, -WR, -LA, -LY, -LW, and –LF.
Water quality analysis
Whole water samples were collected from 1 m into acid washed 4 L polyethylene bottles and kept cool and dark until processed (within 24 hours). Analysis of dissolved (0.45 µm filtrate) and particulate (1.2 µm GFC filter) forms of the major nutrients, dissolved inorganic and organic carbon (DIC, DOC), particulate organic carbon (POC), nitrate/nitrite (NO2/3), ammonium (NH4), total Kjeldahl nitrogen (TKN), particulate organic nitrogen (PON), total phosphorus (TP), total dissolved phosphorus (TDP), soluble reactive phosphorus (SRP)), major ions, and Chl-a was carried out at the National Laboratory for Environmental Testing (Burlington, Ontario) using standard methods (National Laboratory for Environmental Testing Citation1997).
Data analysis
A comparison of means from each zone was carried out using a General Linear Models procedure and Tukey's Studentized Range (HSD) Tests (SAS©) to accommodate asymmetric data. Broad correlational patterns between toxins and water quality measures were evaluated using least squares multivariate analyses with backwards selection (α = 0.05); data were log transformed to stabilize the variance. To identify the primary species of cyanobacteria likely producing MC, pairwise correlation analysis was performed in Excel on untransformed shoreline data of cyanobacterial species composition and particulate MC.
Results and discussion
MC concentrations at offshore stations
Average whole water MC levels were generally higher in S1 in all years (, ) where sites were often dominated by the Aphanizomenon flos-aquae species complex, with trace amounts of the potentially toxic Microcystis ichthyoblabe. In S5, where MCs were also relatively high, species of M. novacekii and M. viridis were also present. Total whole water MCs were measured only in September 2010, during which time they were consistently low despite high Chl-a levels (e.g. only 0.7 µg MC-LR equivalents/L measured at 126 µg Chl-a/L at station 760 in S1) (). No measurements exceeded the Health Canada guidelines for drinking and recreational water (1.5 and 20 µg MC-LR equivalents/L, respectively). Particulate MC was measured during 2008, 2009, 2014, and 2015, in part, to compare them with concentrations measured in 2004, 2006, and 2007 by Chen et al. (Citation2007, Citation2009). Particulate MCs showed no clear spatial patterns of variance (); although average values were slightly higher in S1 and S2, reflecting a few high values measured at stations 730 (S1), 510 and 410 (S2) (0.39, 0.42, 0.27 µg MC-LR equivalents/L respectively). LC-MS/MS measurements in samples from 2014 and 2015 showed that MC-LR was the dominant congener in LOW with MC-LA present in minor amounts. This is the first report of MC congener composition in LOW.
Table 1. Summary statistics for surface water (1 m depth) particulate MCs (2006, 2008–2010, and 2014–2015) and total MCs (MC) (2010 only) in LOW in combined sites in each ECCC zone measured using ELISA (or PPIA if noted).
Figure 2. Box and whisker plots of total microcystin (MC) (top; 2010) and particulate MC (bottom; 2008–2009) in 1 m grab samples offshore among ECCC lake zones as measured by ELISA.
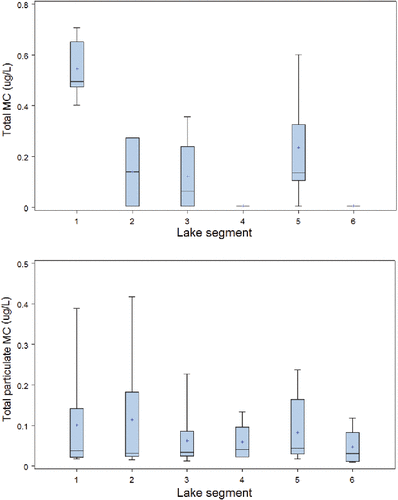
To evaluate the relative toxicity of the phytoplankton community, particulate MC measured by ELISA and PPIA were normalised to Chl-a (). These data showed more distinct among-zone differences, with generally higher average normalized concentrations in the N and NE basins, notably Whitefish Bay (S4), despite its generally low Chl-a levels—although variance about the means meant that these differences were not statistically significant (; ). This suggests that the late summer species assemblages at these sites contain a greater proportion of toxin-producing cells than those in the southern sectors, where the biomass is largely thought to be dominated by species in the Aphanizomenon flos-aquae complex, which to date have not been reported as MC producers. Assignment of microcystin production to A. flos-aquae has been challenging as A. flos-aquae is notoriously difficult to culture (Kozlíková-Zapomêlová E, Academy of Sciences of the Czech Republic, 2013, pers. comm.). Furthermore, accurate identification of A. flos-aquae has been problematic. In cases where isolates have been taxonomically identified as A. flos-aquae, genetic sequencing has suggested otherwise (Li et al. Citation2000, Komárek and Komáreková Citation2006). It is likely that a morphospecies in the A. flos-aquae complex such A. klebahnii or A. yesoense could be the microcystin producer however, further research to confirm which species is needed. PPIA measures were generally higher than corresponding ELISA, suggesting that the overall toxicity may be underestimated by ELISA. since ELISA targets MCs/nodularin while PPIA measures a broader spectrum of protein phosphatase-based toxicity associated with both MCs/nodularin and other cyanobacterial secondary metabolites.
Figure 3. Comparison of Chl-a-normalised total microcystin (MC) analysed using ELISA (top) and PPIA (bottom) across ECCC lake zones, 2008–2009.
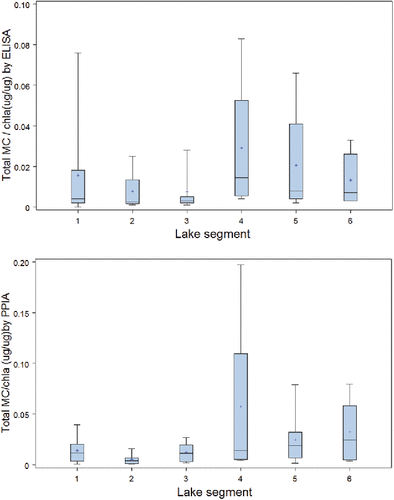
Multivariate analyses of particulate MC against the major physico-chemical parameters showed a strong correlation with NO3 (P < 0.005) but no other nutrient fractions or measures of water transparency and mixing. The relative toxicity of the phytoplankton biomass (Chl-a-normalised MC) was positively related with TDP and inversely with particulate N:P (P < 0.02, P < 0.001 respectively), suggesting lower toxicity in P-deficient assemblages (Guildford and Hecky Citation2000). It is noted, however, that these broad level correlations provide limited insight into the factors influencing toxin production, particularly in view of the significant differences in the physico-chemical regimes across the different LOW embayments and associated phytoplankton assemblages (Watson and Kling Citation2017).
Analysis of combined concentration data from this study with those reported by Chen et al. (Citation2009) showed no systematic change in overall particulate MC levels between 2005 and 2009 (P > 0.05), although highest overall levels were observed in 2008 (). There was significant variance about mean values for each zone and no statistically significant difference among these hydrological sectors was measured. The lack of a measured difference could be a symptom of differences in sampling method (1 m discrete depth vs. 1 m net tows). However, the combined data showed generally higher concentrations in the southern sectors S2 and S3, and among the northern basins in S5 (higher concentrations in Rat Portage and Kenora Bay). Particulate MC showed no relationship with TP or total dissolved phosphorus (TDP), but a highly significant positive relationship with NO3 (P < 0.0003), as seen above with the ECCC dataset, and with NH4 (P < 0.014), as similarly reported by Chen et al. (Citation2009).
Figure 4. Particulate microcystin (MC) from collective data sources, 2006–2009 with a comparison among years (top) and comparison among ECCC zones (bottom).
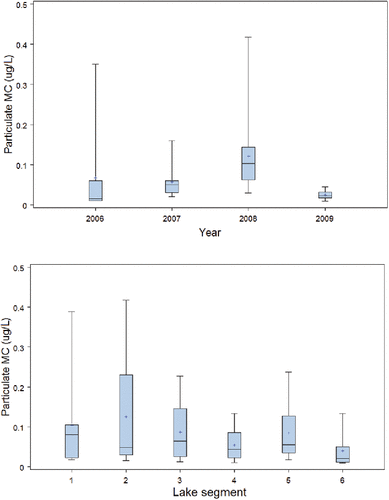
Although the whole water samples discussed thus far contained low toxin concentrations, toxins may become concentrated, for example during calm conditions when buoyancy-regulating cyanobacteria can form surface scums. This was observed during a late summer survey in 2006, which found particulate MC concentrations exceeding 50 µg MC-LR equivalents/L in offshore bloom material near Frenchman's Rock Road (south of Morson, ON). This is further illustrated using net tows collected during a September 2009 survey in the present study, which showed variable and sometimes very high particulate MC concentrations (), particularly at sites 510 (S2), 211 (S3), French Portage Bay (S4), and sites 120 and 140 (S5). The particulate MC concentrations measured in these net tow samples varied between approximately 1 and 190 µg/g dwt; a similarly wide range in PMC was also reported earlier by Chen et al. (Citation2007).
Table 2. Particulate MC measured in net tows, LOW September 2009.
MC concentrations at nearshore and shoreline sites
Inshore samples often contained much higher toxin levels than offshore sites, suggesting locally enhanced toxin production proximal to the shoreline or wind/wave transport of offshore surface scum material towards the shore—a commonly reported phenomenon in many waterbodies. In LOW, concentrations of MCs were generally low (<1 µg MC-LR equivalents/L) in offshore surface samples, but toxin levels along shorelines and beaches were much higher. One-third of the 55 shoreline/beach samples collected at the Frenchman's Rock Road area (S2) in the 2006 survey had particulate MCs in excess of 20 µg MC-LR equivalents/L. The highest concentration recorded was 608 µg MC-LR equivalents/L. In 2009, a total of 63 water samples were collected from shoreline and dock areas (). Some of these samples were collected during phytoplankton blooms (thick, green appearance), while others contained macroscopically identifiable floating particles of phytoplankton. Several shoreline areas contained only low MC concentrations. For example, maximum MC concentrations in Clearwater Bay and Lobstick Bay were less than 0.50 µg MC-LR equivalents/L. Similarly, MC levels were low at Town Island and Channel Island (maximum of 5.4 µg MC-LR equivalents/L near Channel Island). In contrast, several other areas of Lake of the Woods had much higher shoreline concentrations of MC. In increasing order, maximum shoreline concentrations were observed near Frenchman's Rock Road (45.6 µg MC-LR equivalents/L), Pine Portage Bay (59.7 µg MC-LR equivalents/L), Sunbath Island (105.6 µg MC-LR equivalents/L), Nestor Falls (146.6 µg MC-LR equivalents/L), and Bigstone Bay (545.7 µg MC-LR equivalents/L). According to the WHO criteria, all of these maximum concentrations would be considered as high risk for recreational contact. Overall, 25% of the nearshore and shoreline samples collected in 2009 had concentrations of MCs in excess of 20 µg MC-LR equivalents/L.
It is important to note that these MC concentrations are maxima and based on a limited number of samples, and do not imply that high concentrations are always found in these areas of Lake of the Woods. Indeed these areas also experience much lower MC concentrations (). There were some sites with consistently high MC concentrations, as shown by their high mean or median concentrations, notably Bigstone Bay in early September.
Table 3. Mean, median and range of surface water (1 m) MC concentrations in Lake of the Woods in 2009. Concentrations are reported as µg MC-LR equivalents/L.
The concentration of MCs can vary seasonally, from year to year in a lake or even on an hourly basis. Previous studies in western Canada have observed high concentrations of MC in lakes in one year, and low or no MC in other years (Kotak et al. Citation1995, Citation2000). Changes in MC concentration may reflect changes in the species present, their abundance, or the cellular toxin production over time (Kotak et al. Citation1995). Concentration can also vary widely over relatively short distances as a result of wind and water movement (Kotak et al. Citation1995). This often manifests as significant variance in bloom density and toxin levels along shorelines. As part of our study, 14 samples were collected from sites spaced 5 m apart along the shoreline of one of the study areas to examine the variability of MC concentration over a relatively short distance. MC concentrations ranged from 3–170 µg MC-LR equivalents/L (). The most extreme difference was between two adjacent sites which showed MC concentrations of 7.4 and 173.8 µg MC-LR equivalents/L (sites 2 and 3, respectively). This indicates that on a given day areas along this single beach could fall below or far exceed the World Health Organization recreational contact guidelines, suggesting a wide range of risk to the public. It is important to note that there is no routine beach monitoring program for MCs (or other cyanobacterial toxins) on Lake of the Woods, and without collecting and testing samples for toxins or the genes responsible for their production, it is not possible to know whether a bloom is toxic (cf review by Kotak and Zurawell Citation2007). Some of the cyanobacteria identified in LOW have been reported as producers of other cyanobacteria toxins in other systems (anatoxin-a, saxitoxin) (Zurawell et al Citation2005), but to date, these have not been evaluated in this lake. Therefore, a test result that is negative for MC may still be unsafe, due to the presence of these other algal toxins.
Figure 5. Concentration of microcystin (MC) along a shoreline near Frenchman's Rock Road (September 24, 2009). Sampling sites were located 5 m apart and collected within 30 sec. of each other. World Health Organization recreational risk categories: <10 µg MC-LR equivalents/L – Low risk, 10–20 µg MC-LR equivalents/L – Moderate Risk, >20 µg MC-LR equivalents/L – High risk.
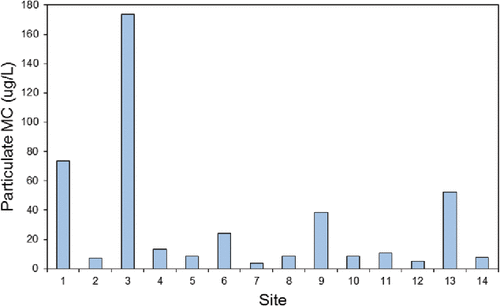
Cyanobacteria species and MC production in Lake of the Woods
A positive correlation was observed between particulate MC concentration and the abundance (cells/mL) and biomass (mg/m3) of several species, although the strength of the correlations in some cases was highly influenced by a single sample. Particulate MC concentration was positively correlated to the abundance of Microcystis ichthyoblabe, Dolichospermum flos-aquae and D. circinale in shoreline samples (). When all species of either Microcystis or Dolichospermum were combined there was also a relationship with MC concentration in shoreline samples (, P < 0.05). All of these species have been shown in other studies to produce MC (e.g. Zurawell et al., Citation2005). There was also a statistically significant correlation (r = 0.76, P < 0.001) between MC concentration and Aphanizomenon spp., although these taxa are not generally reported as toxin producers, and this toxicity may have been derived from small accompanying cyanobacteria associated with the bloom material, such as Pseudanabaena rutilis-viridis recently described from LOW and several other lakes (including Lake Winnipeg) (Kling et al. Citation2012). This small filamentous toxin producer grows free in the plankton or entwined with the colonies of Aphanizomenon flos-aquae complex or other colonial cyanobacteria. We also examined the shoreline samples with MC concentrations exceeding the Health Canada recreational water guideline (i.e., >20 µg MC-LR equivalents/L). A number of species were either dominant (i.e. >50% of the total cyanobacterial biomass in the sample) or co-dominant (i.e. 25–49% of the total cyanobacterial biomass in the sample) in samples where MC concentrations exceeded 20 µg MC-LR equivalents/L (). This examination suggests several species of Microcystis and Dolichospermum as potential producers of MC in Lake of the Woods that should be further investigated. Species that dominated the samples collected from Bigstone Bay (one of the locations with the highest MC concentrations in shoreline samples) included species of Microcystis (M. icthyoblabe and M. flos-aquae), Dolichospermum flos-aquae and Pseudanabaena sp. In an earlier study, Chen et al. (Citation2009) also suggested that MC is likely being produced by species of Dolichospermum, Microcystis and Limnoraphis. It is also noteworthy that species of Dolichospermum, Microcystis and Pseudanabaena are thought to be the main MC producers in Lake Winnipeg, which receives water from LOW via the Winnipeg River (Kotak et al., Citation2011). In LOW, other species such as Pseudanabaena were either dominant or co-dominant in 9% of the shoreline samples containing > 20 µg MC-LR equivalents/L.
Table 4. Correlation analysis between cyanobacterial species abundance and MC concentration in shoreline samples (n = 24).
Table 5. Percent of shoreline samples with MC concentration in excess of 20 µg MC-LR equivalents/L and which cyanobacterial species are either dominant (i.e. >50% of the total cyanobacterial biomass in the sample) or co-dominant (i.e. 25–49% of the total cyanobacterial biomass in the sample).
Conclusion
In this study, we present a first comprehensive assessment of MC measures in LOW from both offshore and inshore samples, and an analysis of these data for spatial and/or temporal trends using measures from several studies conducted between 2006 and 2015. We found no clear long-term trends in microcystins over the years of the surveys. This is consistent with other long-term measures of blooms using satellite imagery (Binding et al. Citation2011), potentially toxic taxa (Watson and Kling Citation2017), and microcystins in sediment core profiles (Zastepa et al. Citation2017). Similarly, we found no clear N-S or other spatial gradient in MC concentrations. However, offshore MC concentrations were consistently higher in three LOW zones (S1, S2, S5) with the first report indicating MC-LR and MC-LA may be the dominant congeners. MC-LA, with a mammalian toxicity as high as MC-LR (Chorus and Bartram Citation2001), appears to be more persistent in aquatic systems (e.g. Newcombe et al. Citation2003, Zastepa et al. Citation2014) and can lead to wildlife fatalities through food web bioaccumulation (Miller et al. Citation2010). Although concentrations of MCs were generally low in samples at offshore sites (<1 µg/L), blooms accumulating along shorelines and beaches frequently had high concentrations (up to ∼600 µg MC-LR equivalents/L, ), posing potential risk to drinking and recreational water in these areas and pointing to a need to sample shorelines at multiple sites to understand the range of toxins along an area. Multivariate analysis of offshore data showed a strong positive correlation of MCs with NO3 (P < 0.005), while the relative toxicity of the algal biomass (Chl-a-normalised MC) was positively related with total dissolved phosphorus (TDP) and inversely with particulate N:P (P < 0.02, P < 0.001 respectively), suggesting lower toxicity in P-deficient assemblages.
These broad trends and correlations provide limited insight into the factors influencing toxin production, particularly in view of the significant differences in the physico-chemical regimes across the different LOW embayments and associated phytoplankton assemblages (Watson and Kling Citation2017) as well as the differences in sampling and analytical methods (Chen et al. Citation2007, Citation2009). The implementation of a monitoring program that considers the complexity of the different embayments and uses standard methods would be better suited to assess sources and drivers of toxin production and future changes in Lake of the Woods. In addition, such a program should include molecular methods to identify taxa responsible for toxin production and measurements of other cyanobacterial metabolites beyond MCs considering that the dominant taxa in most observed blooms in Lake of the Woods are not thought to be a significant source of MC, rather of other toxins and secondary metabolites of concern such as neurotoxins (Cirés and Ballot Citation2016).
Acknowledgments
This study was partly funded by the Lake of the Woods District Property Owners Association (LOWDPOA), through a research grant to AlgalTox International and with support from Environment and Climate Change Canada with special thanks to Tana McDaniel, Tim Pascoe, and the skilled technical team of the Research Support Section for collecting offshore samples. We would like to extend our sincerest thanks to LOWDPOA for funding this important work. In particular, we would like to thank the members of the LOWDPOA Environment Committee for their input and comments on the original proposal, and for their support of the research. This study could not have been possible without the support and direct participation of concerned cottage/camp owners (the “volunteers”) on Lake of the Woods.
References
- Binding CE, Greenberg TA, Bukata RP. 2011. Time series analysis of algal blooms in Lake of the Woods using the MERIS maximum chlorophyll index. J Plankton Res. 33:1847–1852. doi: 10.1093/plankt/fbr079
- Carmichael WW, An J. 1999. Using enzyme-linked immunosorbent assay (ELISA) and a protein phosphatase inhibition assay (PP1 A) for the detection of microcystins and nodularins. Nat Toxins. 7:337–344.
- Chen H, Burke JM, Mossindy T, Fedorak PM, Prepas EE. 2009. Cyanobacteria and microcystin-LR in a complex lake system representing a range in trophic status: Lake of the Woods, Ontario, Canada. J Plankton Res. 31:993–1008.
- Chen H, Burke JM, Dinsmore WP, Prepas EE, and Fedorak PM. 2007. First assessment of cyanobacterial blooms and microcystin-LR in the Canadian portion of Lake of the Woods. Lake Reserv Manage. 23:169–178.
- Chorus I. 2001. Cyanotoxin occurrence in freshwaters—A summary of survey results from different countries. In Cyanotoxins—Occurrence, causes, consequences, ed. Chorus I, pp. 75–78. Berlin: Springer-Verlag
- Cirés S and Ballot A. 2016. A review of the phylogeny, ecology and toxin production of bloom-forming Aphanizomenon spp. and related species within the Nostocales (cyanobacteria). Harmful Algae 54:21–43.
- DeSellas AM, Paterson AM, Clark BJ, Baratono NG, Sellers TJ. 2009. State of the Basin Report for the Lake of the Woods and Rainy River.
- Guildford SJ, Hecky RE. 2000. Total nitrogen, total phosphorus, and nutrient limitation in lakes and oceans: is there a common relationship? Limnol Oceanogr. 45:1213–1223.
- Hargan KE, Paterson AM, Dillon PJ. 2011. A total phosphorus budget for the Lake of the Woods and the Rainy River catchment. J Great Lakes Res. 37:753–763.
- Kling HJ, Laughinghouse HD, Śmarda J, Komárek J, Acreman J, Bruun K, Watson SB, Chen F. 2012. A new red colonial Pseudanabaena (Cyanoprokaryota, Oscillatoriales) from North American large lakes. Fottea. 12:327–339.
- Komárek J, Komáreková J. 2006. Diversity of Aphanizomenon-like cyanobacteria. Czech Phycol. 6:1–32.
- Kotak BG, Zurawell RW. 2007. Cyanobacterial toxins in Canadian freshwaters: a review. Lake Reserv Manage. 23:109–122.
- Kotak BG, Lam AKY, Prepas EE, Kenefick SL, Hrudey SE. 1995. Variability of the hepatotoxin microcystin-LR in hypereutrophic drinking water lakes. J Phycol. 31:248–263.
- Kotak BG, Lam AKY, Prepas EE, Hrudey SE. 2000. Role of chemical and physical variables in regulating microcystin-LR concentration in phytoplankton of eutrophic lakes. Can J Fish Aquat Sci. 57:1584–1593.
- Kotak BG, Gurney S, Herbert C, Kling HJ. 2011. Cyanobacterial (blue-green algal) toxins in Lake Winnipeg. pp. 135–143. In State of Lake Winnipeg: 1999 to 2007. Environment Canada and Manitoba Water Stewardship.
- Li R, Carmichael WW, Liu Y, Watanabe MM. 2000. Taxonomic re-evaluation of Aphanizomenon flos-aquae NH-5 based on morphology and 16 S rRNA gene sequences. Hydrobiologia 438:99–105.
- Miller MA, Kudela RM, Mekebri A, Crane D, Oates SC, Tinker MT, Staedler M, Miller WA, Toy-Choutka S, Dominik C, Hardin D, Langlois G, Murray M, Ward K, Jessup DA. 2010. Evidence for a novel marine harmful algal bloom: cyanotoxin (microcystin) transfer from land to sea otters. Plos ONE 5:e12576.
- National Laboratory for Environmental Testing (NLET). 1997. Schedule of Services. Environment and Climate Change Canada, Canada Centre for Inland Waters, Burlington Ontario, Canada.
- Newcombe G, Cook D, Brooke S, Ho L, Sylman N. 2003. Treatment options for microcystin toxins: similarities and differences between variants. Environ Technol. 24: 299–308.
- Niedermeyer T. 2014. Microcystin congeners described in the literature. Available online: doi:10.6084/m9.figshare.880756. Accessed 25 August 2017.
- Oblak JA. 2009. Water and health in Lake of the Woods and Rainy River for Health Professionals Task Force – International Joint Commission.
- Robertson H, McCracken M. 2003, Magical Mysterious Lake of the Woods. Heartland Associates Inc., Winnipeg, Manitoba.
- Rott E. 1981. Some results from phytoplankton counting intercalibrations. Schweiz Z Hydrol. 43:34–62.
- Schupp DH, Macins V. 1977. Trends in percid yields from Lake of the Woods, 1888–1973. J Fish Res Board Can. 34: 1784–1791.
- Uneo Y, Nagata S, Tsutsumi T, Hasegawa A, Watanabe MF, Park HD, Chen GC, Chen G, Yu SZ. 1996. Detection of microcystins, a blue-green algal hepatotoxin, in drinking water sampled in Haimen and Fusui, endemic areas of primary liver cancer in China, by highly sensitive immunoassay. Carcinogenesis. 17:1317–1321.
- Watson SB, Kling HJ. 2017. Lake of the Woods phyto- and picoplankton: spatiotemporal patterns in blooms, community composition and nutrient deficiency. Lake Reserv Manage. 33:415–432.
- World Health Organization. 2003. Guidelines for safe recreational water environments. Volume I: Coastal and Fresh waters. World Health Organization, Geneva.
- Zastepa A, Pick FR, Blais JM. 2014. Fate and persistence of particulate and dissolved microcystin-LA from Microcystis blooms. Hum Ecol Risk Assess. 20: 1670–1686.
- Zastepa A, Pick FR, Blais JM. 2017. Distribution and flux of microcystin congeners in lake sediments. Lake Reserv Manage. 33:444–451.
- Zhang W, Watson SB, Yerubandi R, Kling HJ. 2012. A linked hydrodynamic, water quality and algal biomass model for a large, multi-basin lake: a working management tool. Ecol Model. 269:37–50.
- Zhou L, Yu H, Chen K. 2002. Relationship between microcystin in drinking water and colorectal cancer. Biomed Env Sci. 15:166–171.
- Zurawell RW, Chen H, Burke JM, Prepas EE. 2005. Hepatotoxic cyanobacteria: a review of the biological importance of microcystins in freshwater environments. J Toxicol Env Health. B 8:1–37.