Abstract
Citrus fruits are a rich source of (poly)phenols, a group of dietary bioactive compounds that protect against developing type 2 diabetes. Our review critically evaluates how experimental in vitro and animal models have elucidated some of the underlying mechanisms on how citrus (poly)phenols affect the markers of type 2 diabetes. According to animal studies, the beneficial effects derived from consuming citrus compounds appear to be related to long-term effects, rather than acute. There are some notable effects from citrus (poly)phenol metabolites on post-absorptive processes, such as modulation of hepatic glucose metabolism and insulin sensitivity in target tissues, but with a more modest effect on digestion and sugar absorption within the gut. Experimental studies on cells and other systems in vitro have indicated some of the possible mechanisms involved, but ∼70% of the studies utilized unrealistically high concentrations and forms of the compounds, compromising physiological relevance. Future studies should discuss the relevance of concentration used in in vitro experiments, relative to the proposed site of action, and also examine the role of catabolites produced by the gut microbiota. Finally, it is important to examine the relationship between the gut microbiota and bioavailability on the action of citrus (poly)phenols.
Introduction
According to the World Health Organization (WHO Citation2016), the global prevalence of diabetes has nearly doubled since 1980, rising from 4.7% to 8.5% in the adult population, of which 90–95% of the cases correspond to type 2 diabetes. High postprandial blood glucose fluctuations and insulin resistance are the key factors increasing the risk of type 2 diabetes (Bhupathiraju et al. Citation2014; Greenwood et al. Citation2013; Livesey et al. Citation2008, Citation2019). (Poly)phenols, a group of compounds naturally present in plants, attenuate some of the risk factors of type 2 diabetes, and also exhibit some anti-inflammatory effects (Abbas et al. Citation2017; Alam et al. Citation2014; Cao et al. Citation2019; Kalt et al. Citation2020; Kwon et al. Citation2007; Tripoli et al. Citation2007; Williamson Citation2017; Williamson, Kay, and Crozier Citation2018). Several epidemiological and human intervention studies suggest increased consumption of (poly)phenol-rich foods is associated with reduced risk of type 2 diabetes and cardiovascular disease (Domínguez-Avila et al. Citation2017; Gee and Johnson Citation2001; Shahidi and Peng Citation2018).
Citrus fruits are widely grown and consumed worldwide, and are a major source of vitamin C and various phytonutrients. Different in vitro, in vivo (animal), and human intervention studies have reported the possible role of citrus fruits and their (poly)phenolic components in attenuating metabolic syndrome (Cerletti et al. Citation2015; Constantin et al. Citation2014; Dong et al. Citation2016; Fujioka et al. Citation2006; Hwang et al. Citation2015; Jung et al. Citation2006; R. W. Li et al. Citation2006; O’Neil et al. Citation2012; Onda et al. Citation2013; de Paiva et al. Citation2019; Ponce, Benassi, and Cesar Citation2019). Among the citrus (poly)phenols, the flavanones, hesperidin 1, narirutin 5, and naringin 6 (Ahmed et al. Citation2017; Constantin et al. Citation2014; Jung et al. Citation2004, Citation2006; Razavi and Hosseinzadeh Citation2019; Zygmunt et al. Citation2010) and the polymethoxyflavones (Gao et al. Citation2018; R. W. Li et al. Citation2006; Onda et al. Citation2013) are the most studied compounds. According to in vitro and animal studies, citrus flavanones exert their anti-diabetic activity via several mechanisms; inhibition of gut glucose transporters, increase in insulin secretion, increased insulin sensitivity in target tissues and reduced hepatic glucose production and output (Ahmed et al. Citation2017; Erlund et al. Citation2001; Jung et al. Citation2004, Citation2006; Kerimi, Gauer, et al. Citation2019).
(Poly)phenols can act either within the gut and/or after absorption on different tissues, including the pancreas, liver, muscle, and adipose tissues, and these two processes are mostly independent. In the gut, modulation of glycemic response is possible mainly by affecting starch digestion and absorption (Sun and Miao Citation2020; Williamson Citation2013). After absorption, mechanisms include modulation of hepatic glucose metabolism and increase insulin secretion and sensitivity. Understanding the mechanism of action of (poly)phenols is crucial for the correct and appropriate design of human studies. For example, it is not worthwhile to perform a short study (≤2 hr) for a compound that takes longer than 2 hr to reach the circulation and acts mainly via post-absorptive mechanisms. The majority of the data on the mechanism of action of (poly)phenols originate from in vitro and animal studies. Hence, the following review paper mainly focuses on unraveling the anti-diabetic mechanism of citrus (poly)phenols by critically analyzing the experimental data, and also highlights approaches for future research.
Citrus (poly)phenols
Citrus fruits are rich in flavonoids, especially which constitute 90% of the (poly)phenols in citrus fruits, mostly as glycosides of hesperetin 8 and naringenin 9 (De Ancos et al. Citation2017; Ballistreri et al. Citation2019; Khan, Zill-E-Huma, and Dangles Citation2014). Citrus fruits are the main food source of hesperidin 1 and naringin 6 (Rampersaud and Valim Citation2017) and these compounds have not been detected in apples, berries (strawberry, bilberry, and cranberry), black grape nor sour cherries (Díaz-García et al. Citation2013). The (poly)phenol content and profile of citrus fruits depend mainly on species and variety. For example, hesperidin 1 is the main flavanone found in oranges, mandarins, lemons, and lime, while grapefruits contain naringin 6 in high concentrations (), contributing to the bitter taste (Díaz-García et al. Citation2013; Gattuso et al. Citation2007; Rampersaud and Valim Citation2017; Turner and Burri Citation2013). Apart from the flavanones, another group of compounds found almost exclusively in citrus fruits is the polymethoxyflavones (PMFs), particularly concentrated in the peel and flavedo of the fruit (Gao et al. Citation2018). Tangeretin 12 and nobiletin 13, the two most abundant PMFs found widely in citrus fruits, have been extensively studied owing to their distinct pharmacological properties (Gao et al. Citation2018; R. W. Li et al. Citation2006). lists the (poly)phenol composition of orange, grapefruit, and lime juices, and the chemical structures of some of the predominant flavanones and polymethoxyflavones (PMF) in citrus fruits are illustrated in .
Table 1. (Poly)phenol composition of citrus juices.
Citrus fruit juices also contain flavone and flavonol glycosides of apigenin, luteolin, tamarixetin, isorhamnetin, kaempferol, and quercetin (Abad-García et al. Citation2012; Díaz-García et al. Citation2013; Gattuso et al. Citation2007; Sun et al. Citation2019; Tomás-Navarro, Vallejo, and Tomás-Barberán Citation2014). Considerable amounts of some flavones have been reported in C. limon (diosmetin 6,8-di-C-glucoside − 4.95 mg/100 mL, apigenin 6,8-di-C-glucoside (vicenin-2) − 1.17 mg/100 mL) and in C. sinensis (vicenin-2 − 5.72 mg/100 mL) juices (Gattuso et al. Citation2007; Tomás-Navarro, Vallejo, and Tomás-Barberán Citation2014). Phenolic acids such as 3′,4′-dihydroxycinnamic acid (caffeic acid), 4-hydroxy-3-methoxybenzoic acid (vanillic acid), 4′-hydroxycinnamic acid (p-coumaric acid), 3,4-dihydroxybenzoic acid (protocatechuic acid), 4′-hydroxy-3′-methoxycinnamic acid (ferulic acid), 4-hydroxy-3,5-dimethoxy-cinnamic acid (sinapic acid), and p-hydroxybenzoic acids were also detected in seven citrus varieties (Sun et al. Citation2019). The presence of chlorogenic and gallic (3,4,5-trihydroxybenzoic) acids have also been reported (Kelebek et al. Citation2009; Sdiri et al. Citation2012), but anthocyanins are rare and limited to some specific pigmented varieties of sweet and blood oranges and lemons (Ballistreri et al. Citation2019; Fallico et al. Citation2017).
Data from in vitro and animal studies
Possible antidiabetic activity of citrus (poly)phenols before absorption (in the gut)
In general, within the gut, the (poly)phenols mainly exert any hypoglycemic effects by attenuating carbohydrate digestion and absorption. They reduce starch digestion by inhibiting the starch digesting enzymes and blunt intestinal glucose absorption by either inhibiting the glucose transporters or by affecting the gene expression of these transporters (Kerimi, Nyambe-Silavwe, et al. Citation2019; Manzano and Williamson Citation2010; Nyambe-Silavwe et al. Citation2015; Lo Piparo et al. Citation2008; Rasouli et al. Citation2017; Schulze et al. Citation2014; Tadera et al. Citation2006; Villa-Rodriguez et al. Citation2017; Wu and Tian Citation2017). Inhibition of both the starch hydrolyzing enzymes and transporters in the gut slows down the release of glucose into the circulation thereby preventing hyperglycemic episodes, a risk factor for the development of type 2 diabetes (Bhupathiraju et al. Citation2014; Greenwood et al. Citation2013; Livesey et al. Citation2008, Citation2019). illustrates the overall summary of results extracted from studies published so far on the effect of citrus (poly)phenols on starch digesting enzymes and sugar transporters using both in vitro and animal studies.
Figure 2. Potential inhibitory mechanisms of citrus (poly)phenols on starch digestion and absorption within the gut. SGLT1 (Sodium-dependent glucose cotransporter 1), GLUT2 (Glucose transporter 1), and GLUT5 (Glucose transporter 5) are sugar transporters located in the brush border membrane.
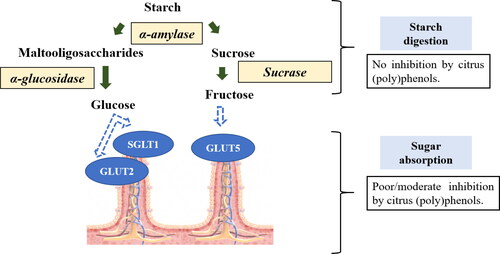
Effect on starch hydrolyzing enzymes
Salivary and pancreatic α-amylases, and the intestinal brush border α-glucosidases (maltase-glucoamylase and sucrase-isomaltase), are the key enzymes involved in digesting dietary starch in humans (Butterworth, Warren, and Ellis Citation2011; Goodman Citation2010; Lin, Lee, and Chang Citation2016; Pyner, Nyambe-Silavwe, and Williamson Citation2017; Visvanathan et al. Citation2019). α-Amylase hydrolyzes starch to maltooligosaccharides by acting upon the internal α-1,4-glycosidic linkages. Since α-amylase is an endo-acting enzyme, the products are maltodextrins with no free glucose (Butterworth, Warren, and Ellis Citation2011; Visvanathan et al. Citation2019, Visvanathan, Houghton, and Williamson Citation2021). The digested maltodextrins are then concomitantly transformed into glucose by the action of intestinal brush border α-glucosidases (Kerimi, Nyambe-Silavwe, et al. Citation2019; Lin, Lee, and Chang Citation2016; Pyner, Nyambe-Silavwe, and Williamson Citation2017). Thus, inhibition of these two enzymes aid in attenuating postprandial glucose peaks after a meal. The inhibitory activity of dietary (poly)phenols on starch digesting enzymes is well documented (Fei et al. Citation2014; Nyambe-Silavwe et al. Citation2015; Pyner, Nyambe-Silavwe, and Williamson Citation2017; Tadera et al. Citation2006; Visvanathan, Houghton, and Williamson Citation2021). However, the inhibitory activity of citrus (poly)phenols on these enzymes is not yet clearly defined and studies done using enzymes from human sources are rare (). This makes interpretation difficult, as enzymes from other sources such as microbial enzymes have very little sequence homology or similarity in inhibitory patterns to human enzymes, and mammalian enzymes show some similarities but also some important differences. For example, Priscilla et al. (Citation2014) clearly show the influence of enzyme sources on the inhibitor by (poly)phenols. In that work, the inhibitory potential of naringenin 9 toward α-glucosidase from yeast and rat intestine was studied. Naringenin 9 showed dramatically better inhibitory potential against yeast α-glucosidase (IC50 = 6.51 µM) compared to rat intestinal α-glucosidase (IC50 = 384 µM). The opposite was observed with acarbose (IC50 = 49.65 and 2.45 µM for the yeast and mammalian α-glucosidases, respectively). Similarly, a comparison between human and rat intestinal α-glucosidases revealed EGCG to show differential inhibition of enzymes from different sources (Pyner, Nyambe-Silavwe, and Williamson Citation2017).
Table 2. In vitro studies on citrus compounds and the markers of type 2 diabetes.
Few studies have reported the effect of pure citrus extracts or the constituent (poly)phenols on these enzymes (). According to Kerimi, Gauer, et al. (Citation2019), hesperidin 1 did not exhibit any potential inhibition toward rat intestinal sucrase and human α-amylase. Similarly, hesperidin 1, naringin 6, neohesperidin 4, and nobiletin 13 showed weak inhibition against the digestive enzymes but did show good starch binding ability, which significantly delayed starch digestion (Shen, Xu, and Lu Citation2012). All four compounds exhibited poor α-glucosidase (yeast) inhibitory activity, and for α-amylase (porcine), nobiletin 13 showed stronger inhibition compared to the other three citrus flavonoids. However, the inhibitory potential was below 30% even at the highest concentration tested (160 µM). Dangyuja (Citrus grandis Osbeck) water extract, containing mainly neohesperidin 4 (160 mg/g extract) and naringin 6 (110 mg/g extract), showed weak inhibition toward rat intestinal α-glucosidase and no inhibitory activity on porcine pancreatic α-amylase (Kim, Shin, and Jang Citation2009). The aglycones hesperetin 8 and naringenin 9, obtained from hesperidinase- and naringinase-treated extract, improved the inhibitory potential moderately (Kim, Shin, and Jang Citation2009). In a study done using human pancreatic α-amylase, an extract from a cross-bred citrus variety (Clementine and Tarocco tetraploids) strongly inhibited α-amylase, close to the activity of the commercially used anti-diabetic drug acarbose () (Casacchia et al. Citation2019). However, more studies using human enzymes are required but, so far, published studies do not indicate promising starch digesting enzyme inhibitory effect from citrus (poly)phenols at physiologically relevant concentrations.
Effect on the glucose transporters
Few studies have reported the possible inhibitory effect of citrus components on glucose absorption in the gut ( and ). Hesperidin 1, hesperetin 8, and whole orange juice inhibited the glucose transporters, GLUT2, SGLT1, and GLUT5 in Caco-2/TC7 cell monolayers (Kerimi, Gauer, et al. Citation2019). Glucose is primarily absorbed by SGLT1 and GLUT2 transporters in the small intestine, whereas GLUT5 is mainly involved in fructose absorption. Orange juice reduced glucose transport by 43% in differentiated Caco2/TC7 cells, compared to a sugar-matched control (Kerimi, Gauer, et al. Citation2019). Hesperidin 1 concentrations of 80 and 800 µM significantly inhibited glucose transport in Caco-2/TC7 cells but at very high concentrations well above that observed in orange juice (1600 µM), hesperidin 1 appeared to enhance glucose transport (Kerimi, Gauer, et al. Citation2019). The solubility of hesperidin 1 in aqueous systems is very low with maximum solubility in pure water not exceeding 40 µM (Tommasini et al. Citation2005). The authors state (Kerimi, Gauer, et al. Citation2019) that hesperidin 1 is soluble up to a concentration of 800 µM when first dissolved in DMSO and then introduced into orange juice, so presumably some factor keeps hesperidin in an apparent solution-like state. Solubility of hesperidin 1 at higher concentrations (>800 µM) is poor and so some effects could be due to a suspension of hesperidin at such high concentration. This could explain why the 1600 µM is less active than the 800 µM in some systems, for example. Solubility will certainly affect activity given that (poly)phenol solubility is a major factor determining bioavailability and bioactivity (Cao et al. Citation2018; Nguyen et al. Citation2015; Vallejo et al. Citation2010).
Table 3. In vivo studies using animal models on citrus compounds and type 2 diabetes.
Hesperidin 1 lowered glucose absorption in differentiated Caco-2/TC7 cells by inhibiting glucose transporters (Kerimi, Gauer, et al. Citation2019). Neohesperidin 4 inhibited absorption of D-[6-3H] glucose in Caco-2/TC7 cells by approximately 50% at a concentration of 100 µM, mainly by interacting with SGLT1. In comparison to hesperidin 1, the aglycone hesperetin 8 more potently inhibited glucose transport in Xenopus oocytes by inhibiting GLUT2, whereas fructose transport (inhibition of GLUT5) was better inhibited by hesperidin 1 (Kerimi, Gauer, et al. Citation2019). Similarly, hesperetin 8 strongly inhibited glucose and fructose absorption in Xenopus oocytes expressing SGLT1, GLUT2, and GLUT5 (Kwon et al. Citation2007). Naringenin 9 showed moderate inhibition, with no effect seen for naringin 6 () (Kwon et al. Citation2007). Naringenin 9 strongly inhibited SGLT1-assisted glucose uptake in everted intestinal sleeves of normal and diabetic rats, with no effect seen with naringin 6 (J. M. Li et al. Citation2006).
Naringenin 9 modulated blood glucose response in normal and diabetic mice mainly by regulating intestinal glucose absorption (Ortiz-Andrade et al. 2008). Administration of naringenin 9 (0, 10, 30, and 50 mg/kg body weight (bw)) reduced intestinal glucose absorption in a dose-dependent manner (>50%) in insulin-resistant rats, tested from blood drawn from the portal vein of the rats 30 min after an oral dosage of 2 g/kg bw glucose (Kannappan and Anuradha Citation2010). In contrast, grapefruit juice (3 mL/kg bw), a good source of naringin 6, did not change blood glucose response in healthy rats after a glucose tolerance test (3.0 g/kg bw glucose), regardless of the route of administration (oral vs. intraperitoneal), indicating no effect of grapefruit (poly)phenols on intestinal glucose absorption (Owira and Ojewole Citation2009). In another study, grapefruit juice (3 mL/kg bw) improved glucose intolerance in streptozotocin (STZ)-induced diabetic rats after an intraperitoneal dose of 3.0 g/kg bw glucose, suggesting a post-absorptive mechanism from the grapefruit components in reducing glucose response (Hayanga et al. Citation2016). Although the aglycones (hesperetin 8 and naringenin 9) appear to show promising inhibitory effects, it should be noted that the aglycones are present in extremely low levels in the fruits naturally, and are less likely to be hydrolyzed in the small intestine to exert any effect on the starch digesting enzymes or the glucose transporters (Actis-Goretta et al. Citation2015; Borges et al. Citation2010, Citation2013). Another point to note is that some studies examine blood glucose in rats while anesthetized (Kannappan and Anuradha Citation2010). In addition to the body being under stress during anesthesia, it also reduces gastrointestinal motility and affects the digestion and absorption processes (Tweedle and Nightingale Citation1989). Thus, the disturbed metabolic function in rats could have also contributed to the favorable effect seen with naringenin 9. In summary, although no strong effects were seen, the few glucose transport studies done so far ( and ) indicate weak to moderate inhibition by the citrus components. More studies are recommended to explore the role of citrus (poly)phenols on glucose transporters using the natural juice and appropriate components of citrus fruits (not the aglycones), including the PMFs at physiologically relevant concentrations that are attainable in the gut.
Possible anti-diabetic activity of citrus (poly)phenols after absorption
In vitro and animal studies report that citrus (poly)phenols affect the markers of type 2 diabetes in different cells and tissues mainly by affecting hepatic glucose metabolism, increasing insulin secretion in the pancreas, and increasing insulin sensitivity in insulin target tissues ( and ). A summary of the proposed post-absorptive anti-diabetic mechanisms of citrus (poly)phenols is depicted in . However, shortcomings in the reported studies make conclusive interpretations difficult. For example, ∼70% of the in vitro studies (post-absorptive mechanism) listed in have utilized unrealistic (poly)phenol doses and compounds, thereby compromising physiological relevance. Thus, in the following sections, in addition to discussing the results, the study designs are critically evaluated for their physiological relevance and practical application.
Figure 3. Post-absorptive antidiabetic mechanism of citrus (poly)phenols derived from in vitro and animal models. Abbreviations: ↑ – Increase, ↓ – Decrease, PI3-K – Phosphatidylinositol 3 kinase, AMPK – Adenosine monophosphate-activated protein kinase, PPAR-γ – Peroxisome proliferator-activated receptor gamma, GLUT4 – Glucose transporter 4, GK – Glucokinase, PEPCK – Phosphoenolpyruvate carboxykinase, G6P – Glucose-6-phosphatase.
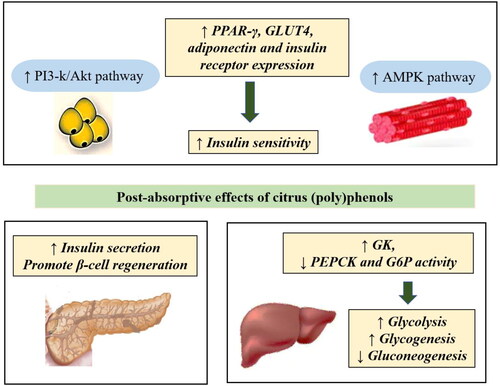
Effect on hepatic glucose homeostasis
The liver is an important metabolic organ that maintains systemic glucose homeostasis by regulating gluconeogenesis, glycogenolysis, glycolysis and other pathways (Petersen, Vatner, and Shulman Citation2017). The liver alone contributes to ∼90% of total endogenous glucose. Citrus flavonoids have been proposed to enhance hepatic glycolysis and glycogen content and reduce hepatic gluconeogenesis ( and ). Both cell and mouse experimental models suggest that citrus flavonoids modulate hepatic glucose metabolism by increasing hepatic glucokinase (GK) and reducing phosphoenolpyruvate carboxykinase (PEPCK) and glucose-6-phosphatase (G6P) mRNA expression and activity (Akiyama et al. Citation2009; Jung et al. Citation2004, Citation2006; do Nascimento et al. Citation2018; Park et al. Citation2013; Shen, Xu, and Lu Citation2012). GK, PEPCK, and G6P play a pivotal role in liver glucose homeostasis and are potential pharmacological targets for the treatment of type 2 diabetes (Rines et al. Citation2016; Sharabi et al. Citation2015). GK is involved in cellular glucose usage and PEPCK and G6P in glucose production (Sharabi et al. Citation2015). The diabetic state lowers GK activity, and elevates PEPCK and G6P levels (Jung et al. Citation2006; Rines et al. Citation2016). Thus, even a small change brought about by (poly)phenols in the expression of these genes could conceivably aid in improving diabetic outcomes. Citrus flavonoids, hesperidin 1, neohesperidin 4, naringin 6, and nobiletin 13 are reported to stimulate glycolysis, increase hepatic glycogen level, and reduce gluconeogenesis in HepG2 cells, mainly via modulating the activity of the enzymes, GK and G6P (Shen, Xu, and Lu Citation2012). The flavonoids significantly elevated the activity of GK and reduced the activity of G6P at concentrations of 10 and 50 µM, which was almost comparable to the activity of the commercial drug metformin (1 mM) (Shen, Xu, and Lu Citation2012), a well-known pharmacological agent used to treat type 2 diabetes acting mainly via suppressing hepatic gluconeogenesis (Petersen, Vatner, and Shulman Citation2017). Though the study determines a favorable effect from these compounds in hepatic glucose homeostasis, it is noteworthy that none of these compounds are found in circulation and the study dose is far above the normal range reported for the flavanone metabolites (maximum 2.2 and 6 µM for hesperetin 8 and naringenin 9 metabolites), thus making the study outcomes physiologically questionable. In general, most of the flavanone glycosides pass unaltered in the small intestine to the colon, are deglycosylated by the gut microbes, absorbed into the colonocytes, and then subjected to phase II metabolism in the colon and/or liver (Brett et al. Citation2008; Pereira-Caro et al. Citation2015, Citation2016; Tomás-Navarro et al. Citation2014; Williamson, Kay, and Crozier Citation2018). The main flavanone metabolites detected in plasma and urine are glucuronide, sulfate, and sulfo-glucuronide conjugated derivatives of hesperetin 8 and naringenin 9 ().
Figure 4. Structures of main hesperetin and naringenin metabolites. Compounds detected in plasma/urine after consuming orange juice, based on data of Pereira-Caro et al. (Citation2014, Citation2016).
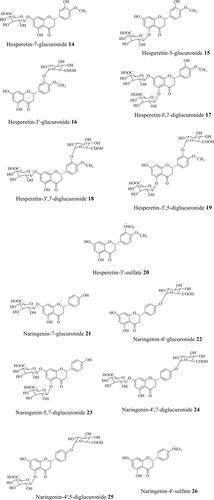
Several studies have reported the positive effect of hesperidin 1 and naringin 6 on hepatic glucose homeostasis in normal and diabetic mice (Akiyama et al. Citation2009, Citation2010; Jung et al. Citation2006, Citation2004; Pu et al. Citation2012). Sweetened clarified grapefruit juice and naringin 6 showed similar anti-diabetic effects as metformin in C57BJ/6 mice fed a high-fat diet (Chudnovskiy et al. Citation2014). Ingestion of clarified grapefruit juice for 100 days reduced the fasting blood glucose level by 13 − 17%, fasting serum insulin level by three-fold and improved glucose intolerance, compared to the control group (Chudnovskiy et al. Citation2014). Hesperidin 1 and naringin 6 significantly up regulated the mRNA expression of hepatic GK in the liver of db/db mice, with naringin 6 additionally suppressing the expression of PEPCK and G6P (Jung et al. Citation2006). The changes observed in mRNA expression were in conjunction with the respective enzyme activities (Jung et al. Citation2004). Furthermore, hesperidin 1 and naringin 6 also significantly increased hepatic glycogen content compared to the diabetic control, indicating the positive effect of these two compounds on glycogenesis and glycogenolysis (Jung et al. Citation2004). Though naringin 6 appeared to be better in regulating hepatic glucose homeostasis than hesperidin 1, the blood glucose-lowering effect was similar for both hesperidin 1 and naringin 6 (Jung et al. Citation2004, Citation2006). Another study carried out with grapefruit juice reported improvement in fasting glucose levels and glucose tolerance, with no effect on the fasting plasma insulin levels of STZ-diabetic rats (Hayanga et al. Citation2016). Grapefruit juice suppressed hepatic gluconeogenesis by significantly enhancing GK activity and hepatic glycogen content and lowering G6P and PEPCK activities (Hayanga et al. Citation2016). Additionally, naringin 6 (0.02% in the diet) significantly reduced PEPCK and G6P mRNA expression by 47% and 62%, respectively, compared to the diabetic control mice (Pu et al. Citation2012).
Oral administration of 100 mg tangeretin 12/kg bw to diabetic rats for 30 days significantly decreased plasma glucose and glycosylated hemoglobin (HbA1c) levels and returned insulin level to near normal (Sundaram, Shanthi, and Sachdanandam Citation2014). Tangeretin 12 treatment reduced blood glucose and HbA1c level in diabetic rats by 2.4- and 2.1-fold, respectively, compared to the control. The anti-diabetic effect exerted by tangeretin 12 was comparable to the activity of the commercial drug glibenclamide (dose 5 mg/kg bw). Furthermore, tangeretin 12 treatment restored liver weight to normalcy, probably as a result of decreased glycogenolysis, protein degradation, and gluconeogenesis in the diabetic rats, and restored the activity of the hepatic carbohydrate metabolizing enzymes hexokinase, pyruvate kinase, lactate dehydrogenase, glucose-6-phosphatase, fructose-1,6-bisphosphatase, glucose-6-phosphate dehydrogenase, glycogen synthase and glycogen phosphorylase to normalcy (Sundaram, Shanthi, and Sachdanandam Citation2014). Similarly, the other most common PMF found in citrus fruits, nobiletin 13, was also reported to improve glucose homeostasis in obese diabetic ob/ob mice treated with nobiletin 13 (200 mg/kg bw) for 5 weeks (Lee et al. Citation2010). Nobiletin 13 treatment significantly reduced blood glucose concentration and HOMA-IR (homeostatic model assessment of insulin resistance), and improved glucose tolerance, plasma adiponectin levels, and also significantly decreased the mRNA expression of PEPCK and G6P in diabetic mice, with no change observed in their respective protein levels (Lee et al. Citation2010). The decrease in mRNA expression of PEPCK and G6P in the nobiletin 13-treated group was 66% and 71%, respectively, compared to the control. Although the PMFs appear to show promising effects in regulating hepatic glucose production and output, the doses used in both studies discussed here are quite high, not achievable just by consuming citrus fruits. The amount of PMF delivered from drinking 250 mL of orange juice is less than 2 mg (Gattuso et al. Citation2007). Thus, the health effects mentioned for the PMFs need to be reassessed with studies using physiologically relevant and practically achievable doses.
Similarly, some studies reporting the possible favorable effect of flavanone aglycones on liver glucose homeostasis, especially the in vitro studies, have also used extremely high concentrations, up to 300 μM (Constantin et al. Citation2014; do Nascimento et al. Citation2018). Though flavanones are abundantly present in citrus fruits, especially in grapefruit and orange fruits and juices, the peak plasma concentrations reported for naringenin and hesperetin metabolites (, 14–26) from studies published so far range from 0.04 − 6 μM and 0.07 − 2.2 μM, respectively (Actis-Goretta et al. Citation2015; Erlund et al. Citation2001; Vallejo et al. Citation2010), which is far below the concentrations employed in most of the studies listed in . Even ingestion of grapefruit juice with ∼1000 μM naringenin 9, did not raise the plasma naringenin 9 level beyond 6 μM (Erlund et al. Citation2001).
Effect on insulin secretion
Insulin plays a key role in glucose homeostasis and is critical in the development of type 2 diabetes (Cantley and Ashcroft Citation2015; Fu, Gilbert, and Liu Citation2012; Röder et al. Citation2016). Insulin is the main hormone involved in lowering blood glucose levels, and is counteracted by several hormones, including glucagon, epinephrine, and glucocorticoids, which can elevate blood glucose concentration (Aronoff et al. Citation2004; Cantley and Ashcroft Citation2015). Type 2 diabetes can occur as a result of insulin insufficiency and/or impaired insulin action in target tissues such as the liver, muscle and adipose tissues. Chronic (72 hr) and acute naringenin 9 treatment, dose-dependently (10−10, 10−8, 10−6 M) enhanced glucose-stimulated insulin secretion in INS-1E cells (rat-derived pancreatic β-cells) (Bhattacharya et al. Citation2014). Naringenin 9 demonstrated a more pronounced effect on insulin secretion than caffeic acid or quercetin (Bhattacharya et al. Citation2014). The presence of naringenin 9 (367 μM) increased glucose-stimulated insulin secretion by ∼1.5-fold in human embryonic stem cell-derived pancreatic endoderm cells (Xing, Yang, and Wu Citation2016). Once again, the concentration of naringenin 9 utilized in the study is far higher than the concentration of naringenin metabolites (, 21–26) normally found in the circulation, and so the results are unlikely to be physiologically relevant (). However, the same favorable effect was seen in the pancreatic endoderm transplanted gestational diabetic mice, where feeding 50 mg/kg bw naringenin 9 for 20 days increased the body weight and restored the blood glucose and insulin levels to normalcy (Xing, Yang, and Wu Citation2016).
Treatment with 0.02% hesperidin 1 and naringin 6 supplemented diet for 5 weeks increased serum insulin and C-peptide levels in the db/db mice with type 2 diabetes (Jung et al. Citation2004). C-peptide is widely used to assess the insulinogenic effect of compounds due to its comparatively longer half-life compared to insulin (Jones and Hattersley Citation2013). There was a 2-fold increase in the C-peptide level in naringenin 9-fed (100 mg/kg bw, 4 weeks) type 2 diabetic rats (Ahmed et al. Citation2017). Furthermore, naringenin 9 protected pancreatic islets of rats from streptozotocin-nicotinamide (STZ/NA)-induced oxidative stress and promoted regeneration of β-cells and reduced vacuolation of the pancreatic islets (Annadurai et al. Citation2012). Similar findings have been reported in STZ-diabetic rats fed a citrus peel extract where the extract improved insulin secretion and reversed the detrimental effect of STZ on β-cells (Sathiyabama et al. 2018). In support, several other studies have reported the positive effect of citrus extract or its (poly)phenols on the insulin level of diabetic rats (Parmar et al. Citation2012; Sundaram, Shanthi, and Sachdanandam Citation2014).
Inhibition of the dipeptidyl peptidase-4 (DPP-4) enzyme is proposed as a potential therapeutic strategy to improve blood glucose concentration (Deacon Citation2019), and there are several DPP-4 inhibitors (gliptins) currently in clinical use (Deacon Citation2019; Gupta et al. Citation2018). DPP-4 inactivates the incretin hormones, glucagon-like-peptide-1 (GLP-1), and glucose-dependent insulinotropic polypeptide (GIP), which are involved in increasing insulin secretion and sensitivity, and simultaneously reduces glucagon secretion from the pancreas. Citrus flavonoids potentially inhibit DPP-4 activity in vitro and in vivo (Fan et al. Citation2013; Gupta et al. Citation2018; Parmar et al. Citation2012; Proença et al. Citation2019). The citrus flavonoids, hesperetin 8, and naringenin 9 inhibited DPP-4 activity ∼15-fold more effectively than the positive control Diprotin A (Fan et al. Citation2013) (). Another study reported that naringin 6 inhibited DPP-4 activity to a similar extent as sitagliptin (Parmar et al. Citation2012). In the same work, feeding naringin 6 (40 mg/kg bw) for 9 days significantly reduced the serum DPP-4 activity by 31% and increased serum insulin level by 68% in Wistar rats compared to the control. However, recent studies have reported citrus flavonoids to be extremely weak inhibitors of human recombinant DPP-4 (Gupta et al. Citation2018; Proença et al. Citation2019). Fan et al. (Citation2013) used DPP-4 derived from porcine kidneys, and the in vitro study by Parmar et al. (Citation2012) does not mention the source. As discussed before, the activity of the (poly)phenols varies widely based on the source of the enzyme. Results gained from studies using human-derived enzymes are considered more applicable to human nutrition. Thus, it is difficult to comment on the effect of citrus (poly)phenols on DPP-4 based on just two studies, and further work is recommended to explore the effect of citrus (poly)phenols on DPP-4.
Effect on insulin sensitivity
Citrus (poly)phenols improve insulin sensitivity in the liver and peripheral tissues by modulating several metabolic pathways through the expression of various genes and proteins (Ahmed et al. Citation2017; Erlund et al. Citation2001; Jung et al. Citation2004, Citation2006; Shen, Xu, and Lu Citation2012; Zygmunt et al. Citation2010). Citrus sinensis (L.) Osbeck (sweet orange) peel extract significantly improved HOMA-IR in STZ-diabetic rats, which was comparable to metformin (Sathiyabama et al. 2018). Further, compared to the diabetic control, citrus extract supplementation in the diet (50 and 100 mg/kg b.w) for 30 days significantly up-regulated peroxisome proliferator-activated receptor gamma (PPAR-γ), GLUT4, and insulin receptor mRNA and protein in diabetic mice (Sathiyabama et al. 2018). The glucose-lowering effect of the citrus extract was postulated to be through activation of PPAR-γ, which in turn stimulated adipocyte differentiation, insulin receptor signaling, and translocation of GLUT4 from the cytosol to the plasma membrane in the adipose tissue in an insulin-dependent manner via affecting the phosphoinositide-3-kinase/protein kinase B (PI3-k/Akt) pathway (Sathiyabama et al. 2018). PPARs are a group of nuclear hormone receptors involved in regulating the expression of genes involved in glucose homeostasis, predominantly expressed in adipose tissues but also found in other tissues such as the liver, heart, and skeletal muscle (Kim and Ahn Citation2004; Vidal-Puig et al. Citation1997). PPAR-γ improves the insulin-sensitizing effects by increasing the expression of GLUT4 in adipose tissues, directly activates hepatic GK expression, inhibits hepatic gluconeogenesis, and also increases the glucose-sensing ability of pancreatic β-cells (Jung et al. Citation2006; Kim and Ahn Citation2004). In another study, hesperidin 1 and naringin 6 were reported to act as agonists for PPAR-γ in db/db mice (Jung et al. Citation2006). The two (poly)phenols significantly increased PPAR-γ protein expression by about 3-fold, but not PPAR-α, in the liver and adipose tissues of diabetic mice. Hesperidin 1 and naringin 6 reduced GLUT2 protein expression in hepatocytes of diabetic mice, a transporter that is normally upregulated in hepatocytes in a diabetic state (Chadt and Al-Hasani Citation2020), and increased hepatic GK mRNA expression, thereby reducing hepatic glucose output (Jung et al. Citation2006). Moreover, GLUT4 protein expression was increased in adipocytes by hesperidin 1 and naringin 6 compared to the control group, indicating enhanced insulin sensitivity and uptake of glucose into adipose tissues in the flavanone treated group (Jung et al. Citation2006).
Key pathways modulated by citrus (poly)phenols affecting insulin sensitivity Citrus (poly)phenols are reported to improve insulin sensitivity by modulating the PI3-k/Akt, the protein kinase A (PKA), and the adenosine monophosphate-activated protein kinase (AMPK) signaling pathways in insulin target tissues (Kim et al. Citation2012; Onda et al. Citation2013; Zygmunt et al. Citation2010). The AMPK, PKA, and the PI3-k/Akt pathways are potential drug targets in the management of type 2 diabetes (Coughlan et al. Citation2014; Huang et al. Citation2018; Sayem et al. Citation2018; Yang and Yang Citation2016). AMPK is an intracellular energy-sensing enzyme, which increases glucose transport and GLUT4 translocation through an insulin-independent mechanism, while the PI3-k/Akt pathway is insulin-dependent (Coughlan et al. Citation2014; Sayem et al. Citation2018). Both AMPK and PI3-k/Akt pathway activation improves insulin sensitivity and metabolic health by stimulating intracellular glucose transport and metabolism in skeletal muscle, fatty acid oxidation in adipose tissues and reduces gluconeogenesis, and stimulates lipolysis in the liver (Coughlan et al. Citation2014; Huang et al. Citation2018; Sayem et al. Citation2018). Thus, the ability of naturally-occurring (poly)phenols to potentially modify these pathways beneficially can be considered an effective dietary strategy to control the development of type 2 diabetes.
Naringenin 9 stimulated glucose uptake in murine muscle cells (L6 myotubes) in a dose-dependent manner (10 − 150 μM) via activating the AMPK pathway (Zygmunt et al. Citation2010). Silencing of AMPK using small interference RNA (siRNA) prevented glucose uptake in the cells, further confirming the importance of the AMPK pathway in the naringenin 9-stimulated glucose uptake. At a concentration of 75 μM, naringenin 9 exhibited maximum glucose uptake, which was similar to the activity exerted by 100 nM insulin (Zygmunt et al. Citation2010). Several other studies have also reported the insulin-like effects of naringenin 9 in HepG2 cells (Allister et al. Citation2005; Borradaile, de Dreu, and Huff Citation2003) and rat adipocytes (Lim, Soh, and Kuppusamy Citation2008). In addition to its insulin-like effects, naringenin 9 significantly enhanced insulin-stimulated glucose uptake in muscle cells at low insulin levels (<10 nM) (Zygmunt et al. Citation2010). Likewise, naringenin 9 increased insulin-stimulated glucose uptake in TNF-α-stimulated insulin-resistant C2C12 myotubes through an AMPK-dependent mechanism (Li et al. Citation2019). Naringin 6 supplementation for 20 weeks at a level of 0.02% in the diet attenuated metabolic syndrome in C57BL/6J mice fed a high-fat diet by activating the AMPK pathway, and by inhibiting the mitogen-activated protein kinase (MAPK)-dependent signaling cascades, including ERK1/2, JNK1/2 and P38 pathways (Pu et al. Citation2012).
In differentiated C2C12 myotubes, a very high concentration of tangeretin 12 (>100 µM) stimulated AMPK activation, promoted GLUT4 translocation from cytosol to the cell membrane and glucose uptake without affecting PPAR-γ expression (Kim et al. Citation2012). In addition, tangeretin 12 (200 mg/kg diet) improved insulin resistance, reduced fat accumulation, and adipocytokine production, especially, leptin and resistin, and reduced the levels of the inflammatory markers MCP-1 and IL-6 in obese mice (Kim et al. Citation2012). Similarly, both tangeretin 12 and nobiletin 13 increased adiponectin secretion and decreased MCP-1 levels in 3T3-L1 adipocytes, whilst nobiletin 13 at a concentration of 128 µM reduced the level of resistin (Miyata et al. Citation2011). Adipocyte-derived cytokines, such as leptin and resistin, are important markers for the development of type 2 diabetes (Kocot et al. Citation2017). Resistin increases insulin resistance in peripheral tissues by affecting AMPK activity and also reduces insulin receptor substrate (IRS-1 and IRS-2) expression, thereby disrupting insulin signaling, leading to increased hepatic gluconeogenesis and reduced glycolysis. In 3T3-F442A adipocytes, tangeretin 12 and nobiletin 13 significantly enhanced glucose uptake from a concentration starting from 5 µM, mainly via activating the PI3-k/Akt and PKA pathways without affecting AMPK (Onda et al. Citation2013). According to the evaluated studies, high concentrations of citrus (poly)phenols appear to modulate glucose homeostasis mainly by affecting the AMPK pathway in muscle tissues, and the PI3-k/Akt pathway in adipose tissues (). However, the reported active concentrations are far above the physiological level.
Insulin sensitizing effect in adipocytes
Adipose tissue stores fat and is an endocrine organ that plays an important role in obesity-associated insulin resistance (Okabe et al. Citation2014). Mature healthy adipose tissues improve insulin resistance by secreting insulin-sensitizing factors, such as adiponectin and leptin. On the other hand, hypertrophied adipose tissues infiltrated by macrophages mainly secrete the insulin resistance factors, TNF-α, retinol-binding protein 4 (RBP4), IL-6, and IL-1β (Hardy, Czech, and Corvera Citation2012; Smith and Kahn Citation2016). Therefore, modulation of adipogenesis and adipocyte hypertrophy can be regarded as useful strategies in the prevention and/or control of obesity-associated insulin resistance. Citrus flavanones are reported to affect adipogenesis and adipocyte gene expression in vitro and in vivo (Ahmed et al. Citation2017; Akiyama et al. Citation2010; Liu et al. Citation2008; Vidal-Puig et al. Citation1997). Navel orange peel extract, naringin 6, and naringenin 9 were reported to significantly increase adiponectin, insulin receptor and GLUT4 mRNA expression in STZ/NA-induced type 2 diabetic rats compared to the diabetic control (Ahmed et al. Citation2017). Adiponectin secreted by adipocytes improves glucose and lipid homeostasis through its insulin-sensitizing properties. A lower level of adiponectin is directly associated with the development of type 2 diabetes and metabolic syndrome (Frankenberg, Reis, and Gerchman Citation2017; Liu et al. Citation2008). Hesperidin 1 and its aglycone hesperetin 8 significantly increased serum adiponectin levels to normalcy, comparable to the non-diabetic control (Akiyama et al. Citation2009). Liu et al. (Citation2008) reported significant up regulation of adiponectin expression in response to 80 µM hesperetin 8 and naringenin 9 in 3T3-L1 adipocytes, comparable to the commercial drug rosiglitazone, but this concentration is an order of magnitude above that achievable physiologically. Nobiletin 13 improved hyperglycemia and insulin resistance in diabetic ob/ob mice by increasing plasma and adipose adiponectin levels, and by up regulating the expression of GLUT1 and GLUT4 in both muscle and adipose tissues (Lee et al. Citation2010). Nobiletin 13 enhanced differentiation of ST-13 preadipocytes into mature adipocytes and increased the adiponectin level in 10 μM nobiletin 13-treated cells by 3.4-fold compared to the control (Kunimasa et al. Citation2009).
In contrast to reports on the beneficial effects of citrus (poly)phenols, several in vitro cell-based studies also report anti-insulin sensitizing effects using MDA-MB-231 breast cancer cells (Yang et al. Citation2013), MC3T3-G2/PA6 adipocytes (Nomura et al. Citation2008), U937 cells (Park Citation1999) and 3T3-L1 adipocytes (Claussnitzer et al. Citation2011; Harmon and Patel Citation2003; Kanda et al. Citation2012; Liu et al. Citation2008; Richard et al. Citation2013). A significant dose-dependent reduction in insulin-stimulated glucose uptake and IRS-1 tyrosine phosphorylation was reported in mature 3T3-L1 adipocytes in response to a high concentration of naringenin 9 (44, 92 and 183 µM) (Richard et al. Citation2013). Further, high concentrations of naringenin 9 also reduced adiponectin secretion in both human (92 µM naringenin 9) and murine adipocytes (183 µM naringenin 9) (Richard et al. Citation2013). Similarly, 20 µM naringenin 9 was found to significantly reduce basal and insulin-stimulated glucose uptake (∼20% inhibition) in both 3T3-L1 and mature human adipocytes (Claussnitzer et al. Citation2011). The inhibitory effect exerted by naringenin 9 was even prominent at a concentration as low as 1 µM (Claussnitzer et al. Citation2011). Several other studies have reported similar effects with naringenin 9 () and the anti-insulin sensitizing effect exhibited by naringenin 9 in adipocytes has been postulated to be due to impaired PI3K/Akt pathway and reduced trafficking of GLUT4 to the plasma membrane (Harmon and Patel Citation2003; Nomura et al. Citation2008; Richard et al. Citation2013). Claussnitzer et al. (Citation2011) also proposed a direct inhibitory effect of naringenin 9 on GLUT4. Likewise, hesperetin 8 was reported to downregulate the expression of GLUT1, inhibit insulin-induced translocation of GLUT4, and suppress phosphorylation of the insulin receptor beta subunit and Akt in MDA-MB-231 breast cancer cells (Yang et al. Citation2013).
In contrast to the reports on the lowering of insulin sensitivity by naringenin 9 on adipose tissue, Liu et al. (Citation2008) reported that both hesperetin 8 and naringenin 9 significantly increased adiponectin mRNA expression and increased PPAR-γ-controlled luciferase expression in a dose-dependent manner in 3T3-L1 adipocytes. Naringenin chalcone, an intermediate derivative of naringenin 9, was reported to enhance insulin-sensitizing effects in 3T3-L1 adipocytes by increasing the expression of adiponectin, both at a transcriptional and translational level (8- and 2.2-fold, respectively), without affecting preadipocyte differentiation up to a concentration of 100 µM (Horiba et al. Citation2010). Microarray studies revealed further up-regulation of several genes linked with energy metabolism (Horiba et al. Citation2010). At a concentration of 100 µM, naringenin 9 treatment led to increased glucose uptake (163%) in primary rat adipocytes, superior to insulin (Lim, Soh, and Kuppusamy Citation2008). Furthermore, naringenin 9 significantly increased glucose uptake in a dose-dependent manner (0.01 − 100 µM naringenin 9) independent of insulin (Lim, Soh, and Kuppusamy Citation2008). Naringenin 9 at >10 µM reduced Toll-like receptor 2 (TLR2) mRNA and protein expression in 3T3-L1 adipocyte during differentiation (Yoshida et al. Citation2013). Toll-like receptors are directly linked with the obesity-associated inflammatory response in adipose tissues, which long term can lead to insulin resistance and type 2 diabetes. The authors saw the same effect being translated in high-fat diet-fed C57BL/6J mice, where supplementation of the diet with 1% naringenin 9 for 16 weeks improved glycemic response and reduced the level of TNF-α, MCP-1, and TLR2 in the adipose tissues of obese mice (Yoshida et al. Citation2013).
In rodents, many studies report a favorable glycemic outcome upon long-term supplementation with citrus extract or pure compound, including naringenin 9 (). Naringenin 9 supplementation for 44 days at 0.05% of the diet increased insulin signaling and sensitivity in fructose-fed insulin-resistant rats (Kannappan and Anuradha Citation2010). Other studies with STZ-treated (Ren et al. Citation2016), STZ-nicotinamide treated (Annadurai et al. Citation2012), diabetic pregnant db/+ (Nguyen‐Ngo, Willcox, and Lappas Citation2019; Xing, Yang, and Wu Citation2016), OFA (Mayneris-Perxachs et al. Citation2019) and LDL-receptor null mice (Mulvihill et al. Citation2009) also demonstrated the positive effect of naringenin 9 on reducing the incidence of insulin resistance and type 2 diabetes (). Depending on the molecular structure and chemical properties, (poly)phenols are capable of modulating the activity or expression of various genes/proteins, and the outcome will depend on the cumulative effect. When the beneficial effects of a compound or a group of compounds outweigh any opposing effects, improvements in the biomarkers of interest are seen. Thus, although cell culture studies sometimes show a negative effect of naringenin 9 on insulin resistance, as a whole, citrus extract and its compounds seem to improve glucose homeostasis, especially in vivo.
Study limitations
The most important point to note is that the concentrations of the (poly)phenols used in most of the in vitro studies reported here are extremely high. Out of the 33 studies listed in on the potential post-absorptive mechanisms, 23 studies have employed physiologically unrealistic (poly)phenol treatments to show an effect. In general, (poly)phenols or their metabolites reach the circulation or target tissues in low concentrations, certainly considerably below 10 µM. According to bioavailability data, the maximum plasma concentration attained for hesperetin 8 and naringenin 9 metabolites range from 0.07 − 2.2 µM and 0.04 − 6 µM (Actis-Goretta et al. Citation2015; Erlund et al. Citation2001; Vallejo et al. Citation2010), respectively, while in vitro studies have used concentrations ranging from 1 µM to >300 µM, with significant effects generally only seen at concentrations toward the higher end (). Since the amount of (poly)phenols found within the gut is generally at least several-fold higher than present in the circulation, the use of higher concentrations in studies looking at effects within the gut is acceptable to a certain extent. Generally, 300 mL of orange juice delivers >300 µmol and >50 µmol of hesperidin 1 and naringenin 9, respectively (Aschoff et al. Citation2015; Gil-Izquierdo et al. Citation2001). Assuming the bioaccessibility of these compounds to be 100%, they will be found in high concentrations in the lumen. On the other hand, studies done on non-gut cells/enzymes which focus on post-absorptive effects should utilize physiologically applicable concentrations, usually of the same order as the maximum plasma concentration (Cmax). Unfortunately, the majority of the studies on non-gut cells have employed non-physiological doses of (poly)phenols (), bringing into question the physiological and clinical relevance in humans.
Further, some studies have also utilized flavanone glycosides in non-gut cells. Use of the aglycones is acceptable to a certain extent, but not the glycosides. To obtain meaningful results, instead of the aglycones, physiologically relevant flavanone conjugates (14–26) and their microbial catabolites should be utilized, as the bioactivity exerted by the metabolites could be different from the parent compound and/or the aglycones. Thus, to identify the potential effects and mechanisms, future studies should emphasize employing physiologically relevant concentrations (<10 µM) of the flavanone metabolites/catabolites.
Overall summary and future recommendations
This paper critically reviews studies reporting the mechanism of effects of citrus (poly)phenols on modulating the risk of type 2 diabetes. Over the past two decades, many studies have focused on identifying the effect of citrus (poly)phenols, especially hesperidin 1/hesperetin 8, narirutin 5/naringin 6/naringenin 9, and the polymethoxyflavones, tangeretin 12, and nobiletin 13 on markers of type 2 diabetes risk and their potential mechanisms of action. Despite numerous studies, shortcomings in study designs make conclusive interpretations difficult. With regard to in vitro studies, although they indicate a possible beneficial effect through identified and proposed mechanisms, about 70% of the studies listed in have utilized physiologically unrealistic concentrations and/or forms of the compounds. Except for studies focusing on the mechanisms within the gut (starch hydrolyzing enzymes and glucose transporters), studies conducted with non-gut cells and enzymes have utilized very high doses, and the concentrations required to see an effect are far above the physiological concentrations. Another point to note is the inappropriate use of compounds in the studies, without considering physiological relevance; there is no point in treating liver cells with hesperidin 1 for example since after consumption, hesperidin 1 is deglycosylated in the gut before absorption. This means that hesperetin 8 and conjugates are found in the blood, and are the chemical species that will encounter liver cells, but not the parent hesperidin. Thus, studies targeting the intestinal lumen should focus on using the flavanone glycosides instead of the aglycones, as these compounds are less likely to be hydrolyzed in the small intestine, and studies focused on post-absorptive mechanisms should utilize the aglycones or their respective metabolites. According to bioavailability studies, the majority of the citrus flavanones undergo Phase II metabolism and the methylated, sulfated, or glucuronidated metabolites are the primary compounds found in the circulation. Going forward, studies should focus more on using the flavanone metabolites and especially the microbiota-derived phenolic catabolites over the aglycones, as the latter is usually at low concentration in the circulation. Hence, future in vitro studies probing the antidiabetic mechanism of citrus (poly)phenols should pay more attention to using physiologically relevant doses and focus on using the appropriate compounds or their metabolites in the experiments, as the bioactivity and efficacy of these compounds depend on their chemical and structural nature.
Overall, the in vitro and animal studies demonstrate a potential beneficial effect of citrus (poly)phenols on the markers of type 2 diabetes, predominantly through post-absorptive mechanisms, mainly by regulating hepatic glucose homeostasis and insulin sensitivity. Within the gut, the flavanones appear to moderately inhibit the glucose transporters, with little effect on starch digesting enzymes. Furthermore, based on the outcomes from animal studies, the beneficial effects derived from consuming citrus compounds appear to be more evident in long-term studies as compared to acute treatments.
Abbreviations | ||
ADP | = | Adenosine diphosphate |
Akt | = | Protein kinase B |
AMPK | = | 5’ adenosine monophosphate-activated protein kinase |
apoB | = | Apolipoprotein B |
bw | = | Body weight |
CD | = | Clathrate |
CGO | = | Citrus grandis Osbeck |
ChREBP | = | Carbohydrate response element-binding protein |
Cmax | = | Maximum plasma concentration |
DMSO | = | Dimethyl sulfoxide |
DPP-4 | = | Dipeptidyl-peptidase 4 |
EGCG | = | Epigallocatechin gallate |
ERK1/2 | = | Extracellular signal-regulated protein kinase |
F16BP | = | Fructose-1,6-bisphosphatase |
FBG | = | Fasting blood glucose |
FBI | = | Fasting blood insulin |
G6P | = | Glucose-6-phosphatase |
GDM | = | Gestational diabetes mellitus |
GIP | = | Glucose-dependent insulinotropic polypeptide |
GK | = | Glucokinase |
GLP-1 | = | Glucagon-like-peptide-1 |
GLUT1 | = | Glucose transporter 1 |
GLUT2 | = | Glucose transporter 2 |
GLUT4 | = | Glucose transporter 4 |
GLUT5 | = | Glucose transporter 5 |
GSK-3 | = | Glycogen synthase kinase-3 |
HbA1c | = | Glycosylated hemoglobin |
HMG-CoA | = | 3-hydroxy-3-methylglutaryl coenzyme A |
HOMA-IR | = | Homeostatic model assessment of insulin resistance |
IFN-γ | = | Interferon-gamma |
IL-10 | = | Interleukin 10 |
IL-1β | = | Interleukin 1 beta |
IL-6 | = | Interleukin 6 |
iNOS | = | Inducible nitric oxide synthase |
Ins1/2 | = | Insulin |
IRS-1/2 | = | Insulin receptor substrate |
JNK1/2 | = | c-Jun N-terminal kinase |
LDL | = | Low-density lipoprotein |
MAPK | = | Mitogen-activated protein kinase |
MCP-1 | = | Monocyte chemoattractant protein |
miR-140 | = | MicroRNA-140 |
mRNA | = | Messenger ribonucleic acid |
MTP | = | Microsomal triglyceride transfer protein |
NF-κB | = | Nuclear factor Kappa B |
PEPCK | = | Phosphoenolpyruvate carboxykinase |
PI3-K | = | Phosphatidylinositol 3 kinase |
PKA | = | Protein kinase A |
PMF | = | Polymethoxyflavone |
PPARα | = | Peroxisome proliferator-activated receptor alpha |
PPAR-γ | = | Peroxisome proliferator-activated receptor gamma |
PTP1B | = | Protein tyrosine phosphatase 1B |
RBP4 | = | Retinol-binding protein 4 |
SGLT1 | = | Sodium-dependent glucose cotransporter |
siRNA | = | Small interference RNA |
SREBP-1 | = | Sterol regulatory element-binding protein |
STZ | = | Streptozotocin |
STZ/NA | = | Streptozotocin-nicotinamide |
TC | = | Total cholesterol |
TG | = | Triglyceride |
TLR-2 | = | Toll-like receptor 2 |
TNF-α | = | Tumor necrosis factor-alpha. |
Acknowledgements
We thank Monash University for an International PhD Scholarship to RV.
Disclosure statement
The authors declare no conflicts of interest.
References
- Abad-García, B., S. Garmón-Lobato, L. A. Berrueta, B. Gallo, and F. Vicente. 2012. On line characterization of 58 phenolic compounds in citrus fruit juices from Spanish cultivars by high-performance liquid chromatography with photodiode-array detection coupled to electrospray ionization triple quadrupole mass spectrometry. Talanta 99:213–24. doi: 10.1016/j.talanta.2012.05.042.
- Abbas, M., F. Saeed, F. M. Anjum, M. Afzaal, T. Tufail, M. S. Bashir, A. Ishtiaq, S. Hussain, and H. A. R. Suleria. 2017. Natural polyphenols: An overview. International Journal of Food Properties 20 (8):1689–99. doi: 10.1080/10942912.2016.1220393.
- Actis-Goretta, L., T. P. Dew, A. Lévèques, G. Pereira-Caro, M. Rein, A. Teml, C. Schäfer, U. Hofmann, M. Schwab, M. Eichelbaum, et al. 2015. Gastrointestinal absorption and metabolism of hesperetin-7-O-rutinoside and hesperetin-7-O-glucoside in healthy humans. Molecular Nutrition & Food Research 59 (9):1651–62. doi: 10.1002/mnfr.201500202.
- Ahmed, O. M., M. A. Hassan, S. M. Abdel-Twab, and M. N. Abdel Azeem. 2017. Navel orange peel hydroethanolic extract, naringin and naringenin have anti-diabetic potentials in type 2 diabetic rats. Biomedicine & Pharmacotherapy = Biomedecine & Pharmacotherapie 94:197–205. doi: 10.1016/j.biopha.2017.07.094.
- Aja, P. M., F. I. Izekwe, A. C. Famurewa, E. U. Ekpono, F. E. Nwite, I. O. Igwenyi, J. N. Awoke, O. G. Ani, C. Aloke, N. A. Obasi, et al. 2020. Hesperidin protects against cadmium-induced pancreatitis by modulating insulin secretion, redox imbalance and inos/nf-ĸb signaling in rats. Life Sciences 259:118268. doi: 10.1016/j.lfs.2020.118268.
- Akiyama, S., S. I. Katsumata, K. Suzuki, Y. Ishimi, J. Wu, and M. Uehara. 2010. Dietary hesperidin exerts hypoglycemic and hypolipidemic effects in streptozotocin-induced marginal type 1 diabetic rats. Journal of Clinical Biochemistry and Nutrition 46 (1):87–92. doi: 10.3164/jcbn.09-82.
- Akiyama, S., S.-I. Katsumata, K. Suzuki, Y. Nakaya, Y. Ishimi, and M. Uehara. 2009. Hypoglycemic and hypolipidemic effects of hesperidin and cyclodextrin-clathrated hesperetin in goto-kakizaki rats with type 2 diabetes. Bioscience, Biotechnology, and Biochemistry 73 (12):2779–82. doi: 10.1271/bbb.90576.
- Alam, M. A., N. Subhan, M. M. Rahman, S. J. Uddin, H. M. Reza, and S. D. Sarker. 2014. Effect of citrus flavonoids, naringin and naringenin, on metabolic syndrome and their mechanisms of action. Advances in Nutrition (Bethesda, Md.) 5 (4):404–17. doi: 10.3945/an.113.005603.
- Ali, M. Y., S. Zaib, M. M. Rahman, S. Jannat, J. Iqbal, S. K. Park, and M. S. Chang. 2019. Didymin, a dietary citrus flavonoid exhibits anti-diabetic complications and promotes glucose uptake through the activation of pi3k/akt signaling pathway in insulin-resistant hepg2 cells. Chemico-Biological Interactions 305:180–94. doi: 10.1016/j.cbi.2019.03.018.
- Allister, E. M., N. M. Borradaile, J. Y. Edwards, and M. W. Huff. 2005. Inhibition of microsomal triglyceride transfer protein expression and apolipoprotein b100 secretion by the citrus flavonoid naringenin and by insulin involves activation of the mitogen-activated protein kinase pathway in hepatocytes. Diabetes 54 (6):1676–83. doi: 10.2337/diabetes.54.6.1676.
- Alu’datt, M. H., T. Rababah, M. N. Alhamad, M. A. Al-Mahasneh, K. Ereifej, G. Al-Karaki, M. Al-Duais, J. E. Andrade, C. C. Tranchant, S. Kubow, et al. 2017. Profiles of free and bound phenolics extracted from citrus fruits and their roles in biological systems: Content, and antioxidant, anti-diabetic and anti-hypertensive properties. Food & Function 8 (9):3187–97. doi: 10.1039/C7FO00212B.
- Annadurai, T., A. R. Muralidharan, T. Joseph, M. J. Hsu, P. A. Thomas, and P. Geraldine. 2012. Antihyperglycemic and antioxidant effects of a flavanone, naringenin, in streptozotocin-nicotinamide-induced experimental diabetic rats. Journal of Physiology and Biochemistry 68 (3):307–18. doi: 10.1007/s13105-011-0142-y.
- Aronoff, S. L., K. Berkowitz, B. Shreiner, and L. Want. 2004. Glucose metabolism and regulation: Beyond insulin and glucagon. Diabetes Spectrum 17 (3):183–90. doi: 10.2337/diaspect.17.3.183.
- Aschoff, J. K., S. Kaufmann, O. Kalkan, S. Neidhart, R. Carle, and R. M. Schweiggert. 2015. In vitro bioaccessibility of carotenoids, flavonoids, and vitamin c from differently processed oranges and orange juices [Citrus sinensis (l.) Osbeck]. Journal of Agricultural and Food Chemistry 63 (2):578–87. doi: 10.1021/jf505297t.
- Ballistreri, G., S. Fabroni, F. V. Romeo, N. Timpanaro, M. Amenta, and P. Rapisarda. 2019. Anthocyanins and other polyphenols in citrus genus: Biosynthesis, chemical profile, and biological activity. In Polyphenols in plants, ed. R. R. Watson, 2nd Edition, 191–215. London: Academic Press. doi: 10.1016/B978-0-12-813768-0.00014-1.
- Bhattacharya, S., N. Oksbjerg, J. F. Young, and P. B. Jeppesen. 2014. Caffeic acid, naringenin and quercetin enhance glucose-stimulated insulin secretion and glucose sensitivity in ins-1e cells. Diabetes, Obesity & Metabolism 16 (7):602–12. doi: 10.1111/dom.12236.
- Bhupathiraju, S. N., D. K. Tobias, V. S. Malik, A. Pan, A. Hruby, J. E. Manson, W. C. Willett, and F. B. Hu. 2014. Glycemic index, glycemic load, and risk of type 2 diabetes: Results from 3 large us cohorts and an updated meta-analysis. The American Journal of Clinical Nutrition 100 (1):218–32. doi: 10.3945/ajcn.113.079533.
- Borges, G., M. E. J. Lean, S. A. Roberts, and A. Crozier. 2013. Bioavailability of dietary (poly)phenols: A study with ileostomists to discriminate between absorption in small and large intestine. Food & Function 4 (5):754–62. doi: 10.1039/c3fo60024f.
- Borges, G., W. Mullen, A. Mullan, M. E. J. Lean, S. A. Roberts, and A. Crozier. 2010. Bioavailability of multiple components following acute ingestion of a polyphenol-rich juice drink. Molecular Nutrition & Food Research 54 (S2):S268–S77. doi: 10.1002/mnfr.200900611.
- Borradaile, N. M., L. E. de Dreu, and M. W. Huff. 2003. Inhibition of net hepg2 cell apolipoprotein b secretion by the citrus flavonoid naringenin involves activation of phosphatidylinositol 3-kinase, independent of insulin receptor substrate-1 phosphorylation. Diabetes 52 (10):2554–61. doi: 10.2337/diabetes.52.10.2554.
- Brett, G. M., W. Hollands, P. W. Needs, B. Teucher, J. R. Dainty, B. D. Davis, J. S. Brodbelt, and P. A. Kroon. 2009. Absorption, metabolism and excretion of flavanones from single portions of orange fruit and juice and effects of anthropometric variables and contraceptive pill use on flavanone excretion. British Journal of Nutrition 101 (5):664–75. doi: 10.1017/S000711450803081X.
- Butterworth, P. J., F. J. Warren, and P. R. Ellis. 2011. Human α-amylase and starch digestion: An interesting marriage. Starch - Stärke 63 (7):395–405. doi: 10.1002/star.201000150.
- Cantley, J., and F. M. Ashcroft. 2015. Q&A: Insulin secretion and type 2 diabetes: why do β-cells fail?BMC Biology 13 (1):33. doi: 10.1186/s12915-015-0140-6.
- Cao, H., J. Ou, L. Chen, Y. Zhang, T. Szkudelski, D. Delmas, M. Daglia, and J. Xiao. 2019. Dietary polyphenols and type 2 diabetes: Human study and clinical trial. Critical Reviews in Food Science and Nutrition 59 (20):3371–9. doi: 10.1080/10408398.2018.1492900.
- Cao, R., Y. Zhao, Z. Zhou, and X. Zhao. 2018. Enhancement of the water solubility and antioxidant activity of hesperidin by chitooligosaccharide. Journal of the Science of Food and Agriculture 98 (6):2422–7. doi: 10.1002/jsfa.8734.
- Casacchia, T., M. A. Occhiuzzi, F. Grande, B. Rizzuti, M. C. Granieri, C. Rocca, A. Gattuso, A. Garofalo, T. Angelone, and G. Statti. 2019. A pilot study on the nutraceutical properties of the citrus hybrid tacle® as a dietary source of polyphenols for supplementation in metabolic disorders. Journal of Functional Foods 52:370–81. doi: 10.1016/j.jff.2018.11.030.
- Cerletti, C., F. Gianfagna, C. Tamburrelli, A. De Curtis, M. D’Imperio, W. Coletta, L. Giordano, R. Lorenzet, P. Rapisarda, G. Reforgiato Recupero, et al. 2015. Orange juice intake during a fatty meal consumption reduces the postprandial low-grade inflammatory response in healthy subjects. Thrombosis Research 135 (2):255–9. doi: 10.1016/j.thromres.2014.11.038.
- Chadt, A., and H. Al-Hasani. 2020. Glucose transporters in adipose tissue, liver, and skeletal muscle in metabolic health and disease. Pflugers Archiv: European Journal of Physiology 472 (9):1273–98. doi: 10.1007/s00424-020-02417-x.
- Chudnovskiy, R., A. Thompson, K. Tharp, M. Hellerstein, J. L. Napoli, and A. Stahl. 2014. Consumption of clarified grapefruit juice ameliorates high-fat diet induced insulin resistance and weight gain in mice. Ed. Makoto Makishima. PLoS One 9 (10):e108408. doi: 10.1371/journal.pone.0108408.
- Claussnitzer, M., T. Skurk, H. Hauner, H. Daniel, and M. J. Rist. 2011. Effect of flavonoids on basal and insulin-stimulated 2-deoxyglucose uptake in adipocytes. Molecular Nutrition & Food Research 55 (S1):S26–S34. doi: 10.1002/mnfr.201000372.
- Constantin, R. P., R. P. Constantin, A. Bracht, N. S. Yamamoto, E. L. Ishii-Iwamoto, and J. Constantin. 2014. Molecular mechanisms of citrus flavanones on hepatic gluconeogenesis. Fitoterapia 92:148–62. doi: 10.1016/j.fitote.2013.11.003.
- Coughlan, K., R. Valentine, N. Ruderman, and A. Saha. 2014. AMPK activation: A therapeutic target for type 2 diabetes?Diabetes, Metabolic Syndrome and Obesity: Targets and Therapy 7:241–53. doi: 10.2147/DMSO.S43731.
- Deacon, C. F. 2019. Physiology and pharmacology of dpp-4 in glucose homeostasis and the treatment of type 2 diabetes. Frontiers in Endocrinology 10:80. doi: 10.3389/fendo.2019.00080.
- De Ancos, B., A. Cilla, R. Barberá, C. Sánchez-Moreno, and M. P. Cano. 2017. Influence of orange cultivar and mandarin postharvest storage on polyphenols, ascorbic acid and antioxidant activity during gastrointestinal digestion. Food Chemistry 225:114–24. doi: 10.1016/j.foodchem.2016.12.098.
- de la Garza, A. L., U. Etxeberria, M. P. Lostao, B. San Román, J. Barrenetxe, J. A. Martínez, and F. I. Milagro. 2013. Helichrysum and grapefruit extracts inhibit carbohydrate digestion and absorption, improving postprandial glucose levels and hyperinsulinemia in rats. Journal of Agricultural and Food Chemistry 61 (49):12012–9. doi: 10.1021/jf4021569.
- de Paiva, A., D. Gonçalves, P. Ferreira, E. Baldwin, and T. Cesar. 2019. Postprandial effect of fresh and processed orange juice on the glucose metabolism, antioxidant activity and prospective food intake. Journal of Functional Foods 52:302–9. doi: 10.1016/j.jff.2018.11.013.
- Díaz-García, M. C., J. M. Obón, M. R. Castellar, J. Collado, and M. Alacid. 2013. Quantification by UHPLC of total individual polyphenols in fruit juices. Food Chemistry 138 (2-3):938–49. doi: 10.1016/j.foodchem.2012.11.061.
- do Nascimento, G. S., R. P. Constantin, E. H. Gilglioni, C. V. de Castro Ghizoni, A. Bracht, K. S. Utsunomiya, N. S. Yamamoto, E. L. Ishii-Iwamoto, J. Constantin, and R. P. Constantin. 2018. The acute effects of citrus flavanones on the metabolism of glycogen and monosaccharides in the isolated perfused rat liver. Toxicology Letters 291:158–72. doi: 10.1016/j.toxlet.2018.04.001.
- Domínguez-Avila, J. A., A. Wall-Medrano, G. R. Velderrain-Rodríguez, C.-Y. O. Chen, N. J. Salazar-López, M. Robles-Sánchez, and G. A. González-Aguilar. 2017. Gastrointestinal interactions, absorption, splanchnic metabolism and pharmacokinetics of orally ingested phenolic compounds. Food & Function 8 (1):15–38. doi: 10.1039/C6FO01475E.
- Dong, H., C. Rendeiro, A. Kristek, L. J. Sargent, C. Saunders, L. Harkness, I. Rowland, K. G. Jackson, J. P. E. P. Spencer, and J. A. Lovegrove. 2016. Addition of orange pomace to orange juice attenuates the increases in peak glucose and insulin concentrations after sequential meal ingestion in men with elevated cardiometabolic risk. The Journal of Nutrition 146 (6):1197–203. doi: 10.3945/jn.115.226001.
- Erlund, I., E. Meririnne, G. Alfthan, and A. Aro. 2001. Plasma kinetics and urinary excretion of the flavanones naringenin and hesperetin in humans after ingestion of orange juice and grapefruit juice. The Journal of Nutrition 131 (2):235–41. doi: 10.1093/jn/131.2.235.
- Fallico, B., G. Ballistreri, E. Arena, S. Brighina, and P. Rapisarda. 2017. Bioactive compounds in blood oranges (Citrus sinensis (l.) Osbeck): Level and intake. Food Chemistry 215:67–75. doi: 10.1016/j.foodchem.2016.07.142.
- Fan, J., M. H. Johnson, M. A. Lila, G. Yousef, and E. G. de Mejia. 2013. Berry and citrus phenolic compounds inhibit dipeptidyl peptidase iv: Implications in diabetes management. Evidence-Based Complementary and Alternative Medicine: eCAM 2013:479505–13. doi: 10.1155/2013/479505.
- Fei, Q., Y. Gao, X. Zhang, Y. Sun, B. Hu, L. Zhou, S. Jabbar, and X. Zeng. 2014. Effects of Oolong tea polyphenols, EGCG, and EGCG3″me on pancreatic α-amylase activity in vitro. Journal of Agricultural and Food Chemistry 62 (39):9507–14. doi: 10.1021/jf5032907.
- Frankenberg, A. D v., A. F. Reis, and F. Gerchman. 2017. Relationships between adiponectin levels, the metabolic syndrome, and type 2 diabetes: A literature review. Archives of Endocrinology and Metabolism 61 (6):614–22. doi: 10.1590/2359-3997000000316.
- Fu, Z., E. R. Gilbert, and D. Liu. 2012. Regulation of insulin synthesis and secretion and pancreatic beta-cell dysfunction in diabetes. Current Diabetes Reviews 9 (1):25–53. doi: 10.2174/15733998130104.
- Fujioka, K., F. Greenway, J. Sheard, and Y. Ying. 2006. The effects of grapefruit on weight and insulin resistance: Relationship to the metabolic syndrome. Journal of Medicinal Food 9 (1):49–54. doi: 10.1089/jmf.2006.9.49.
- Gamo, K., H. Miyachi, K. Nakamura, and N. Matsuura. 2014. Hesperetin glucuronides induce adipocyte differentiation via activation and expression of peroxisome proliferator-activated receptor-γ. Bioscience, Biotechnology, and Biochemistry 78 (6):1052–9. doi: 10.1080/09168451.2014.910097.
- Gao, Z., W. Gao, S.-L. Zeng, P. Li, and E.-H. Liu. 2018. Chemical structures, bioactivities and molecular mechanisms of citrus polymethoxyflavones. Journal of Functional Foods 40:498–509. doi: 10.1016/j.jff.2017.11.036.
- Gattuso, G., D. Barreca, C. Gargiulli, U. Leuzzi, and C. Caristi. 2007. Flavonoid composition of citrus juices. Molecules (Basel, Switzerland) 12 (8):1641–73. doi: 10.3390/12081641.
- Gee, J., and I. Johnson. 2001. Polyphenolic compounds: Interactions with the gut and implications for human health. Current Medicinal Chemistry 8 (11):1245–55. doi: 10.2174/0929867013372256.
- Gil-Izquierdo, A., M. I. Gil, F. Ferreres, and F. A. Tomás-Barberán. 2001. In vitro availability of flavonoids and other phenolics in orange juice. Journal of Agricultural and Food Chemistry 49 (2):1035–41. doi: 10.1021/jf0000528.
- Gong, Y., X.-Y. Qin, Y.-Y. Zhai, H. Hao, J. Lee, and Y.-D. Park. 2017. Inhibitory effect of hesperetin on α-glucosidase: Molecular dynamics simulation integrating inhibition kinetics. International Journal of Biological Macromolecules 101:32–9. doi: 10.1016/j.ijbiomac.2017.03.072.
- Goodman, B. E. 2010. Insights into digestion and absorption of major nutrients in humans. Advances in Physiology Education 34 (2):44–53. doi: 10.1152/advan.00094.2009.
- Greenwood, D. C., D. E. Threapleton, C. E. L. Evans, C. L. Cleghorn, C. Nykjaer, C. Woodhead, and V. J. Burley. 2013. Glycemic index, glycemic load, carbohydrates, and type 2 diabetes: Systematic review and dose-response meta-analysis of prospective studies. Diabetes Care 36 (12):4166–71. doi: 10.2337/dc13-0325.
- Guo, J., H. Tao, Y. Cao, C.-T. Ho, S. Jin, and Q. Huang. 2016. Prevention of obesity and type 2 diabetes with aged citrus peel (Chenpi) extract. Journal of Agricultural and Food Chemistry 64 (10):2053–61. doi: 10.1021/acs.jafc.5b06157.
- Gupta, A., G. A. Jacobson, J. R. Burgess, H. F. Jelinek, D. S. Nichols, C. K. Narkowicz, and H. A. Al-Aubaidy. 2018. Citrus bioflavonoids dipeptidyl peptidase-4 inhibition compared with gliptin antidiabetic medications. Biochemical and Biophysical Research Communications 503 (1):21–5. doi: 10.1016/j.bbrc.2018.04.156.
- Hardy, O. T., M. P. Czech, and S. Corvera. 2012. What causes the insulin resistance underlying obesity?Current Opinion in Endocrinology, Diabetes, and Obesity 19 (2):81–7. doi: 10.1097/MED.0b013e3283514e13.
- Harmon, A. W., and Y. M. Patel. 2003. Naringenin inhibits phosphoinositide 3-kinase activity and glucose uptake in 3T3-L1 adipocytes. Biochemical and Biophysical Research Communications 305 (2):229–34. doi: 10.1016/S0006-291X(03)00720-4.
- Hayanga, J. A., S. P. Ngubane, A. N. Murunga, and P. M. O. Owira. 2016. Grapefruit juice improves glucose intolerance in streptozotocin-induced diabetes by suppressing hepatic gluconeogenesis. European Journal of Nutrition 55 (2):631–8. doi: 10.1007/s00394-015-0883-4.
- Horiba, T., I. Nishimura, Y. Nakai, K. Abe, and R. Sato. 2010. Naringenin chalcone improves adipocyte functions by enhancing adiponectin production. Molecular and Cellular Endocrinology 323 (2):208–14. doi: 10.1016/j.mce.2010.03.020.
- Huang, X., G. Liu, J. Guo, and Z. Su. 2018. The PI3K/AKT pathway in obesity and type 2 diabetes. International Journal of Biological Sciences 14 (11):1483–96. doi: 10.7150/ijbs.27173.
- Hwang, J.-T., H. J. Yang, K.-C. Ha, B.-O. So, E.-K. Choi, and S.-W. Chae. 2015. A randomized, double-blind, placebo-controlled clinical trial to investigate the anti-diabetic effect of Citrus junos Tanaka peel. Journal of Functional Foods 18:532–7. doi: 10.1016/j.jff.2015.08.019.
- Johnston, K., P. Sharp, M. Clifford, and L. Morgan. 2005. Dietary polyphenols decrease glucose uptake by human intestinal Caco-2 cells. FEBS Letters 579 (7):1653–7. doi: 10.1016/j.febslet.2004.12.099.
- Jones, A. G., and A. T. Hattersley. 2013. The clinical utility of c-peptide measurement in the care of patients with diabetes. Diabetic Medicine: A Journal of the British Diabetic Association 30 (7):803–17. doi: 10.1111/dme.12159.
- Jung, U. J., M.-K. Lee, K.-S. Jeong, and M.-S. Choi. 2004. The hypoglycemic effects of hesperidin and naringin are partly mediated by hepatic glucose-regulating enzymes in C57BL/KsJ-db/db mice. The Journal of Nutrition 134 (10):2499–503. doi: 10.1093/jn/134.10.2499.
- Jung, U. J., M.-K. Lee, Y. B. Park, M. A. Kang, and M.-S. Choi. 2006. Effect of citrus flavonoids on lipid metabolism and glucose-regulating enzyme mRNA levels in type-2 diabetic mice. The International Journal of Biochemistry & Cell Biology 38 (7):1134–45. doi: 10.1016/j.biocel.2005.12.002.
- Kalt, W., A. Cassidy, L. R. Howard, R. Krikorian, A. J. Stull, F. Tremblay, and R. Zamora-Ros. 2020. Recent research on the health benefits of blueberries and their anthocyanins. Advances in Nutrition (Bethesda, Md.) 11 (2):224–36. doi: 10.1093/advances/nmz065.
- Kanda, K., K. Nishi, A. Kadota, S. Nishimoto, M. C. Liu, and T. Sugahara. 2012. Nobiletin suppresses adipocyte differentiation of 3T3-L1 cells by an insulin and ibmx mixture induction. Biochimica et Biophysica Acta 1820 (4):461–8. doi: 10.1016/j.bbagen.2011.11.015.
- Kannappan, S., and C. V. Anuradha. 2010. Naringenin enhances insulin-stimulated tyrosine phosphorylation and improves the cellular actions of insulin in a dietary model of metabolic syndrome. European Journal of Nutrition 49 (2):101–9. doi: 10.1007/s00394-009-0054-6.
- Kelebek, H., S. Selli, A. Canbas, and T. Cabaroglu. 2009. HPLC determination of organic acids, sugars, phenolic compositions and antioxidant capacity of orange juice and orange wine made from a Turkish cv. Microchemical Journal 91 (2):187–92. doi: 10.1016/j.microc.2008.10.008.
- Kerimi, A., J. S. Gauer, S. Crabbe, J. W. Cheah, J. Lau, R. Walsh, P. F. Cancalon, and G. Williamson. 2019. Effect of the flavonoid hesperidin on glucose and fructose transport, sucrase activity and glycaemic response to orange juice in a crossover trial on healthy volunteers. The British Journal of Nutrition 121 (7):782–92. doi: 10.1017/S0007114519000084.
- Kerimi, A., H. Nyambe-Silavwe, A. Pyner, E. Oladele, J. S. Gauer, Y. Stevens, and G. Williamson. 2019. Nutritional implications of olives and sugar: Attenuation of post-prandial glucose spikes in healthy volunteers by inhibition of sucrose hydrolysis and glucose transport by oleuropein. European Journal of Nutrition 58 (3):1315–6. doi: 10.1007/s00394-018-1662-9.
- Khan, M. K., Zill-E-Huma, and O. Dangles. 2014. A comprehensive review on flavanones, the major citrus polyphenols. Journal of Food Composition and Analysis 33 (1):85–104. doi: 10.1016/j.jfca.2013.11.004.
- Kim, G.-N., J.-G. Shin, and H.-D. Jang. 2009. Antioxidant and antidiabetic activity of dangyuja (Citrus grandis Osbeck) extract treated with aspergillus saitoi. Food Chemistry 117 (1):35–41. doi: 10.1016/j.foodchem.2009.03.072.
- Kim, H.-i., and Y.-h. Ahn. 2004. Role of peroxisome proliferator-activated receptor- in the glucose-sensing apparatus of liver and -cells. Diabetes 53 (Supplement 1):S60–S65. doi: 10.2337/diabetes.53.2007.S60.
- Kim, M. S., H. J. Hur, D. Y. Kwon, and J.-T. Hwang. 2012. Tangeretin stimulates glucose uptake via regulation of AMPK signaling pathways in C2C12 myotubes and improves glucose tolerance in high-fat diet-induced obese mice. Molecular and Cellular Endocrinology 358 (1):127–34. doi: 10.1016/j.mce.2012.03.013.
- Kocot, J., P. Dziemidok, M. Kiełczykowska, A. Hordyjewska, G. Szcześniak, and I. Musik. 2017. Adipokine profile in patients with type 2 diabetes depends on degree of obesity. Medical Science Monitor: International Medical Journal of Experimental and Clinical Research 23:4995–5004. doi: 10.12659/MSM.904318.
- Kunimasa, K., S. Kuranuki, N. Matsuura, N. Iwasaki, M. Ikeda, A. Ito, Y. Sashida, Y. Mimaki, M. Yano, M. Sato, et al. 2009. Identification of nobiletin, a polymethoxyflavonoid, as an enhancer of adiponectin secretion. Bioorganic & Medicinal Chemistry Letters 19 (7):2062–4. doi: 10.1016/j.bmcl.2009.02.002.
- Kwon, O., P. Eck, S. Chen, C. P. Corpe, J. Lee, M. Kruhlak, and M. Levine. 2007. Inhibition of the intestinal glucose transporter GLUT2 by flavonoids. FASEB Journal: Official Publication of the Federation of American Societies for Experimental Biology 21 (2):366–77. doi: 10.1096/fj.06-6620com.
- Lee, Y.-S., B.-Y. Cha, K. Saito, H. Yamakawa, S.-S. Choi, K. Yamaguchi, T. Yonezawa, T. Teruya, K. Nagai, and J.-T. Woo. 2010. Nobiletin improves hyperglycemia and insulin resistance in obese diabetic ob/ob mice. Biochemical Pharmacology 79 (11):1674–83. doi: 10.1016/j.bcp.2010.01.034.
- Li, J. M., C. T. Che, C. B. S. Lau, P. S. Leung, and C. H. K. Cheng. 2006. Inhibition of intestinal and renal Na+-glucose cotransporter by naringenin. The International Journal of Biochemistry & Cell Biology 38 (5-6):985–95. doi: 10.1016/j.biocel.2005.10.002.
- Li, R. W., A. G. Theriault, K. Au, T. D. Douglas, A. Casaschi, E. M. Kurowska, and R. Mukherjee. 2006. Citrus polymethoxylated flavones improve lipid and glucose homeostasis and modulate adipocytokines in fructose-induced insulin resistant hamsters. Life Sciences 79 (4):365–73. doi: 10.1016/j.lfs.2006.01.023.
- Li, S., Y. Zhang, Y. Sun, G. Zhang, J. Bai, J. Guo, X. Su, H. Du, X. Cao, J. Yang, et al. 2019. Naringenin improves insulin sensitivity in gestational diabetes mellitus mice through AMPK. Nutrition & Diabetes 9 (1):28. doi: 10.1038/s41387-019-0095-8.
- Lim, S., K. Soh, and U. Kuppusamy. 2008. Effects of naringenin on lipogenesis, lipolysis and glucose uptake in rat adipocyte primary culture: A natural antidiabetic agent. The Internet Journal of Alternative Medicine 5 (2):1–5. doi: 10.5580/22f3.
- Lin, A. H.-M., B.-H. Lee, and W.-J. Chang. 2016. Small intestine mucosal α-glucosidase: A missing feature of in vitro starch digestibility. Food Hydrocolloids. 53:163–71. doi: 10.1016/j.foodhyd.2015.03.002.
- Liu, L., S. Shan, K. Zhang, Z.-Q. Ning, X.-P. Lu, and Y.-Y. Cheng. 2008. Naringenin and hesperetin, two flavonoids derived from citrus aurantium up-regulate transcription of adiponectin. Phytotherapy Research: PTR 22 (10):1400–3. doi: 10.1002/ptr.2504.
- Livesey, G., R. Taylor, T. Hulshof, and J. Howlett. 2008. Glycemic response and health - A systematic review and meta-analysis: Relations between dietary glycemic properties and health outcomes. American Journal of Clinical Nutrition. 87:258S–268S. doi: 10.1093/ajcn/87.1.258s.
- Livesey, G., R. Taylor, H. F. Livesey, A. E. Buyken, D. J. A. Jenkins, L. S. A. Augustin, J. L. Sievenpiper, A. W. Barclay, S. Liu, T. M. S. Wolever, et al. 2019. Dietary glycemic index and load and the risk of type 2 diabetes: Assessment of causal relations. Nutrients 11 (6):1436. doi: 10.3390/nu11061436.
- Lo Piparo, E., H. Scheib, N. Frei, G. Williamson, M. Grigorov, and C. J. Chou. 2008. Flavonoids for controlling starch digestion: Structural requirements for inhibiting human alpha-amylase. Journal of Medicinal Chemistry 51 (12):3555–61. doi: 10.1021/jm800115x.
- Manzano, S., and G. Williamson. 2010. Polyphenols and phenolic acids from strawberry and apple decrease glucose uptake and transport by human intestinal Caco-2 cells. Molecular Nutrition & Food Research 54 (12):1773–80. doi: 10.1002/mnfr.201000019.
- Mayneris-Perxachs, J., J. M. Alcaide-Hidalgo, E. de la Hera, J. M. del Bas, L. Arola, and A. Caimari. 2019. Supplementation with biscuits enriched with hesperidin and naringenin is associated with an improvement of the metabolic syndrome induced by a cafeteria diet in rats. Journal of Functional Foods 61:103504. doi: 10.1016/j.jff.2019.103504.
- Menichini, F., M. R. Loizzo, M. Bonesi, F. Conforti, D. De Luca, G. A. Statti, B. de Cindio, F. Menichini, and R. Tundis. 2011. Phytochemical profile, antioxidant, anti-inflammatory and hypoglycemic potential of hydroalcoholic extracts from Citrus medica l. cv diamante flowers, leaves and fruits at two maturity stages. Food and Chemical Toxicology: An International Journal Published for the British Industrial Biological Research Association 49 (7):1549–55. doi: 10.1016/j.fct.2011.03.048.
- Miyata, Y., H. Tanaka, A. Shimada, T. Sato, A. Ito, T. Yamanouchi, and H. Kosano. 2011. Regulation of adipocytokine secretion and adipocyte hypertrophy by polymethoxyflavonoids, nobiletin and tangeretin. Life Sciences 88 (13-14):613–8. doi: 10.1016/j.lfs.2011.01.024.
- Mulvihill, E. E., E. M. Allister, B. G. Sutherland, D. E. Telford, C. G. Sawyez, J. Y. Edwards, J. M. Markle, R. A. Hegele, and M. W. Huff. 2009. Naringenin prevents dyslipidemia, apolipoprotein b overproduction, and hyperinsulinemia in LDL receptor-null mice with diet-induced insulin resistance. Diabetes 58 (10):2198–210. doi: 10.2337/db09-0634.
- Mulvihill, E. E., J. M. Assini, J. K. Lee, E. M. Allister, B. G. Sutherland, J. B. Koppes, C. G. Sawyez, J. Y. Edwards, D. E. Telford, A. Charbonneau, et al. 2011. Nobiletin attenuates VLDL overproduction, dyslipidemia, and atherosclerosis in mice with diet-induced insulin resistance. Diabetes 60 (5):1446–57. doi: 10.2337/db10-0589.
- Murugesan, N., K. Woodard, R. Ramaraju, F. L. Greenway, A. A. Coulter, and C. J. Rebello. 2020. Naringenin increases insulin sensitivity and metabolic rate: A case study. Journal of Medicinal Food 23 (3):343–8. doi: 10.1089/jmf.2019.0216.
- Nguyen, T. T. H., S.-H. Yu, J. Kim, E. An, K. Hwang, J.-S. Park, and D. Kim. 2015. Enhancement of quercetin water solubility with steviol glucosides and the studies of biological properties. Functional Foods in Health and Disease 5 (12):437. doi: 10.31989/ffhd.v5i12.221.
- Nguyen‐Ngo, C., J. C. Willcox, and M. Lappas. 2019. Anti-diabetic, anti-inflammatory, and anti-oxidant effects of naringenin in an in vitro human model and an in vivo murine model of gestational diabetes mellitus. Molecular Nutrition & Food Research 63 (19):e1900224. doi: 10.1002/mnfr.201900224.
- Nomura, M., T. Takahashi, N. Nagata, K. Tsutsumi, S. Kobayashi, T. Akiba, K. Yokogawa, S. Moritani, and K. Miyamoto. 2008. Inhibitory mechanisms of flavonoids on insulin-stimulated glucose uptake in MC3T3-G2/PA6 adipose cells. Biological & Pharmaceutical Bulletin 31 (7):1403–9. doi: 10.1248/bpb.31.1403.
- Nyambe-Silavwe, H., J. A. Villa-Rodriguez, I. Ifie, M. Holmes, E. Aydin, J. M. Jensen, and G. Williamson. 2015. Inhibition of human α-amylase by dietary polyphenols. Journal of Functional Foods 19:723–32. doi: 10.1016/j.jff.2015.10.003.
- O’Neil, C. E., T. A. Nicklas, G. C. Rampersaud, and V. L. Fulgoni. III. 2012. 100% orange juice consumption is associated with better diet quality, improved nutrient adequacy, decreased risk for obesity, and improved biomarkers of health in adults: National health and nutrition examination survey, 2003-2006. Nutrition Journal 11 (1):107. doi: 10.1186/1475-2891-11-107.
- Oboh, G., and A. O. Ademosun. 2011. Shaddock peels (Citrus maxima) phenolic extracts inhibit α-amylase, α-glucosidase and angiotensin i-converting enzyme activities: A nutraceutical approach to diabetes management. Diabetes & Metabolic Syndrome: Clinical Research & Reviews 5 (3):148–52. doi: 10.1016/j.dsx.2012.02.008.
- Okabe, Y., T. Shimada, T. Horikawa, K. Kinoshita, K. Koyama, K. Ichinose, M. Aburada, and K. Takahashi. 2014. Suppression of adipocyte hypertrophy by polymethoxyflavonoids isolated from Kaempferia parviflora. Phytomedicine: International Journal of Phytotherapy and Phytopharmacology 21 (6):800–6. doi: 10.1016/j.phymed.2014.01.014.
- Onda, K., N. Horike, T. Suzuki, and T. Hirano. 2013. Polymethoxyflavonoids tangeretin and nobiletin increase glucose uptake in murine adipocytes. Phytotherapy Research: PTR 27 (2):312–6. doi: 10.1002/ptr.4730.
- Ortiz-Andrade, R. R., J. C. Sánchez-Salgado, G. Navarrete-Vázquez, S. P. Webster, M. Binnie, S. García-Jiménez, I. León-Rivera, P. Cigarroa-Vázquez, R. Villalobos-Molina, and S. Estrada-Soto. 2008. Antidiabetic and toxicological evaluations of naringenin in normoglycaemic and NIDDM rat models and its implications on extra-pancreatic glucose regulation. Diabetes, Obesity and Metabolism 10 (11):1097–104. doi: 10.1111/j.1463-1326.2008.00869.x.
- Owira, P. M. O., and J. A. O. Ojewole. 2009. Grapefruit juice improves glycemic control but exacerbates metformin-induced lactic acidosis in non-diabetic rats. Methods and Findings in Experimental and Clinical Pharmacology 31 (9):563–70. doi: 10.1358/mf.2009.31.9.1435463.
- Park, H.-J., U. J. Jung, S.-J. Cho, H.-K. Jung, S. Shim, and M.-S. Choi. 2013. Citrus unshiu peel extract ameliorates hyperglycemia and hepatic steatosis by altering inflammation and hepatic glucose- and lipid-regulating enzymes in db/db mice. The Journal of Nutritional Biochemistry 24 (2):419–27. doi: 10.1016/j.jnutbio.2011.12.009.
- Park, J. B. 1999. Flavonoids are potential inhibitors of glucose uptake in U937 cells. Biochemical and Biophysical Research Communications 260 (2):568–74. doi: 10.1006/bbrc.1999.0890.
- Parmar, H. S., P. Jain, D. S. Chauhan, M. K. Bhinchar, V. Munjal, M. Yusuf, K. Choube, A. Tawani, V. Tiwari, E. Manivannan, et al. 2012. DPP-iv inhibitory potential of naringin: An in silico, in vitro and in vivo study. Diabetes Research and Clinical Practice 97 (1):105–11. doi: 10.1016/j.diabres.2012.02.011.
- Pereira-Caro, G., G. Borges, I. Ky, A. Ribas, L. Calani, D. Del Rio, M. N. Clifford, S. A. Roberts, and A. Crozier. 2015. In vitro colonic catabolism of orange juice (poly)phenols. Molecular Nutrition & Food Research 59 (3):465–75. doi: 10.1002/mnfr.201400779.
- Pereira-Caro, G., G. Borges, J. van der Hooft, M. N. Clifford, D. Del Rio, M. E. J. Lean, S. A. Roberts, M. B. Kellerhals, and A. Crozier. 2014. Orange juice (poly)phenols are highly bioavailable in humans. The American Journal of Clinical Nutrition 100 (5):1378–84. doi: 10.3945/ajcn.114.090282.
- Pereira-Caro, G., I. A. Ludwig, T. Polyviou, D. Malkova, A. García, J. M. Moreno-Rojas, and A. Crozier. 2016. Identification of plasma and urinary metabolites and catabolites derived from orange juice (poly)phenols: Analysis by high-performance liquid chromatography-high-resolution mass spectrometry. Journal of Agricultural and Food Chemistry 64 (28):5724–35. doi: 10.1021/acs.jafc.6b02088.
- Petersen, M. C., D. F. Vatner, and G. I. Shulman. 2017. Regulation of hepatic glucose metabolism in health and disease. Nature Reviews. Endocrinology 13 (10):572–87. doi: 10.1038/nrendo.2017.80.
- Ponce, O., R. Benassi, and T. Cesar. 2019. Orange juice associated with a balanced diet mitigated risk factors of metabolic syndrome: A randomized controlled trial. Journal of Nutrition & Intermediary Metabolism 17 (May):100101. doi: 10.1016/j.jnim.2019.100101.
- Priscilla, D. H., D. Roy, A. Suresh, V. Kumar, and K. Thirumurugan. 2014. Naringenin inhibits α-glucosidase activity: A promising strategy for the regulation of postprandial hyperglycemia in high fat diet fed streptozotocin induced diabetic rats. Chemico-Biological Interactions 210 (1):77–85. doi: 10.1016/j.cbi.2013.12.014.
- Proença, C., M. Freitas, D. Ribeiro, S. M. Tomé, A. N. Araújo, A. M. S. Silva, P. A. Fernandes, and E. Fernandes. 2019. The dipeptidyl peptidase-4 inhibitory effect of flavonoids is hindered in protein rich environments. Food & Function 10 (9):5718–31. doi: 10.1039/C9FO00722A.
- Pu, P., D.-M. Gao, S. Mohamed, J. Chen, J. Zhang, X.-Y. Zhou, N.-J. Zhou, J. Xie, and H. Jiang. 2012. Naringin ameliorates metabolic syndrome by activating amp-activated protein kinase in mice fed a high-fat diet. Archives of Biochemistry and Biophysics 518 (1):61–70. doi: 10.1016/j.abb.2011.11.026.
- Purushotham, A., M. Tian, and M. A. Belury. 2009. The citrus fruit flavonoid naringenin suppresses hepatic glucose production from fao hepatoma cells. Molecular Nutrition & Food Research 53 (2):300–7. doi: 10.1002/mnfr.200700514.
- Pyner, A., H. Nyambe-Silavwe, and G. Williamson. 2017. Inhibition of human and rat sucrase and maltase activities to assess antiglycemic potential: Optimization of the assay using acarbose and polyphenols. Journal of Agricultural and Food Chemistry 65 (39):8643–51. doi: 10.1021/acs.jafc.7b03678.
- Rampersaud, G. C., and M. F. Valim. 2017. 100% citrus juice: Nutritional contribution, dietary benefits, and association with anthropometric measures. Critical Reviews in Food Science and Nutrition 57 (1):129–40. doi: 10.1080/10408398.2013.862611.
- Rasouli, H., S. M.-B. Hosseini-Ghazvini, H. Adibi, and R. Khodarahmi. 2017. Differential α-amylase/α-glucosidase inhibitory activities of plant-derived phenolic compounds: A virtual screening perspective for the treatment of obesity and diabetes. Food & Function 8 (5):1942–54. doi: 10.1039/C7FO00220C.
- Razavi, B. M., and H. Hosseinzadeh. 2019. A review of the effects of Citrus paradisi (grapefruit) and its flavonoids, naringin, and naringenin in metabolic syndrome. In Bioactive food as dietary interventions for diabetes, ed. R. R. Watson and V. R. Preedy, 515–43. London: Academic Press. doi: 10.1016/B978-0-12-813822-9.00034-5.
- Rebello, C. J., F. L. Greenway, F. H. Lau, Y. Lin, J. M. Stephens, W. D. Johnson, and A. A. Coulter. 2019. Naringenin promotes thermogenic gene expression in human white adipose tissue. Obesity 27 (1):103–11. doi: 10.1002/oby.22352.
- Ren, B., W. Qin, F. Wu, S. Wang, C. Pan, L. Wang, B. Zeng, S. Ma, and J. Liang. 2016. Apigenin and naringenin regulate glucose and lipid metabolism, and ameliorate vascular dysfunction in type 2 diabetic rats. European Journal of Pharmacology 773:13–23. doi: 10.1016/j.ejphar.2016.01.002.
- Richard, A. J., Z. Amini-Vaughan, D. M. Ribnicky, and J. M. Stephens. 2013. Naringenin inhibits adipogenesis and reduces insulin sensitivity and adiponectin expression in adipocytes. Evidence-Based Complementary and Alternative Medicine: eCAM 2013:549750–10. doi: 10.1155/2013/549750.
- Rines, A. K., K. Sharabi, C. D. J. Tavares, and P. Puigserver. 2016. Targeting hepatic glucose metabolism in the treatment of type 2 diabetes. Nature Reviews. Drug Discovery 15 (11):786–804. doi: 10.1038/nrd.2016.151.
- Röder, P. V., B. Wu, Y. Liu, and W. Han. 2016. Pancreatic regulation of glucose homeostasis. Experimental & Molecular Medicine 48:e219. doi: 10.1038/emm.2016.6.
- Saito, T., D. Abe, and K. Sekiya. 2007. Nobiletin enhances differentiation and lipolysis of 3T3-L1 adipocytes. Biochemical and Biophysical Research Communications 357 (2):371–6. doi: 10.1016/j.bbrc.2007.03.169.
- Sathiyabama, R. G., G. Rajiv Gandhi, M. Denadai, G. Sridharan, G. Jothi, P. Sasikumar, J. d. S. Siqueira Quintans, N. Narain, L. E. Cuevas, et al. 2018. Evidence of insulin-dependent signalling mechanisms produced by Citrus sinensis (l.) Osbeck fruit peel in an insulin resistant diabetic animal model. Food and Chemical Toxicology: An International Journal Published for the British Industrial Biological Research Association 116 (Pt B):86–99. doi: 10.1016/j.fct.2018.03.050.
- Sayem, A., A. Arya, H. Karimian, N. Krishnasamy, A. Ashok Hasamnis, and C. Hossain. 2018. Action of phytochemicals on insulin signaling pathways accelerating glucose transporter (GLUT4) protein translocation. Molecules 23 (2):258. doi: 10.3390/molecules23020258.
- Schulze, C., A. Bangert, G. Kottra, K. E. Geillinger, B. Schwanck, H. Vollert, W. Blaschek, and H. Daniel. 2014. Inhibition of the intestinal sodium-coupled glucose transporter 1 (SGLT1) by extracts and polyphenols from apple reduces postprandial blood glucose levels in mice and humans. Molecular Nutrition & Food Research 58 (9):1795–808. doi: 10.1002/mnfr.201400016.
- Sdiri, S., A. Bermejo, P. Aleza, P. Navarro, and A. Salvador. 2012. Phenolic composition, organic acids, sugars, vitamin c and antioxidant activity in the juice of two new triploid late-season mandarins. Food Research International 49 (1):462–8. doi: 10.1016/j.foodres.2012.07.040.
- Shahidi, F., and H. Peng. 2018. Bioaccessibility and bioavailability of phenolic compounds. Journal of Food Bioactives 4:11–68. doi: 10.31665/JFB.2018.4162.
- Sharabi, K., C. D. J. Tavares, A. K. Rines, and P. Puigserver. 2015. Molecular pathophysiology of hepatic glucose production. Molecular Aspects of Medicine 46:21–33. doi: 10.1016/j.mam.2015.09.003.
- Shen, W., Y. Xu, and Y.-H. Lu. 2012. Inhibitory effects of citrus flavonoids on starch digestion and antihyperglycemic effects in HepG2 cells. Journal of Agricultural and Food Chemistry 60 (38):9609–19. doi: 10.1021/jf3032556.
- Smith, U., and B. B. Kahn. 2016. Adipose tissue regulates insulin sensitivity: Role of adipogenesis, de novo lipogenesis and novel lipids. Journal of Internal Medicine 280 (5):465–75. doi: 10.1111/joim.12540.
- Sun, L., and M. Miao. 2020. Dietary polyphenols modulate starch digestion and glycaemic level: A review. Critical Reviews in Food Science and Nutrition 60 (4):541–55. doi: 10.1080/10408398.2018.1544883.
- Sun, Y., W. Tao, H. Huang, X. Ye, and P. Sun. 2019. Flavonoids, phenolic acids, carotenoids and antioxidant activity of fresh eating citrus fruits, using the coupled in vitro digestion and human intestinal HepG2 cells model. Food Chemistry 279:321–7. doi: 10.1016/j.foodchem.2018.12.019.
- Sundaram, R., P. Shanthi, and P. Sachdanandam. 2014. Effect of tangeretin, a polymethoxylated flavone on glucose metabolism in streptozotocin-induced diabetic rats. Phytomedicine: International Journal of Phytotherapy and Phytopharmacology 21 (6):793–9. doi: 10.1016/j.phymed.2014.01.007.
- Tadera, K., Y. Minami, K. Takamatsu, and T. Matsuoka. 2006. Inhibition of alpha-glucosidase and alpha-amylase by flavonoids. Journal of Nutritional Science and Vitaminology 52 (2):149–53. doi: 10.3177/jnsv.52.149.
- Tomás-Navarro, M., F. Vallejo, E. Sentandreu, J. L. Navarro, and F. A. Tomás-Barberán. 2014. Volunteer stratification is more relevant than technological treatment in orange juice flavanone bioavailability. Journal of Agricultural and Food Chemistry 62 (1):24–7. doi: 10.1021/jf4048989.
- Tomás-Navarro, M., F. Vallejo, and F. A. Tomás-Barberán. 2014. Bioavailability and metabolism of citrus fruit beverage flavanones in humans. In Polyphenols in human health and disease, vol. 1, 537–51. Academic Press, London Ronald R.Watson, Victor R. Preedy and Sherma Zibadi, eds. doi: 10.1016/B978-0-12-398456-2.00040-2.
- Tommasini, S., M. L. Calabrò, R. Stancanelli, P. Donato, C. Costa, S. Catania, V. Villari, P. Ficarra, and R. Ficarra. 2005. The inclusion complexes of hesperetin and its 7-rhamnoglucoside with (2-hydroxypropyl)-beta-cyclodextrin. Journal of Pharmaceutical and Biomedical Analysis 39 (3-4):572–80. doi: 10.1016/j.jpba.2005.05.009.
- Tripoli, E., M. L. Guardia, S. Giammanco, D. D. Majo, and M. Giammanco. 2007. Citrus flavonoids: Molecular structure, biological activity and nutritional properties: A review. Food Chemistry 104 (2):466–79. doi: 10.1016/j.foodchem.2006.11.054.
- Turner, T., and B. Burri. 2013. Potential nutritional benefits of current citrus consumption. Agriculture 3 (1):170–87. doi: 10.3390/agriculture3010170.
- Tweedle, D., and P. Nightingale. 1989. Anesthesia and gastrointestinal surgery. Acta Chirurgica Scandinavica. Supplementum 550:131–9.
- Uddin, N., M. R. Hasan, M. M. Hossain, A. Sarker, A. H. M. N. Hasan, A. F. M. M. Islam, M. M. H. Chowdhury, M. S. Rana, A. H. M. Nazmul Hasan, A. F. M. Mahmudul Islam, et al. 2014. In vitro α–amylase inhibitory activity and in vivo hypoglycemic effect of methanol extract of Citrus macroptera Montr. fruit. Asian Pacific Journal of Tropical Biomedicine 4 (6):473–9. doi: 10.12980/APJTB.4.2014C1173.
- Vallejo, F., M. Larrosa, E. Escudero, M. P. Zafrilla, B. Cerdá, J. Boza, M. T. García-Conesa, J. C. Espín, and F. A. Tomás-Barberán. 2010. Concentration and solubility of flavanones in orange beverages affect their bioavailability in humans. Journal of Agricultural and Food Chemistry 58 (10):6516–24. doi: 10.1021/jf100752j.
- Vidal-Puig, A. J., R. V. Considine, M. Jimenez-Liñan, A. Werman, W. J. Pories, J. F. Caro, and J. S. Flier. 1997. Peroxisome proliferator-activated receptor gene expression in human tissues. Effects of obesity, weight loss, and regulation by insulin and glucocorticoids. Journal of Clinical Investigation 99 (10):2416–22. doi: 10.1172/JCI119424.
- Villa-Rodriguez, J. A., E. Aydin, J. S. Gauer, A. Pyner, G. Williamson, and A. Kerimi. 2017. Green and chamomile teas, but not acarbose, attenuate glucose and fructose transport via inhibition of GLUT2 and GLUT5. Molecular Nutrition & Food Research 61 (12):1700566. doi: 10.1002/mnfr.201700566.
- Visvanathan, R., M. J. Houghton, and G. Williamson. 2021. Maltoheptaoside hydrolysis with chromatographic detection and starch hydrolysis with reducing sugar analysis: Comparison of assays allows assessment of the roles of direct α-amylase inhibition and starch complexation. Food Chemistry 343:128423. doi: 10.1016/j.foodchem.2020.128423.
- Visvanathan, R., C. Jayathilake, R. Liyanage, and R. Sivakanesan. 2019. Applicability and reliability of the glucose oxidase method in assessing α-amylase activity. Food Chemistry 275:265–72. doi: 10.1016/j.foodchem.2018.09.114.
- WHO. 2016. Global report on diabetes. World Health Organization. Geneva, Switzerland: WHO Press.
- Williamson, G. 2013. Possible effects of dietary polyphenols on sugar absorption and digestion. Molecular Nutrition & Food Research 57 (1):48–57. doi: 10.1002/mnfr.201200511.
- Williamson, G. 2017. The role of polyphenols in modern nutrition. Nutrition Bulletin 42 (3):226–35. doi: 10.1111/nbu.12278.
- Williamson, G., C. D. Kay, and A. Crozier. 2018. The bioavailability, transport, and bioactivity of dietary flavonoids: A review from a historical perspective. Comprehensive Reviews in Food Science and Food Safety 17 (5):1054–112. doi: 10.1111/1541-4337.12351.
- Wu, S., and L. Tian. 2017. Diverse phytochemicals and bioactivities in the ancient fruit and modern functional food pomegranate (punica granatum). Molecules (Basel, Switzerland) 22 (10):1606. doi: 10.3390/molecules22101606.
- Xing, B., F. Yang, and X. Wu. 2016. Naringenin enhances the efficacy of human embryonic stem cell-derived pancreatic endoderm in treating gestational diabetes mellitus mice. Journal of Pharmacological Sciences 131 (2):93–100. doi: 10.1016/j.jphs.2016.04.014.
- Yang, H., and L. Yang. 2016. Targeting camp/pka pathway for glycemic control and type 2 diabetes therapy. Journal of Molecular Endocrinology 57 (2):R93–108. doi: 10.1530/JME-15-0316.
- Yang, Y., J. Wolfram, K. Boom, X. Fang, H. Shen, and M. Ferrari. 2013. Hesperetin impairs glucose uptake and inhibits proliferation of breast cancer cells. Cell Biochemistry and Function 31 (5):374–9. doi: 10.1002/cbf.2905.
- Yoshida, H., W. Watanabe, H. Oomagari, E. Tsuruta, M. Shida, and M. Kurokawa. 2013. Citrus flavonoid naringenin inhibits TLR2 expression in adipocytes. The Journal of Nutritional Biochemistry 24 (7):1276–84. doi: 10.1016/j.jnutbio.2012.10.003.
- Zhao, C., C. Zhao, and H. Zhao. 2020. Defective insulin receptor signaling in patients with gestational diabetes is related to dysregulated miR-140 which can be improved by naringenin. The International Journal of Biochemistry & Cell Biology 128:105824. doi: 10.1016/j.biocel.2020.105824. PMC: 32814161.
- Zheng, M., S. Lu, and J. Xing. 2021. Enhanced antioxidant, anti-inflammatory and α-glucosidase inhibitory activities of citrus hesperidin by acid-catalyzed hydrolysis. Food Chemistry 336:127539. doi: 10.1016/j.foodchem.2020.127539.
- Zygmunt, K., B. Faubert, J. MacNeil, and E. Tsiani. 2010. Naringenin, a citrus flavonoid, increases muscle cell glucose uptake via AMPK. Biochemical and Biophysical Research Communications 398 (2):178–83. doi: 10.1016/j.bbrc.2010.06.048.