Abstract
Branched chain amino acids (BCAA) and their derivatives are bioactive molecules with pleiotropic functions in the human body. Elevated fasting blood BCAA concentrations are considered as a metabolic hallmark of obesity, insulin resistance, dyslipidaemia, nonalcoholic fatty liver disease, type 2 diabetes and cardiovascular disease. However, since increased BCAA amount is observed both in metabolically healthy and obese subjects, a question whether BCAA are mechanistic drivers of insulin resistance and its morbidities or only markers of metabolic dysregulation, still remains open. The beneficial effects of BCAA on body weight and composition, aerobic capacity, insulin secretion and sensitivity demand high catabolic potential toward amino acids and/or adequate BCAA intake. On the opposite, BCAA-related inhibition of lipogenesis and lipolysis enhancement may preclude impairment in insulin sensitivity. Thereby, the following review addresses various strategies pertaining to the modulation of BCAA catabolism and the possible roles of BCAA in energy homeostasis. We also aim to elucidate mechanisms behind the heterogeneity of ramifications associated with BCAA modulation.
Introduction
The tremendous economic and healthcare burden connected with the rising number of overweight and obesity incidents requires effective methods for its prevention and treatment. Obesity, with a consequent insufficient metabolic control, precedes the development of insulin resistance, type 2 diabetes and cardiovascular disorders (Wondmkun Citation2020), therefore understanding the biochemical networks underlying the energy imbalance is urgent. Accordingly, the biological markers of diabetes that predict the disease severity are highly anticipated. It is well-known that the inappropriate intermediary turnover of an array of nutrients prevails during obesity and related diseases, with increased concentrations of circulating glucose, long-chain fatty acids (LCFA), and ketone bodies constituting the insulin resistance metabotype (Knebel et al. Citation2016). Until recently, the role of amino acids was largely neglected despite their fundamental biological functions and strong associations with metabolic morbidities. Among amino acids, branched chain amino acids (BCAA; leucine, isoleucine, valine) act as a central hub linking nutrient turnover. Importantly, modification of amino acid composition in a diet is an alternative therapeutic approach to counteract metabolic disorders and a potential replacement for long-term caloric restriction, which may be difficult to implement for patients.
BCAA metabolism and molecular basis of their action
BCAA account for approximately 35% of essential amino acids in the body, with the approximate concentrations of circulating amino acids maintained at the level of 200 μM for valine, 100 μM for leucine and 60 μM for isoleucine in the fasted state (Neinast, Murashige, et al. Citation2019). Since BCAA cannot be produced in the human cells, they need to be provided with food or be synthesized by gut microbiota (Pedersen et al. Citation2016). BCAA absorption in enterocytes, reabsorption in nephrons and further uptake by BCAA-catabolizing tissues are facilitated by several protein transporters common for neutral amino acids. The most prevalent are: L-type amino acid transporter 1/protein complex of solute carrier family 7 member 5 (LAT1/SLC7a5) and SLC3a2, the latter chain that ensures proper stabilization of the complex within the plasma membrane (Biswas, Duffley, and Pulinilkunnil Citation2019). Other transport systems include the Na+-dependent SLC6a19/B0AT1 carrier, with a preference for large neutral aliphatic amino acids, which is localized in the apical membrane of intestinal and renal epithelium (Hewton, Johal, and Parker Citation2021). BCAA metabolism occurs within the mitochondria, the site of catabolic enzymes localization, and recently, a mitochondrial transporter for BCAA has been recognized, namely SLC25a44 (Yoneshiro et al. Citation2019). First step in BCAA utilization constitutes the reversible conversion of leucine, isoleucine and valine into their corresponding α-keto acids (BCKA), such as α-ketoisocaproate (KIC), α-keto-β-methylvalerate (KMV) and α-ketoisovalerate (KIV), catalyzed by branched chain amino transferase (Bcat) (). Mitochondrial Bcat isoform (BCATm or Bcat2) is ubiquitously expressed, while the cytosolic isoenzyme (BCATc or Bcat1) abundance is limited to the brain, ovaries and placenta (Polis and Samson Citation2020; Holeček Citation2020). Unlike other essential amino acids, BCAA are firstly degraded in the skeletal muscle or heart, instead of liver, because of very low hepatic Bcat2 activity (Holeček Citation2020). As a consequence, a marked pool of BCAA, that avoided hepatic uptake, appears in the systemic circulation. Secondly, BCKA undergo irreversible oxidative decarboxylation mediated by branched chain α-keto acid dehydrogenase (Bckdh) to produce coenzyme A (CoA) esters. Bckdh is composed of four catalytic subunits (Bckdha/E1α, Bckdhb/E1β, Dbt/E2, Dld/E3), of which E1 is a thiamin-dependent decarboxylase, whilst E2 subunit belongs to acyltransferases. E3 component is a flavoprotein and participates in the re-oxidation of lipoic acid paralleled by a reduction in nicotinamide adenine dinucleotide (NAD+) to NADH. To guarantee a steady plasma level of BCAA, Bckdh activity is strictly controlled under various growth and nutrient environments. The increased availability of leucine and isoleucine stimulates Bckdh activity, while NADH and CoA esters are allosteric inhibitors of the enzyme (Kainulainen, Hulmi, and Kujala Citation2013). Furthermore, the rate of Bckdh-mediated reactions is modified by post-translational phosphorylation/dephosphorylation of the E1α subunit (Ser 293). Phosphorylation of this component is catalyzed by branched chain α-keto acid dehydrogenase kinase (Bckdk) and leads to a reduction in Bckdh activity (). In turn, dephosphorylation of Bckdha-E1α activates the enzyme and is mediated by protein phosphatase 2 Cm (PP2Cm), encoded by protein phosphatase Mg2+/Mn2+ dependent 1 K (PPM1K) gene (Kainulainen, Hulmi, and Kujala Citation2013). The resulting acyl-CoA esters are catabolized separately and yield either ATP, CO2 and H2O, or are incorporated into alternative pathways for lipogenesis, gluconeogenesis, ketone bodies or de novo cholesterol synthesis (Neinast, Murashige, et al. Citation2019). BCAA catabolism provides also nitrogen for the synthesis of non-essential amino acids and DNA (Mayers et al. Citation2016; White et al. Citation2020) or urea cycle intermediates in the skeletal muscle during obesity (White et al. Citation2020) ().
Figure 1. Associations between glucose, fatty acid and amino acid metabolism. BCAA are metabolized within mitochondria through branched chain amino acid aminotransferase (Bcat) that catalyzes the transamination of BCAA by the transfer of amino group to α-ketoglutarate with a simultaneous generation of glutamate. Glutamate provides amino group to generate alanine from pyruvate or for condensation with ammonia to form glutamine. Branched chain keto acids (BCKA) are oxidatively decarboxylated by branched chain keto acid dehydrogenase (Bckdh). Finally, acyl-CoA metabolism diverges into separate pathways to CO2, and succinyl-CoA (valine, glucogenic) or acetyl-CoA (leucine, ketogenic), or acetyl-CoA and succinyl-CoA (isoleucine, glucogenic and ketogenic) production. Acetyl-CoA may also derive from lipolysis or glycolysis, and its conversion to CO2 and H2O depends on tricarboxylic acid cycle capacity. Among valine intermediates, β-hydroxyisobutyrate (3-HIB) and β-aminoisobutyric acid (BAIBA), can be secreted outside the cell and exert paracrine/endocrine functions. Abbreviations: Abat, 4-aminobutyrate aminotransferase; Acat, acetyl-CoA acetyltransferase; Acc, acetyl-CoA carboxylase; Acl, ATP citrate lyase; Acs, fatty acyl-CoA synthetase; Agpat, 1-acylglycerol-3-phosphate-O-acyltransferase; Aldh6a1/Mmsdh, aldehyde dehydrogenase 6 family member A1/methylmalonic semialdehyde dehydrogenase; Alt, alanine transaminase; Ast, aspartate transaminase; Atgl, adipose triglyceride lipase; BCFA, branched chain fatty acids; Bckdk, branched chain keto acid dehydrogenase kinase; Cpt1/2, carnitine palmitoyltransferase 1/2; Cs, citrate synthase; Dgat, diacylglycerol acyltransferase; Fasn, fatty acid synthase; Gldh, glutamate dehydrogenase; Gpat, glycerol-3-phosphate acyltransferase; Gs, glutamine synthetase; Hadha, hydroxyacyl-CoA dehydrogenase subunit α; Hbdh, 3-hydroxybutyrate dehydrogenase; Hibadh, 3-hydroxyisobutyric acid dehydrogenase; Hibch, 3-hydroxy-isobutyryl-CoA hydrolase; HMG lyase, 3-hydroxy-3-methylglutaryl-CoA lyase; Hsl, hormone-sensitive lipase; Ibdh, isobutyryl-CoA dehydrogenase; Ivd, isovaleryl-CoA dehydrogenase; Kicd, α-ketoisocaproate dioxygenase; Ldh, lactate dehydrogenase; Mcd, malonyl-CoA decarboxylase; Me, malic enzyme; Mgat, acyl CoA:monoacylglycerol acyltransferase; Mgl, monoacylglycerol lipase; Mhbd, α-methyl-β-hydroxybutyryl-CoA dehydrogenase; MUFA, monounsaturated fatty acids; Mut, methylmalonyl-CoA mutase; Oxct1, 3-oxoacid CoA transferase; Pc, pyruvate carboxylase; Pcc, propionyl-CoA carboxylase; Pdh, pyruvate dehydrogenase; Pepck, phosphoenolpyruvate carboxykinase; Pk, pyruvate kinase; PP2Cm, protein phosphatase 2 Cm; Sbcad, methylbutyryl CoA dehydrogenase; Scd1, stearoyl-CoA desaturase; Sdh, serine dehydratase; Shmt, serine hydroxymethyltransferase.
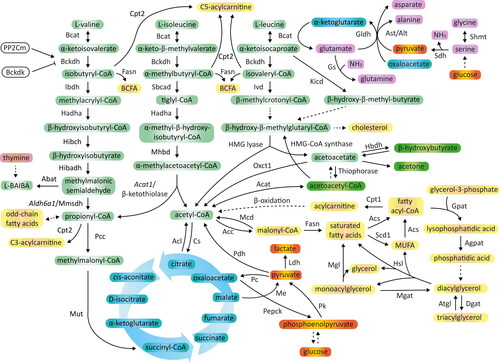
Among various factors that trigger BCAA turnover, Krüppel-like factor 15 (KLF15) is also an upstream controller of carbohydrate and lipid homeostasis (Sun et al. Citation2016; Gray et al. Citation2007; Prosdocimo et al. Citation2014). The loss of KLF15 is responsible for downstream BCAA metabolism defects (e.g., reduced Bcat2 level) (Sun et al. Citation2016), while BCAA reciprocally inhibit KLF15 through phosphoinositide-3-kinase (PI3K)/Akt signaling, therefore affecting the energy homeostasis (Liu, Dong, et al. Citation2017). Moreover, liver-specific lipogenesis-activating transcriptional factor, i.e., carbohydrate response element-binding protein (ChREBP-β) can orchestrate lipid and BCAA metabolism by the activation of de novo lipogenesis and an increase in Bckdk/PP2Cm ratio (White et al. Citation2018), which predispose to BCAA accumulation. Nonetheless, the presence of ChREBP binding site in the regulatory motif of Bckdk was confirmed in rats and humans, but not in mice. It is one of the causes for high fructose-mediated increase in hepatic Bckdk activity in rats, but not in high sucrose fed mice (Lee et al. Citation2021).
Pleiotropic functions of BCAA result from the activation of multiple cellular signaling pathways. The best characterized is mammalian target of rapamycin (mTOR) activation, which is a serine-threonine protein kinase from PI3K family. There are two functionally independent mTOR complexes, mTORC1 and mTORC2, that co-operate with diverse downstream factors, including ribosomal protein S6 kinase 1 (p70 S6K1), eukaryotic translation initiation factor 4E binding protein 1 (4E-BP1) and sterol regulatory element binding protein 1 (Srebp1) (Jhanwar-Uniyal et al. Citation2019) to regulate the vast array of physiological processes. BCAA drive the mTORC1 protein stability and its translocation to the lysosomal surface, wherein mTORC1 upstream activator, GTP-loaded Rheb, is localized () (Bar-Peled and Sabatini Citation2014). Therefore, the activation of mTORC1 influences protein synthesis, cellular proliferation, glucose and lipid metabolism, as well as the responses to endogenous or environmental stressors (Dickinson et al. Citation2011; Saxton and Sabatini Citation2017). The effects of BCAA-related mTORC2 stimulation are concentrated on the cell survival, migration and cytoskeleton organization due to its interactions with a group of kinases related to protein kinase A (PKA), PKG and PKC (AGC kinases) and Ras homologous (Rho) GTPase (Fu and Hall Citation2020).
Figure 2. The network of intracellular signals transduction that modulate mammalian target of rapamycin (mTOR) activity. BCAA within the cell are sensed by leucyl tRNA synthetase and Sestrin, what precludes related GTP binding (Rag) complex activation that in turn stimulates GTP binding to Ras homolog enriched in brain (Rheb) protein and its activation, ultimately leading to mTORC1 upregulation. BCAA also suppress mTORC1 inhibitors, such as general control nonderepressible 2 (Gcn2) and 5’AMP-activated protein kinase (AMPK). The activity of mTORC1 is positively regulated by oxygen through protein regulated in DNA damage and development (Redd1), hormones or growth factors through phosphoinositide-3-kinase (PI3K)/Akt or mitogen-activated protein kinase kinase 1/2 (Mek1/2)/extracellular signal-regulated kinase 1/2 (Erk1/2) signaling cascades, which inactivate tuberous sclerosis complex 2 (TSC2) subunit of the TSC complex that stimulates Rheb activity. Upon activation, mTORC1 regulates various proteins, including unc-51 like autophagy activating kinase 1 (Ulk1), p70 S6 kinase (p70 S6K) and eukaryotic translation initiation factor 4E binding protein 1 (4E-BP1). Abbreviations: Deptor, DEP domain-containing mTOR-interacting protein; Gator, GTP-ase activating protein activity toward Rag; HIF-1α, hypoxia-inducible factor 1α; IRS1/2, insulin receptor substrate 1/2; mLST8, mammalian lethal with SEC13 protein 8; mSin1, mammalian stress-activated protein kinase-interacting 1; PDK1, 3-phosphoinositide-dependent kinase 1; Pras40, proline-rich Akt substrate of 40 kDa; Protor 1/2, protein observed with Rictor 1/2; Raptor, regulated associated protein of mTOR; Rictor, rapamycin insensitive companion of mTOR.
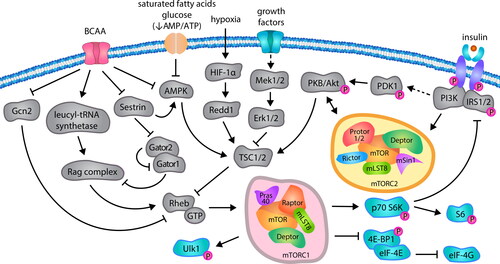
Tissue-specific BCAA metabolism in obesity and its comorbidities
BCKA are mainly produced in the skeletal muscle considering their high BCAA aminotransferase activity. Despite low Bckdh activity, high muscle mass, accounting for approximately 40% of body weight, makes them also an important site of BCKA decarboxylation. BCAA, especially leucine, have unique ability to promote protein synthesis in the skeletal muscle through the mTORC1-mediated upregulation of mRNA translation () (Lynch and Adams Citation2014). Studies in rats and cultured cells showed also an inhibitory role of BCAA in muscle protein breakdown through the reduction in autophagy (Sugawara et al. Citation2009) and downregulation of ubiquitin-proteasome system-driven protein degradation (Zhao et al. Citation2015). During obesity, an increase in BCAA is associated with augmented muscular glutamate production. Excessive nitrogen load is dissipated by the transfer of amino group from glutamate to pyruvate or oxaloacetate to form alanine or aspartate, respectively. Chronic activation of these pathways precludes depletion of pyruvate stores that can be resynthesized from glycine (White et al. Citation2020). Concomitantly, ammonia released due to BCAA transamination and serine dehydratase activity can be detoxified by glutamine synthesis (). The above products (i.e., alanine, aspartate, glutamine) exit skeletal muscle, carrying nitrogen for urea synthesis in the liver and its renal excretion, or provide NH3 for protein, nucleotide or neurotransmitter generation, and move carbons for gluconeogenesis in the liver, kidneys or brain (Holecek Citation2013). This regulation becomes impaired in chronic liver damage, that associates with hyperammonemia, which in turn promotes BCAA catabolism and glutamine generation, lowers plasma branched chain to aromatic amino acid ratio, contributes to hepatic encephalopathy occurrence and progression of liver disease (Holecek et al. Citation2011). BCAA supplementation is recommended as a therapy in the liver cirrhosis since it may prevent ammonia accumulation by glutamine production and an improvement in nutritional status (Dam et al. Citation2013; Gluud et al. Citation2013). However, conflicting results were documented in terms of BCAA administration (Gluud et al. Citation2017). To minimize the risk of BCAA overload, a paralleled α-ketoglutarate and/or phenylbutyrate administration may be considered in order to reduce glutamine breakdown within enterocytes and to enhance its urinary excretion (Holecek Citation2013). Moreover, phase of liver damage (i.e., recovery stage or acute liver injury) as well as dose and duration of BCAA supply should be carefully considered before therapy implementation (Holecek Citation2013).
The metabolic consequences of obesity involve the reorganization of BCAA metabolism throughout the body, and these modifications depend on tissue type and localization. It was shown that adipose tissue exhibits higher rates of BCAA oxidation per mg of tissue in comparison to the skeletal muscle and significantly contributes to systemic BCAA catabolism (Herman et al. Citation2010). Literature data link BCAA metabolism within adipocytes with tissue oxygenation, inflammation and fibrogenesis. In fact, a significant positive association between adipose tissue oxygenation (pO2) and BCAA catabolic genes expression (i.e., Bckdhb, Hadh, Hibadh, Aldh6a1, Hmgcl, Mut, Acat1, Mccc2) was observed in humans (Festa et al. Citation2006). In obesity, adipose tissue is characterized by 44% lower capillary density, decreased blood flow, constrained oxygen consumption and declined mitochondrial oxidative capacity (Engin Citation2017). Hypoxia-mediated reduction in Bckdh and Bcat2 (Wallace et al. Citation2018) evokes higher BCAA level in obese individuals along with insulin resistance as well as plasminogen activator inhibitor-1 (PAI-1) production (Cifarelli et al. Citation2020), which is also known to predispose to type 2 diabetes development (Festa et al. Citation2006). Likewise, white adipose tissue BCATm and Bckdh contents were reduced in various models of obesity (obese Zucker rats, db/db mice, diet-induced obese mice) (Lackey et al. Citation2013). The heterogeneity of adipocyte populations is related to a higher expression of most genes involved in BCAA catabolism in brown adipocytes as compared to white adipose tissue in mice and humans (Nilsen et al. Citation2020). It is attributed to the crucial role of amino acids in thermogenesis, since cold exposure reinforced the expression of BCAA-catabolic genes, while brown adipocyte-restricted defect in BCAA utilization impaired systemic BCAA clearance in BckdhUCP1 knockout mice (Yoneshiro et al. Citation2019). It should also be noticed that the response to obesity may differ between the subcutaneous and visceral fat depots. During the early stages of obesity (pre-obese; body mass index (BMI) of 25.0-29.9 kg/m2) BCAA-related enzymes were upregulated in the subcutaneous adipose tissue as compared to control group (BMI of 18.5-24.9 kg/m2) to cope with excessive amino acid abundance. In later phases reduced subcutaneous BCAA metabolic capacity was observed and corresponded with the severity of obesity (Biswas, Tozer, et al. Citation2020). Other data, however, showed that the lowered expression of BCAA-catabolic genes (Bcat1, Bcat2 and Bckdhb) was restricted to visceral depots, not subcutaneous adipocytes in the patients with BMI 38.5 ± 4.25 kg/m2 and 34.0 ± 3.3 kg/m2 (Pakiet et al. Citation2020; Boulet et al. Citation2015). Furthermore, a positive correlation between plasma BCAA and waist-to-hip ratio (Iwasa et al. Citation2015) supports the important role of visceral fat in metabolic disturbances. The above inconsistencies in BCAA catabolism within adipose tissue subtypes may reflect their different metabolic characteristic. Especially, the hypertrophy of visceral adipocytes is an independent prerequisite for insulin resistance, while subcutaneous tissue has lower impact on systemic insulin signaling in humans. Therefore, the higher total subcutaneous adipocyte mass (i.e., 80% of adipose tissue) and lipid overflow to other organs, can be more important in worsening glycaemic control than adipocyte morphology (Cancello et al. Citation2006).
BCAA facilitate adipocyte differentiation by regulating mTORC1/S6K1 (lineage commitment) and mTORC1/4E-BP1/peroxisome proliferator-activated receptor γ (PPARγ) (terminal steps) signaling (Estrada-Alcalde et al. Citation2017; Ricoult and Manning Citation2013), and serve as a major anaplerotic and lipogenic source. Downstream BCAA intermediates (e.g., acetyl-CoA or propionyl-CoA) may be metabolized to even- or odd-chain fatty acids (Green et al. Citation2016) or monomethyl branched-chain fatty acids (BCFA). BCFA generation occurs through the export of short-chain branched acyl moieties produced by Bckdh out of mitochondria by carnitine acetyltransferase, and their incorporation in de novo fatty acid biosynthesis, catalyzed by fatty acid synthase (Fasn) (). The production of BCFA was induced in response to cold exposure (Yoneshiro et al. Citation2019) or weight loss (Su et al. Citation2015), while the lowered amount of plasma BCFA in obese patients most likely resulted from a reduced BCAA catabolic flux (Wallace et al. Citation2018). Furthermore, adipose tissue BCFA concentrations were positively associated with skeletal muscle insulin sensitivity (Su et al. Citation2015), while plasma BCFA content inversely correlated with triacylglycerol level and insulin resistance measured by the homeostasis model assessment (HOMA) index (Pakiet et al. Citation2020).
Changes in BCAA metabolism within one organ contribute to the modifications in their utilization in the other tissues. It is suggested that increased BCAA level leads to the activation of early steps in BCAA utilization, based on the elevated urinary excretion of BCAA metabolites (isovalerylglycine, α-keto-β-methylvalerate, α-ketoisovalerate) noticed in high fat fed mice (Boulangé et al. Citation2013). Particularly, obesity-related increase in hepatic Bckdk expression and reduced Bckdh activity in ob/ob mice and Zucker fatty rats may be compensated by Bckdh complex activation in the skeletal muscle (White et al. Citation2016). Likewise, in db/db mice valine contribution to succinate formation in white adipose tissue and liver was reduced by about 60% and 20%, respectively, while it was increased in the skeletal muscle by approximately 50% (Neinast, Jang, et al. Citation2019). Therefore, muscles are a major contributor to BCAA utilization as well as a source of BCKA and other metabolites in both lean and obese states. The amino acid intermediates can be degraded up to the saturation of tricarboxylic acid (TCA) cycle. Afterwards, the overload of muscular mitochondrial capacity leads to the accumulation of short- and medium-chain acyl-CoA, the mediators of insulin resistance (White et al. Citation2016). Other data, however, implied that muscular BCAA-metabolism remains intact, despite chronic energy excess in both diet-mediated (Neinast, Jang, et al. Citation2019; Solon-Biet et al. Citation2019; She, Van Horn, et al. Citation2007; Biswas, Dao, et al. Citation2020) and genetic models of obesity (She, Van Horn, et al. Citation2007). Therefore, the above discrepancies cannot be simply explained by hyperphagia and associated higher BCAA consumption in genetic models of obesity as compared to subjects with dietary induced mass gain. In conclusion, it seems that to a certain threshold in BCAA amount, suppressed amino acid metabolism in the liver and/or adipose tissue can cause their repartition between other organs. Skeletal muscles and/or adipose tissue overflowed with BCAA are then vulnerable to mitochondrial dysfunction and insulin resistance. In patients with established type 2 diabetes this leads to a general reduction in the expression of genes responsible for BCAA degradation (Lerin et al. Citation2016; Hernández-Alvarez et al. Citation2017) proving that chronic metabolic imbalance surpasses the adaptive responses.
Mechanisms behind impaired BCAA metabolism
Systemic and tissue-specific alterations in BCAA metabolism are noticed in diverse disorders (e.g., type 1 diabetes, trauma, sepsis, coronavirus disease) (Wu et al. Citation2021; Tamanna and Mahmood Citation2014) () as a resultant of the rates of protein degradation/synthesis, tissue-specific amino acid transport, capacity for oxidative metabolism, tissue BCAA excretion to the blood as well as individual metabolic phenotype (lean, overweight, diabetic) (Adams Citation2011). However, the exact causes for an increase in BCAA content during obesity, nonalcoholic fatty liver disease (NAFLD) or type 2 diabetes (Newgard et al. Citation2009; Iwasa et al. Citation2015) are still being assessed. Most important derangements involve improper diet, decreased BCAA oxidation and induced protein breakdown (Nagao and Yamakado Citation2016). Because of the heterogeneous metabolic defects observed in patients, it is difficult to unambiguously determine the molecular background for these anomalies.
Table 1. Selected factors affecting BCAA metabolism in the blood, skeletal muscle, liver and adipose tissue.
The overabundance of other oxidizable substrates, such as carbohydrates, blunts BCAA catabolism through the phosphorylation of Bckdha as observed in vitro in L6 myotubes (Koves et al. Citation2008), rat heart (Sans, Jolly, and Harris Citation1980) and hepatocytes (Williamson, Wałajtys-Rode, and Coll Citation1979). Similarly, increased cellular glucose uptake in transgenic mice evokes the suppression of BCAA metabolizing genes, reduction in BCAA oxidation and increase in blood BCAA concentration (Herman et al. Citation2010; Shao et al. Citation2018). Mechanisms for this antagonistic cross-talk involve the inhibition of cAMP response element binding protein (CREB)-dependent transcription of KLF15, eventually resulting in the activation of mTOR (Shao et al. Citation2018) and 4E-BP1 as well as mitogen-activated protein kinase kinase (Mek)/extracellular signal-regulated kinase (Erk) pathways, that also overstimulate protein synthesis and progress to cardiac hypertrophy (Walejko et al. Citation2021). These signaling cascades underlie the relationship between chronically elevated BCAA and cardiovascular disorders in obesity. Therefore, high levels of circulating BCAA at baseline, especially valine and leucine, can predict the risk for cardiovascular diseases in lean, overweight and obese individuals (Sun et al. Citation2016; Nie et al. Citation2018; Mangge et al. Citation2016; Biswas, Tozer, et al. Citation2020). On the other hand, obesity-associated insulin resistance and inhibition of glycolysis can also disturb BCAA catabolism due to reduced pyruvate and oxaloacetate supply to replenish α-ketoglutarate for Bcat reaction. The concomitant excessive glutamate is a precursor for glutamine production () as confirmed by elevated muscular glutamine synthetase activity noticed in diabetic rats (Feng, Banner, and Max Citation1990).
BCAA compete also with fatty acids for oxidation pathways as exemplified by diminished adipose levels of Bcat2 and Bckdh transcripts and Bckdh protein content in high fat diet fed mice (Estrada-Alcalde et al. Citation2017). In the excess of lipid supply, isoleucine contribution to TCA cycle lowered in brown and white adipocytes, and skeletal muscle (by 75%, 50% and approximately 20%) (Neinast, Jang, et al. Citation2019). Similarly, primary adipocytes isolated from mice fed high fat diet showed significantly reduced rates of BCAA oxidation and their incorporation into proteins or lipids (Estrada-Alcalde et al. Citation2017). Excessive free fatty acids compete with BCAA for the same oxidation pathways and the products of their degradation (i.e., acetyl-CoA and NADH) can allosterically inhibit Bckdh activity (Tan et al. Citation2018), likely because of its structural similarity to pyruvate dehydrogenase (Pdh), a key glycolytic enzyme (Adams Citation2011). Accordingly, in healthy adults, a strong association between plasma BCAA amount and nutrient oversupply was noticed (Elshorbagy et al. Citation2018), hence, BCAA-related enzymes activity is reduced by circulating LCFA and their metabolites in the state of insulin resistance and in diabetic subjects (Ruskovska and Bernlohr Citation2013; Adams Citation2011; Tan et al. Citation2018). For instance, type 2 diabetes- and obesity-related impairment in mitochondrial function substantially affect BCAA pathway. In fact, peroxisome proliferator-activated receptor γ co-activator 1α (PGC-1α) and PGC-1β enhance the expression of multiple BCAA catabolic genes in C2C12 myotubes (Jang et al. Citation2016), while such molecular interplay is disrupted in the metabolic distress. In a mouse model of skeletal muscle-specific knockdown of mitochondrial ATP synthase, BCAA provided acetyl-CoA groups for lipogenesis (Sánchez-González et al. 2020), mirroring obesity. Eventually, lipid, glucose and BCAA oxidation in overloaded mitochondria becomes insufficient and their plasma concentrations rise (Tan et al. Citation2018). Interestingly, polyunsaturated fatty acids (PUFA), associated with low risk of type 2 diabetes, also augment plasma BCAA content, although whether it is secondary to PUFA influence on energy substrate homeostasis or occurs as a consequence of alterations in insulin response remains unknown (Karakas et al. Citation2016). Moreover, excessive LCFA may influence the redox state (Ruskovska and Bernlohr Citation2013; Adams Citation2011), which contributes to deleterious modifications in the activity of enzymes responsible for amino acid combustion. For instance, impaired detoxification of reactive oxygen/nitrogen species (ROS/RNS) in obese mice coincided with 2-3 fold exacerbation of protein carbonyls content in white adipose tissue (Grimsrud et al. Citation2007). The carbonylation can affect approximately 28% of enzymes involved in BCAA catabolism, including Bcat2, Bckdha and 3-hydroxyisobutyryl-CoA hydrolase (Hibch) (Curtis et al. Citation2012). To support these findings, healthy men consuming ∼6000 kcal/day of the common U.S. diet (50% carbohydrate, 35% fat, and 15% protein) developed insulin resistance after 2 to 3 days as a consequence of oxidative stress-induced carbonylation of various proteins and oxidation-related inactivation of Glut4 (Boden et al. Citation2015). In muscle cells, redox disorders can directly diminish the level of BCAA-related gene expression (Lerin et al. Citation2016). Therefore, oxidative stress may be the initial event connected with excessive weight gain, while pro-inflammatory processes or endoplasmic reticulum stress seem the later events in the sequence of insulin resistance development caused by imbalanced diet (Boden et al. Citation2015).
Improper BCAA metabolism may also result from the alterations in the ratio of proteolysis/protein synthesis. For instance, during the initial stage of starvation, increased proteolysis and reduced BCAA oxidation contribute to rises in circulating BCAA amount. Furthermore, Yao et al. suggested that the increase in BCAA in morbidly obese non-diabetic subjects is consequential to the improper insulin-mediated control of proteolysis rather than a defect in BCAA catabolism itself (Yao et al. Citation2019). To prove this conclusion, regained ability of insulin to inhibit proteolysis after bariatric surgery decreased the rate of protein breakdown and selectively reduced plasma BCAA concentration (Tan et al. Citation2020) concomitantly with even increased total amino acid content (Bozadjieva Kramer et al. Citation2020). Lower circulating BCAA levels after bariatric surgery were connected with an increase in BCATm and total Bckdh-E1α expression specifically in the subcutaneous and visceral adipose tissue as compared to observations before gastric bypass (She, Van Horn, et al. Citation2007; Bozadjieva Kramer et al. Citation2020). Unlike dietary manipulation (i.e., 1000 kcal/day), gastric bypass surgery normalized glycated hemoglobin (HbA1c) concentrations and evoked greater improvements in glucose tolerance in oral glucose tolerance test (OGTT) in morbidly obese patients with type 2 diabetes. Additionally, more pronounced decreases in BCAA and related metabolites were noticed after surgery as compared to a 10 kg weight loss caused by a diet regimen, suggesting that plasma amino acids can enhance glycaemic control (Laferrère et al. Citation2011). Nonetheless, neither BCAA supplementation nor PPM1K knockout impeded the beneficial effects of sleeve gastrectomy in mice (i.e., body weight loss and glucose tolerance) (Bozadjieva Kramer et al. Citation2020). Furthermore, high plasma concentrations of BCAA corresponded with low adiponectin content and elevated leptin quantity in non-diabetic population (Katagiri et al. Citation2018). Reciprocally, the disturbed adipokines secretion in obesity may affect BCAA catabolism, since diminished adiponectin release (i.e., BCAA catabolism stimulator) augmented circulating BCAA and BCKA levels (Lian et al. Citation2015). On the other hand, resistin overexpression in rat cardiomyocytes stimulated cellular [3H]-leucine uptake and its incorporation into proteins, as one of the factors linked with insulin sensitivity deterioration (Kang et al. Citation2011). It is worth mentioning that obesity is not always associated with blood BCAA upregulation, since insulin sensitive obese females had similar amino acid concentration as in the lean group (Lackey et al. Citation2013).
Discrepancies between the metabolic phenotypes can be linked with variable susceptibility to develop high fat diet-dependent obesity in population. This vulnerability depends on the regulation of intestinal lipid metabolism-related genes (Kondo et al. Citation2006), varied expression of liver proteins, potential for metabolic adaptations as well as circulating BCAA amount (Boulangé et al. Citation2013; Kondo et al. Citation2006). Particularly, based on the metabolic response of C57BL/6 mice for high fat feeding two phenotypes can be distinguished, i.e., resistance or predisposition to high fat diet-induced obesity development. The former condition characterize higher increases in the initial steps of β-oxidation and BCAA oxidation followed by upregulated ketogenesis that protects against mitochondria overload with acyl-CoA (Boulangé et al. Citation2013). Additionally, preliminary results indicated polymorphism of Bcat2 and Bckdh genes, and identified variants associated with lower circulating BCAA concentration (Serralde-Zúñiga et al. Citation2014).
BCAA as markers of insulin resistance and type 2 diabetes
Insulin acutely augments BCAA uptake and oxidation in the striated muscle as a consequence of higher abundance of amino acid transporters (e.g., LAT1) (Walker et al. Citation2014) as well as an inhibitory effect of insulin on lipolysis in white adipose tissue and hence the increased oxidation of other nutrients (Neinast, Jang, et al. Citation2019). Moreover, insulin, acting specifically on mediobasal hypothalamus (MBH), contributes to upregulated hepatic Bckdh activity (Gannaban et al. Citation2021; Sohn Citation2015), although excessive fat feeding impedes central insulin control of BCAA catabolism (Shin et al. Citation2014). Insulin exerts an additive effect with BCAA to activate mTORC1 () and protein synthesis in the skeletal muscle. However, BCAA-ingestion alone was not sufficient to stimulate muscle protein synthesis by insulin (Everman et al. Citation2016), most likely due to insulin-elicited suppression of proteolysis and deficiency of other essential amino acids. In the insulin resistant state, elevated BCAA concentration can also fail to augment the net muscle mass due to insulin inability to suppress protein degradation (She et al. Citation2013). Excessive BCAA and their derivatives can impair insulin sensitivity through the permanent stimulation of mTORC1/S6K1 signaling pathway (), which is activated either directly by leucine or by potentiating this effect by valine and isoleucine (Moberg et al. Citation2016). The resultant inhibitory phosphorylation of insulin receptor substrates (IRS1 and IRS2) impedes insulin signal transduction because of proteasomal degradation of IRS (Lynch and Adams Citation2014). However, some studies suggest a dissociation between insulin resistance and BCAA-induced mTORC1 cascade (D’Antona et al. Citation2010) or only a transient activation of S6K1 (Weickert et al. Citation2011). It is also conceivable that a synergistic effect of BCAA and saturated fatty acids on mTORC1 activity may be crucial to induce insulin signaling disorders (Newgard et al. Citation2009; Marafie et al. Citation2019).
Integrated genomic analyses in human and mouse populations confirmed a connection between glycaemic traits and the expression of BCAA catabolic genes (Moberg et al. Citation2016), being most pronounced for adipose tissue, less for the liver, while absent in the skeletal muscle (Zhou et al. Citation2019). As a consequence, plasma levels of leucine and its metabolite, KIC, were substantially elevated (+50% and +27%, respectively) in fasted obese type 2 diabetic subjects related to obese non-diabetic African-American women and correlated with HbA1c concentration (Fiehn et al. Citation2010). However, the relationship of plasma BCAA with diabetes incidence, insulin sensitivity, and insulin clearance differed with ethnicity, and was stronger in Caucasians and Hispanics nations as compared to African-Americans (Lee et al. Citation2016). Interestingly, BCAA levels are higher in men than in women (Connelly, Wolak-Dinsmore, and Dullaart Citation2017) and increases in circulating BCAA levels are paired with insulin resistance development only in women with larger central adiposity, further supporting a primary role of visceral fat in systemic BCAA equilibrium (Würtz et al. Citation2014). Disturbances in BCAA metabolism aggravated with metabolic syndrome occurrence. It was shown that omental, but not subcutaneous, Bcat2, Bckdha and Bckdhb mRNA expression was reduced in obese adult females with metabolic syndrome as compared to healthy weight-matched obese women (Lackey et al. Citation2013) and reflected in a significantly higher circulating BCAA concentrations (Serralde-Zúñiga et al. Citation2014; Allam-Ndoul et al. Citation2015; Kujala et al. Citation2016). Apart from glucose intolerance, BCAA affect also oxidative balance, mitochondrial function and cellular survival, all of which may promote diabetes (Siddik and Shin Citation2019). Recent study conducted on 1,321 individuals with different risks for type 2 diabetes (i.e., from ADDITION-PRO cohort) did not provide genetic evidence for the causative role of BCAA in the development of insulin resistance, but suggested that insulin resistance itself can evoke higher plasma fasting BCAA concentrations (Mahendran et al. Citation2017). Nevertheless, the elevation in plasma BCAA and BCKA levels predicted type 2 diabetes development in various study cohorts and preceded the disease by over 10 years (Long et al. Citation2020; Arany and Neinast Citation2018; Wang et al. Citation2011). Therefore, BCAA are valuable biomarkers for early detection and diagnosis of insulin resistance in non-diabetic obese patients and strong indicators of the later risk of type 2 diabetes (Zhao et al. Citation2016) as well as potential biomarkers in the diagnosis of diabetes complications (Del Coco et al. Citation2019). In the opposite to these observations, in middle-aged individuals from East Asian and Western countries that participated in International Study of Macro-/Micronutrients and Blood Pressure (INTERMAP) higher BCAA intake was associated with reduced prevalence of overweight or obesity (Qin et al. Citation2011).
BCAA can also serve as markers for increased risk of impaired glucose tolerance or type 2 diabetes mellitus development after pregnancy complicated by gestational diabetes mellitus (GDM). In a longitudinal study, derangements in leucine and isoleucine metabolism were present in the first and second trimester of pregnancy, prior GDM development. There was also a positive relationship between BCAA levels and several markers of energy control (i.e., triacylglycerol, glycerol and leptin) as well as a negative relationship with high-density lipoprotein (HDL) concentration (Andersson-Hall et al. Citation2018). The meta-analysis study also indicated a potential role of plasma BCAA for the early diagnosis of GDM (Zhao, Wang et al. Citation2019). However, metabolomics data showed a decrease in BCAA concentration throughout pregnancy (Zhao, Li, et al. Citation2019; Allman et al. Citation2020; Allman et al. Citation2021) accompanied by no changes in insulin resistance (Allman et al. Citation2020) or its aggravation in women with obesity (Allman et al. Citation2021). Such observations highlight that higher levels of BCAA alone are not sufficient to trigger insulin resistance and challenge a direct link between blood BCAA content and the risk of insulin signal impairment in pregnant women. Some data associated leucine, but not the total BCAA, with obesity-related cancer risk (Tobias et al. Citation2020). Pancreatic cancer cells have elevated BCAA uptake through the overexpression of amino acid carriers (i.e., Slc1a5, Slc3a2, Slc7a5), and hence BCAA serve as a carbon source in lipid biosynthesis (Lee et al. Citation2019), presumably in a hypoxia-dependent manner (Zhang et al. 2021). BCAA-lowering approaches (e.g., nutritional or pharmacological) are potentially salutary in terms of obesity-complications. However, manipulating dietary energy substrates merits careful investigation because apart from caloric provision nutrients are also important signaling molecules.
Dietary interventions aimed at BCAA regulation and their impact on systemic and tissue-specific insulin sensitivity
Inappropriate diet has long been referred a major contributor to the escalation of obesity-dependent insulin resistance. Studies focused on protein intake modulation demonstrated that low protein diet significantly reduced plasma BCAA and BCKA levels (Zhou et al. Citation2019), allowed to induce glucose and fatty acids oxidation (Maida et al. Citation2016) as well as improved whole-body and tissue-specific glucose tolerance (i.e., indicated by a higher pAkt (Thr308)/Akt ratio in skeletal muscle, liver and white adipose tissue) in ob/ob mice (Zhou et al. Citation2019; Maida et al. Citation2016). Conversely, infusion of amino acids mixture to healthy male volunteers had deleterious consequences on skeletal muscle insulin sensitivity due to suppression of glucose transport with a significant decline in glycogen biosynthesis (Krebs et al. Citation2002). Over the years, the manipulation of total or individual BCAA intake, instead of protein modulation, gained more attention.
BCAA deprivation
Deprivation of individual or total BCAA profoundly impacts energy homeostasis and the observed results are somewhat reminiscent of dietary BCAA addition (). Short-term switch from a standard diet to leucine- or BCAA-deprived diet reduced hepatic expression of genes responsible for the synthesis of fatty acids and triacylglycerol, such as Srebp-1c, ATP citrate lyase (Acl), Fasn, stearoyl-CoA desaturase (Scd), glucose-6-phosphate dehydrogenase (G6pd), acetyl-CoA carboxylase (Acc) and malate dehydrogenase (Me) in mice (Guo and Cavener Citation2007; Solon-Biet et al. Citation2019). Moreover, a diminished BCAA intake increased preference for carbohydrate oxidation as measured by elevated respiratory quotient and induced Pepck expression (i.e., the enzyme for the first committed step in gluconeogenesis) in murine liver (Solon-Biet et al. Citation2019). Several molecular and cellular adjustments were developed also in the adipose tissue of dietary leucine-deprived mice. These changes encompassed increased lipolysis (i.e., activated cAMP/PKA/phosphorylated hormone sensitive lipase (pHSL) and PPARα pathways) and suppressed lipogenesis in white adipocytes. In brown adipose tissue upregulated fatty acid transport, β-oxidation and thermogenesis were documented (Cheng et al. Citation2010). Consistently, leucine, isoleucine or valine deprivation in mice increased energy expenditure followed by a reduced overall accumulation of adipose tissue independently of food intake (Wanders et al. Citation2015; Du et al. Citation2012). A remodeling of white adipose tissue was based on enhanced uncoupling protein 3 (UCP3)-mediated mitochondrial uncoupling of oxidative phosphorylation, but also on the activation of lipogenic genes (i.e., Acaca and Fasn) (Wanders et al. Citation2015). With regards to the insulin signaling, short-term dietary reduction in BCAA resulted in advantageous effect in patients with type 2 diabetes (Karusheva et al. Citation2019). The molecular background included increased phosphorylation of insulin receptor (Tyr1150/1151) and Akt (Ser473) based on data from HepG2 cells, primary mouse hepatocytes and C2C12 myotubes. Similarly, lean mice on a diet devoid of either amino acid expressed improved systemic glucose control connected with higher hepatic phosphorylation of insulin receptor (Tyr1150/1151), Akt (Ser473) and 5’AMP-activated protein kinase (AMPK; Thr172), diminished phosphorylation of p70 S6K1 (Thr389) and S6 (Ser235/236), reduced food intake, and diminished postprandial glucose levels in the liver. Consequently, already 1 day of valine or isoleucine deficiency accelerated a decrease in blood glucose level during insulin tolerance test (ITT) in db/db mice (Xiao et al. Citation2014). Despite functional similarities between total BCAA, the latest report showed that beneficial effects of all BCAA deprivation were most strongly reproduced by isoleucine depletion, while only minor changes were noticed by dietary leucine reduction () (Yu et al. Citation2021).
Table 2. Metabolic characteristic of subjects undergone dietary BCAA deprivation or supplementation.
When coupled with high-fat provision, long-term dietary intervention (i.e., low BCAA administration) protected obese mice from insulin resistance (i.e., based on glucose and insulin tolerance tests). It was accompanied by decreased mass gain, reduced lipogenic genes (i.e., Fasn, Scd1) expression in the liver and lowered hepatic lipid droplet size as well as increased energy expenditure. Importantly, these outcomes were achieved without changes in animal activity (Cummings et al. Citation2018). Nonetheless, in Zucker fatty rats the impact of low dietary BCAA on systemic insulin sensitivity was negligible, although glucose tolerance increased in the skeletal muscle. Particularly, BCAA reduction promoted muscular non-oxidative glucose metabolism and increased reliance on fatty acid oxidation (White et al. Citation2016). Moreover, diminution of BCAA improved mitochondrial export of acyl-glycine and elevated urinary excretion of these adducts, which indicates that reduced glycine pool due to excessive BCAA in obesity contributes to acyl-CoA overabundance (White et al. Citation2016). Similarly, BCAA-restricted diet, independently of Bckdh activity, entailed a shift in substrate utilization that favored fatty acid oxidation instead of glucose metabolism with a concomitant decrease in triacylglycerol amount in the heart of Zucker fatty rats () (McGarrah et al. 2020). The above data imply that increased BCAA level in obesity can lead to cardiac dysfunction due to alterations in nutrients use. Nonetheless, diminished BCAA supply did not modulate cardiac insulin signaling in obese rats (McGarrah et al. 2020). Furthermore, BCAA restriction selectively lowered acyl-CoA, but not acylcarnitine, level in the muscle, therefore indicating that BCAA do not modify fatty acid uptake or Cpt1-dependent mitochondrial acylcarnitine transport, instead they inhibit inter-mitochondrial acyl-CoA oxidation (White et al. Citation2016). These conclusions are, however, inconsistent with a well-known acylcarnitine overabundance in skeletal muscle of obese animals and lack of alterations in circulating non-esterified fatty acids (NEFA) and triacylglycerol content in BCAA-treated models (Li et al. Citation2013; Harris et al. Citation2017) as well as with the proposed 3-hydroxyisobutyrate (3-HIB)-mediated paracrine stimulation of fatty acid transport into skeletal muscle in mice (Jang et al. Citation2016). It appears that different metabolic background in the above models, evokes specific adaptive mechanisms to be triggered. Moreover, the effects of BCAA deprivation on lean mass preservation during weight loss are not yet addressed.
A crucial role in sensing dietary BCAA undernutrition is ascribed to a serine/threonine kinase termed general control nonderepressible 2 (Gcn2). In response to amino acid deficiency, cellular uncharged tRNA bind with Gcn2 inducing its structural changes and activation, which in turn phosphorylates eukaryotic initiation factor 2α (eIF2α) and reduces global translation program to preserve energy (Averous et al. Citation2016). Simultaneously, Gcn2 inhibits mTOR/S6K1 cascade in the liver to improve insulin sensitivity () (Xiao et al. Citation2011), while Gcn-/- mice fail to augment glucose clearance in response to dietary valine deprivation (Xiao et al. Citation2014). Literature data implicated Gcn2 also in the regulation of lipid metabolism, since Gcn-/- mice were unable to repress the expression of lipogenic genes (i.e., Srebp-1c and Fasn), thus progressing to liver steatosis (Guo and Cavener Citation2007). Additionally, the low protein diet-induced activation of both Gcn2 and PGC-1α subsequently triggered fibroblast growth factor 21 (FGF21) release and adaptive changes in the postprandial oxidation of energy substrates (Chalvon-Demersay et al. Citation2019; Cornu et al. Citation2014; Fontana et al. Citation2016). FGF21 is a hepatokine considered as a signal of improved metabolic homeostasis due to, e.g., stimulation of AMPK pathway, beiging of white adipose tissue and higher energy expenditure (Wanders et al. Citation2015) as well as upregulation of adiponectin (Fontana et al. Citation2016). The dynamic regulation of FGF21 was illustrated by an entirely regressed levels of the hormone after switching mice from low protein diet to a standard chow (Maida et al. Citation2016). Recent data, however, questioned the ability of BCAA to influence the FGF21 synthesis, restricting this effect to protein diminution (Fontana et al. Citation2016). Nevertheless, there was a transient increase in FGF21 level observed 12 days after a switch from Western diet (high-fat, high-sugar) to Western low BCAA diet. In contrast to this acute phase, greater energy expenditure and weight loss despite, unchanged caloric intake, was noticed after ∼12 weeks consumption of Western diet deprived in BCAA, independently of FGF21 secretion (Cummings et al. Citation2018). The increased FGF21 content therefore reflected amino acid imbalance, although the stable expression of downstream target genes in white adipose tissue (i.e., FGF21 co-receptor Fgfr1c and Klotho β-like protein Klb) excluded the possibility of FGF21 resistance (Solon-Biet et al. Citation2019). The non-crucial role of BCAA in FGF21 control was confirmed by its upregulation observed in obese mice fed protein-reduced diet repleted with BCAA (Maida et al. Citation2017). It appears that BCAA deprivation may mediate only temporal FGF21 release, and to a smaller extent than as a part of an amino acid mixture. Higher level of FGF21 after BCAA restriction was attributed to improved mitochondrial efficiency in white adipose tissue (Karusheva et al. Citation2019) as well as coincided with elevated Akt phosphorylation (Ser473) in the liver (Wanders et al. Citation2015). Interestingly, FGF21 promoted an increase in energy expenditure in mice fed low-isoleucine, but not low-valine, diet (Yu et al. Citation2021). Since BCAA depletion recapitulated the beneficial effects of protein-depleted diet (i.e., by improving glucose tolerance and body composition in mice and humans) despite lack of alterations in FGF21 content and without any manipulations in the dietary carbohydrate:protein ratio (Fontana et al. Citation2016), other regulatory mechanisms must be involved.
BCAA are associated with gut flora metabolism. To support this connection, mice receiving fecal microbiome from the obese twins displayed substantial increases in serum BCAA concentrations (Ridaura et al. Citation2013). Among human gut microbial species mainly Prevotella, Streptococcus and Bacteroides biosynthesize BCAA (Pedersen et al. Citation2016; Yue et al. Citation2019). It was shown that gut microbiota dysbiosis features obesity-related metabolic dysfunctions, including type 2 diabetes (Han and Lin Citation2014). In insulin resistant patients, the higher content of circulating BCAA was connected with enriched microbiome enzymes for BCAA synthesis (P. copri or B. vulgatus) and diminished level of genes encoding bacterial inward BCAA transporters (B. crossotus and E. siraeum) (Pedersen et al. Citation2016). However, this differences explained only 2.4–3.1% of the variance in serum BCAA levels, and 1% was explained by gut species variety (Pedersen et al. Citation2016). Although low LAT1 expression was confirmed in the colon (Hayase et al. Citation2017), further physiological investigations are needed to establish mechanisms for intestinal BCAA transfer to the bloodstream as well as the intestinal segment wherein this transport takes place. Interestingly, intermittent BCAA deprivation (Wei et al. Citation2018) and short-term BCAA restriction modified the composition of gut microbiome toward elevated Bacteroides and reduced Firmicutes phyla abundance (Karusheva et al. Citation2019). Especially, Bacteroides were associated with obesity and signals of insulin resistance in humans (Dewulf et al. Citation2013), while increased Prevotella/Bacteroides ratio corresponded with improved glucose tolerance (Kovatcheva-Datchary et al. Citation2015). Moreover, the altered composition of microbial species may correspond with changes in the production of acetate, propionate or butyrate by microbiota that in turn can regulate the susceptibility to diet-induced insulin resistance (Lin et al. Citation2012).
BCAA supplementation
BCAA administration affects the expression of multiple genes, including those involved in protein synthesis and energy metabolism (Drummond et al. Citation2017). At the cellular level, C2C12 myotubes treated with leucine (2 mM for 24 h) had substantially elevated indicators of mitochondrial function, lipogenesis and fatty acid oxidation (Rivera et al. Citation2020). What is more, leucine oversupply promoted muscle contractility (Martin et al. Citation2017), insulin-stimulated glucose uptake in rat muscle cells (Liu et al. Citation2014) and glycogen synthesis in L6 myotubes as a consequence of glycogen synthase kinase-3 inhibition (Peyrollier et al. 2000). However, 4 times higher than normal doses of BCAA triggered a decrease in glycogen content and lowered Akt phosphorylation (Ser473) under basal and insulin-stimulated conditions (Crossland et al. Citation2020). It seems that in the skeletal muscle concentration-dependent effects of BCAA overabundance are observed, so that when BCAA amount exceeds the capacity of cellular adaptive mechanisms, the negative outcomes become visible. Regarding HepG2 cells, in the presence of increased BCAA concentrations, transcription factors linking glucose and lipid homeostasis were upregulated (i.e., ChREBP, Srebp-1c, LXRα), which augment the expression of lipogenic enzymes, stimulate glucose-sensing system (i.e., increased Glut2 and glucokinase expression) and elevate glucose uptake through myostatin-dependent AMPK modulation (Zarfeshani, Ngo, and Sheppard Citation2014; Higuchi et al. Citation2011). The above data suggest that abundance in BCAA may reflect in NAFLD-like phenotype development in the liver.
Notwithstanding the evidence toward increased BCAA level in the course of metabolic disorders, BCAA supplementation can improve metabolic characteristic of the subject. Several factors, however, modulate the BCAA-related effects, including age or dietary background of the subject. To support this, neither mitochondrial biogenesis nor oxidative defence were improved in young mice in response to BCAA provision, although older subjects exhibited beneficial adaptations (D’Antona et al. Citation2010) (). Additionally, animal sensitivity to exogenous BCAA depends on the dietary protein levels. Specifically, enrichment of low protein diet with BCAA promoted energy storage in both lean and fat mass in mice, while the above effect did not occur when normal protein provision was secured (Mu et al. Citation2018). The effects of BCAA addition are also more pronounced in obese subjects than in lean rodents, wherein BCAA treatment did not affect body weight, glucose homeostasis, insulin sensitivity and blood lipid profile (Zhang et al. Citation2016; Li et al. Citation2013). In contrast, in db/db mice BCAA suppressed hepatic and adipose tissue inflammation (Terakura et al. Citation2012) as well as diminished circulating level of pro-inflammatory adipokines (i.e., TNF-α, IL-6 and leptin) (Zhang et al. Citation2007; Jiao et al. Citation2016). Other metabolic improvements in obese/diabetic mice encompassed lowered weight gain, alleviated triacylglycerol storage in the skeletal muscle, liver and white adipose tissue, diminished hepatic expression of lipogenic enzymes and restored level of lipolysis-inducing proteins in white adipose tissue (Arakawa et al. Citation2011; Terakura et al. Citation2012; Jiao et al. Citation2016; Li et al. Citation2013; Macotela et al. Citation2011; Binder et al. Citation2014) as well as enhanced thermogenic program in brown adipocytes (Jiao et al. Citation2016). Since plasma free fatty acid content remained stable, augmented oxidative decomposition of lipids might occur after BCAA supply through PPARα-mediated mechanisms (Arakawa et al. Citation2011). It is also plausible that higher BCAA degradation rates provided acetyl-CoA pool for lipid synthesis and, at the same time, inhibited Pdh activity, what augmented fatty acid oxidation in order to maintain the net energy balance. Collectively, BCAA increased energy expenditure, ultimately reversing the increase in adipocyte size and mitigated hepatic steatosis observed in obese mice. In Sprague-Dawley rats, administration of leucine alleviated oxidative stress, hepatic gluconeogenesis and protected from insulin resistance, despite the augmented adiposity (Li et al. Citation2013). Possibly, adipocyte hyperplasia was caused by elevated glucose uptake, which reduced circulating carbohydrate levels in animals. Importantly, the positive impact of BCAA provision on mitochondrial biogenesis in the skeletal muscle depends on stage of obesity and was observed in the late hyperglycemic phase in rats (32 weeks). During early hyperinsulineamic phase (24 weeks) leucine contributed to mitochondrial dysfunction and incomplete oxidation of BCAA and lipid species (Liu, Li et al. Citation2017). Dietary interventions based on leucine, isoleucine or BCAA mixture supplementation did not impede insulin signal transmission and even improvements in glucose tolerance were noticed (Zhang et al. Citation2007; Jiao et al. Citation2016; Ma et al. Citation2020), despite sustained activation of mTORC1/p70 S6K pathway (Macotela et al. Citation2011). Moreover, based on twin-pair study, higher BCAA ingestion was related to attenuated insulin resistance, inflammation, blood pressure and adiposity-related metabolites, independently of genetic and environmental factors (Jennings et al. Citation2016). Longitudinal BCAA-enriched diet consumption improved insulin sensitivity also in individuals with chronic liver disease (Kawaguchi et al. Citation2008), nonalcoholic steatohepatitis-related cirrhosis (Miyake et al. Citation2012) or in elderly subjects with type 2 diabetes (Solerte et al. 2008). However, the beneficial effects were not a rule for BCAA addition, since in genetic model of obesity, repletion of BCAA combined with protein deprivation had no effect on body mass in ob/ob mice (Zhou et al. Citation2019) or glucose control (Maida et al. Citation2017). Likewise, a diet enriched with whey proteins (i.e., dietary supplement with high content of BCAA) did not change glucose homeostasis in obese women (Piccolo et al. Citation2015) and mice (Roquetto et al. Citation2020). Altogether, the effects of BCAA seem more pronounced during dietary interventions than in genetically obese animals, presumably because of initially lower BCAA levels. Furthermore, whether caloric contribution from BCAA or modifications in cellular signaling cascades underlie insulin resistance modulation may differ between genetic and dietary models of BCAA overabundance.
In contrast to the above results, other data revealed that lean mice supplied with exogenous BCAA were characterized by increased expression of pro-inflammatory cytokines (Mu et al. Citation2018), elevated hepatic level of triacylglycerol, increased fat score and plasma α-keto-δ-(NGNG-dimethylguanidino)-valeric acid (DMGV) amount, a bio-marker for NAFLD (Solon-Biet et al. Citation2019). The observed BCAA-mediated fatty acid accumulation can occur through de novo production (Green et al. Citation2016; Rivera et al. Citation2020) or through Bckdk-related Acl phosphorylation (White et al. Citation2018). Specifically, Acl converts citrate to acetyl-CoA, a precursor for malonyl-CoA (), which is Cpt-1 inhibitor and a substrate for lipid synthesis (Foster Citation2012). Additionally, even reduced body weight of high fat-fed obese mice supplemented with BCAA did not protect from NAFLD development. The underlying mechanisms included exacerbated hepatic lipotoxicity as a result of reduced Srebp-1c and its target gene expressions (i.e., Acc1, Fasn, Scd1, Elovl6, Dgat1) in mTOR-dependent manner (Zhang et al. Citation2016). Hence, the downregulation of lipogenesis rates precluded excessive fatty acids neutralization within triacylglycerol pool in the liver and lipotoxic fractions may be formed. Hyperlipidemia was also exacerbated by abnormal adipocyte lipolysis that aggravated lipotoxicity in the liver and resulted in deteriorated whole-body insulin sensitivity (Zhang et al. Citation2016). Conceivably, BCAA-dependent suppression of insulin-mediated anti-lipolytic effect intensified hyperlipidemia. AMPKα2 phosphorylation and subsequent phosphodiesterases inhibition as well as elevated hormone sensitive lipase (Hsl) phosphorylation (Ser660), but no changes in Atgl level, contributed to these effects (Zhang et al. Citation2016). In BCAA-treated mice insulin failed to increase lipogenesis and reduce gluconeogenesis also through an augmented Akt2 ubiquitin-dependent degradation (Zhao et al. Citation2020). Interestingly, lipolysis was not the main reason for the development of NAFLD-like phenotype, since acipimox did not alleviate the effects caused by BCAA oversupply. Nonetheless, inhibition of lipolysis alleviated BCAA-dependent hyperglycemia, weight loss and enhanced insulin sensitivity (Zhang et al. Citation2016). BCAA-mediated liver damage was confirmed by upregulated plasma alanine transaminase (Alt) and aspartate transaminase (Ast) content (Solon-Biet et al. Citation2019), suppressed hepatic autophagy, augmented oxidative stress, inflammatory responses and apoptosis of hepatocytes (Zhang et al. Citation2016). Further inquiry using a diet-induced mouse model of NAFLD, showed that BCAA-related dysregulation in TCA cycle significantly contributed to mitochondrial dysfunction and insulin resistance development (Sunny et al. Citation2015; Newgard et al. Citation2009). Moreover, BCAA administration did not avert diabetes in patients with cirrhosis and instead higher plasma glucose concentration were noticed (Nakanishi et al. Citation2019). The impaired hepatic buffer function also contributed to muscle and kidney lipid infiltration and accumulation (Zhao et al. Citation2020). The opposite consequences of BCAA treatment, i.e., improved (Jiao et al. Citation2016) or deteriorated (Zhang et al. Citation2016; Zhao et al. Citation2020) metabolic phenotype in obese rodents, uncover the flexibility of metabolic processes and high variety of adaptations depending on the used model, including dose and type of dietary intervention (leucine or all BCAA administration), duration of diet administration and dietary background. Moreover, in Sprague-Dawley rats intrahypothalamic BCAA infusions declined circulating glucose level and required higher glucose delivery during euglycemic pancreatic clamp to maintain euglycemia. More interestingly, when lipid provision preceded the above intervention, the effects of leucine were abolished. Therefore, BCAA may centrally control hepatic glucose generation, while fatty acids disrupt this effect (Arrieta-Cruz, Su, and Gutiérrez-Juárez Citation2016).
BCAA can also influence other amino acid balance and, as a consequence, the processes regulated by them. In this regard, high rates of BCAA flux into peripheral tissues, particularly skeletal muscle, provides nitrogen donors for glutamate synthesis, which in turn may stimulate the transamination of pyruvate to alanine and its subsequent transport to hepatocytes (). Alanine is a highly gluconeogenic amino acid (Newgard et al. Citation2009) connected with an increased risk of type 2 diabetes incidence (Vangipurapu et al. Citation2019). Additionally, the excess BCAA-derived nitrogen groups entering pyruvate-alanine cycle are linked with lowered glycine concentration (White et al. Citation2020), while an increase in glycine content after BCAA restriction may have favorable impact on insulin sensitivity (White et al. Citation2016; Vangipurapu et al. Citation2019). However, a therapeutic strategy based on exogenous glycine supplementation was not sufficient to neutralize the deleterious consequences of BCAA overabundance in obese animals (White et al. Citation2020).
Despite the fact that higher dietary BCAA intake elevates the risk of type 2 diabetes (Isanejad et al. Citation2017; Zheng et al. Citation2016), BCAA can improve the effects of anti-diabetic therapy. It is evident that obesity, insulin resistance and type 2 diabetes are associated with decreased activity of AMPK/sirtuin 1 (Sirt1) axis (Saha et al. Citation2014) and hence PGC-1α, i.e., proteins responsible for sensing cellular energy status and modulation of mitochondrial function (Ruderman et al. Citation2010; Supruniuk, Mikłosz, and Chabowski Citation2020). Leucine supplementation enabled Sirt1 activation in the presence of lower levels of NAD+ (Bruckbauer and Zemel Citation2014) what strengthened the efficacy of 6-weeks metformin treatment in obese mice. Furthermore, co-stimulation with leucine enabled to reduce metformin dose by 83% without the deleterious loss of its efficacy (L. Fu et al. Citation2015). Importantly, metformin dose reduction can minimize adverse side-effects observed in humans, mostly originated from the gastrointestinal tract (Brietzke Citation2015). Similarly, synergistic influence of metformin, resveratrol and leucine metabolite, β-hydroxy-β-methyl-butyrate (HMB), on the improvement of insulin sensitivity was noticed in db/db mice (Bruckbauer and Zemel Citation2013).
Energy balance and appetite responses can be homeostatically controlled by central amino acid sensing mechanisms and neurotransmission modulation. According to the ‘protein leverage’ hypothesis, higher energy intake can be evoked by a protein-restricted diet since the central control mechanisms seek to meet a hypothetical threshold level for protein delivery (Simpson and Raubenheimer Citation2005). Whenever elevated fuel intake is coupled with increased energy expenditure, metabolic homeostasis remains preserved. In the opposite situation, doubled BCAA intake-induced hyperphagia was the primary cause for BCAA-induced obesity and shortened lifespan in mice (Solon-Biet et al. Citation2019). The augmented food intake after elevated BCAA provision can be the consequence of BCAA and non-BCAA amino acids competition for the same neuronal carriers, such as LAT1. It is therefore likeable that the compensatory increase in food intake was related with the insufficiency of non-BCAA amino acids in the brain, instead of BCAA overabundance (Solon-Biet et al. Citation2019). For instance, hyperphagia developed in response to a low protein-diet even after the normalization of BCAA content (Laeger et al. Citation2014). Importantly, reduced aromatic amino acid (i.e., tryptophan, phenylalanine, tyrosine) transport to the brain affects neurotransmission, including diminished serotonin secretion from dorsal raphe nuclei (Solon-Biet et al. Citation2019). In fact, treatment with serotonin agonists downregulated food intake, while the reciprocal effect was demonstrated for serotonin inhibitors (Halford et al. Citation2007). In line with these findings, the administration of selective serotonin reuptake inhibitor (SSRI), fluoxetine, to hyperphagic mice overfed with BCAA diminished their appetite. Additionally, caloric restriction or BCAA co-supplementation with tryptophan or threonine significantly suppressed hyperphagia (Solon-Biet et al. Citation2019), while tryptophan, but not serotonin, provision also minimized anxiety-like behaviors (Coppola et al. Citation2013). Contrary to the above observations, most literature data indicate either no changes or an appetite-reducing effect of BCAA () by their impact on central and hormonal mediators of food intake. In line with this, adult women consuming nutrition bars with greater leucine content were characterized by increased food efficiency and reduced hunger (Bolster et al. Citation2018). Interestingly, in rodents the differences between food intake were noticed only for simultaneous high-fat and high-BCAA provision, not when animals were maintained on a standard diet (Newgard et al. Citation2009). One of the amino acid-sensing centers is localized within the mediobasal hypothalamus. Leucine-mediated upregulation of mTOR cascade and AMPK inhibition within the neurons governs the interplay between anorexigenic proopiomelanocortin, and orexigenic neuropeptide Y and agouti-related peptide (AgRP) (Watterson et al. Citation2013), hence contributing to BCAA-mediated suppression of food intake. Moreover, p70 S6K1 and Erk1/2 axes have been revealed to mediate leucine sensing to regulate feeding. Thereafter, elevated leucine availability activated neurocircuit engaging proopiomelanocortin neurons of the mediobasal hypothalamus, oxytocin neurons of the paraventricular hypothalamus and neurons within the brainstem nucleus of the solitary tract, ultimately reducing food consumption. Additionally, it was proposed that the long-term consequences of leucine supplementation would encompass a diminished frequency of meal intake by the activation of transcription factor CREB (Blouet et al. Citation2009). It is also evident that the degree of dietary BCAA manipulation must be sufficient to overcome neuronal BCAA balance in order to affect appetite. Particularly, ventricular injections of high doses of leucine considerably declined the consumption of standard diet, while reduced dietary leucine intake was not sufficient to induce hyperphagia in Sprague-Dawley rats (Laeger et al. 2014). In this regard, the diet-elicited changes in plasma leucine are seven-fold higher than those in the cerebrospinal fluid (Blouet et al. Citation2009). Because the above-mentioned data were strongly impacted by several factors, including species-dependent metabolic regulation, experimental conditions (i.e., duration of diet, the relative quantity of BCAA to non-BCAA, concentration of other nutrients), central or dietary BCAA administration, the physiological relevance of BCAA in terms of appetite control needs further investigation.
BCAA levels are connected with circulating leptin concentrations and its action. Sprague-Dawley rats undergone 16 weeks of dietary leucine and high fat co-administration were characterized by restored leptin sensitivity based on reduced serum leptin levels, without food intake or body weight differences. These animals had diminished expression of leptin receptor in the hypothalamus and adipose tissue, elevated adipocyte expression of downstream leptin receptor targets, such as Janus kinase 2 (JAK2) and signal transducer and activator of transcription 3 (Stat3), and diminished leptin inhibitor content namely suppressor of cytokine signaling 3 (Socs3) (Yuan et al. Citation2015). Accordingly, exogenous leucine supplementation to patients with genetic leptin deletion reduced appetite and corrected metabolic parameters (Howard and Flier Citation2006) as well as leucine supplementation to obese mice exacerbated leptin-related responses (Binder et al. Citation2014). The lower weight gain due to satiety and reduced hepatic lipogenesis can be noticed already after 1 week upon a diet transition to leucine-enriched meals in the initially high fat fed C57BL/6 mice (Freudenberg, Petzke, and Klaus Citation2013). In contrast, hyperphagia was leptin-independent considering the unchanged expression of hypothalamic genes mediating leptin signal (i.e., Lepr, Socs3) in mice housed on a high BCAA diet (Solon-Biet et al. Citation2019). Furthermore, BCAA-elicited incretins release, i.e., glucagon-like peptide-1 (GLP-1), gastric inhibitory polypeptide (GIP) and ghrelin in the gastrointestinal tract, presumably through mTORC1 cascade stimulation, can affect food intake and insulin secretion (Li et al. Citation2018; Zhou et al. Citation2015; Chen and Reimer Citation2009). Leucine supplementation influenced also the endocrine function of adipocytes, including increased circulating levels of adiponectin. Lack of a paralleled improvement in insulin sensitivity (Torres-Leal et al. Citation2011) may be related with the adiponectin isoform being induced (Pajvani et al. Citation2003), although it warrants further investigation.
The heart is another organ wherein metabolic and functional changes were noticed upon BCAA administration. The majority of cardiac BCKA is sequestrated from TCA cycle and undergo reamination, presumably due to low SLC25a44 abundance (Walejko et al. Citation2021). Failing heart exhibited reduced expression of BCAA-metabolizing genes (i.e., 25 of 46 genes tested), with an increase in KIC, KIV and KMV, but not BCAA content (Sun et al. Citation2016). In turn, BCAA intermediates strongly affect energy substrate preference in the heart by reducing the expression of genes responsible for glucose oxidation, and inducing the level of glycolysis-related enzymes and those involved in fatty acid metabolism (i.e., CD36/SR-B2, Fabp3, Acsl, Cpt1b, Hadhb, Mcad). Because of upregulated fatty acid oxidation-related lipid peroxidation (i.e., increased amount of superoxide, MDA and 4-HNE), neonatal rat ventricular myocytes (NRVM) were more vulnerable to hypoxia/reoxygenation injury in the presence of high BCAA concentrations (Li, Xiong, et al. Citation2020). The molecular signaling involved BCAA-, BCKA- and their derivatives-dependent inactivation of Gcn2 that promoted the activation of transcription factor 6 (ATF6)/PPARα pathway in cardiomyocytes (Li, Xiong, et al. Citation2020). Importantly, BCAA-induced oxidative stress, cardiomyocyte apoptosis and heart dysfunction in mice was averted by the inhibition of fatty acid oxidation and by PPARα downregulation (Li, Xiong, et al. Citation2020). On the contrary, mice receiving BCAA prior ischemia/reperfusion injury induction exhibited diminished infarct size and improved cell viability. The mTOR signaling, but not PI3K/Akt/glycogen synthase kinase 3β (GSK3β) pathway, appeared to mediate this cytoprotective consequences of BCAA (Satomi et al. Citation2020). The precondition effect of BCAA may be therefore crucial to achieve the protective influence of BCAA on cardiac function.
Pharmacological or genetic interventions affecting BCAA catabolism
The metabolism and concentration of amino acids can be changed by several drugs as a part of their hypoglycemic action. PPARγ is a major regulator of BCAA metabolism in white and brown adipocytes in rats (Hsiao et al. Citation2011) and humans (Sears et al. Citation2009), since it triggers BCAA shift into triacylglycerol formation and induces the expression of Bcat2 and Bckdh. On the other hand, adipose-specific PPARγ knockdown caused lipodystrophy, impeded glucose tolerance and elevated serum BCAA concentration in mice (Blanchard et al. Citation2018). These observations align with induced Bcat and Bckdh expression in adipocytes in response to thiazolidinediones (TZD), PPARγ ligands (Lackey et al. Citation2013; Blanchard et al. Citation2018), followed by a reduced mTOR-dependent inhibitory phosphorylation of IRS1 (Ser636/639) in soleus muscle as well as alleviated whole-body insulin resistance (Blanchard et al. Citation2018).
Metformin, one of the most prevalent therapeutics for type 2 diabetes, also declines BCAA level (Walford et al. Citation2013) as well as activates mTORC1 inhibitor, i.e., AMPK (Howell et al. Citation2017). Recently, the hypoglycemic and anti-hyperlipidemic properties of another anti-diabetic drug, berberine (Feng et al. Citation2019), were connected with its impact on BCAA metabolism. Particularly, the reduced abundance of gut BCAA-producing bacteria and exacerbated BCAA catabolism in the liver and white adipose tissue of high fat diet-fed mice were noticed (Yue et al. Citation2019). At least partially, the above effect depended on the activation of AMPK/PGC-1α pathway (Li, Wang, et al. Citation2020) that stimulated BCAA catabolism (Biswas, Duffley, and Pulinilkunnil Citation2019). Similarly, sitagliptin, approved in about 130 counties for the treatment of adult patients with type 2 diabetes (Scott Citation2017), reduced plasma valine level (Liao et al. Citation2019). Independently of drug’s mechanism of action, the BCAA-lowering effect accompanied a therapy of obese and diabetic patients also using alogliptin, a dipeptidyl peptidase-4 inhibitor (Iwasa et al. Citation2015) or glipizide, a sulfonylurea medication (Walford et al. Citation2013). Empagliflozin, a member of antidiabetic sodium-glucose co-transporter 2 inhibitors, may restore derangements in BCAA oxidation in parallel with an increase in ketone bodies, so that providing energy substrates for the heart and preventing from heart failure in diabetic patients (Kappel et al. Citation2017). Additionally, clofibrate, a PPARα activator used for hyperlipidemia treatment, is another potent stimulator of hepatic Bckdh and BCAA oxidation (Kobayashi et al. Citation2002). Surprisingly, a single dose of clofibrate impaired glucose tolerance in rats, while this effect was restrained by BCAA supplementation (Kadota et al. Citation2012). Therefore, it is possible that the opposite consequences in terms of macronutrients metabolism and insulin resistance are triggered by long- and short-term BCAA decrease.
BCAA-related protein-targeted interventions modifying BCAA metabolism and insulin sensitivity
BCAA transporters
Considering lack of BCAA-specific transporters, literature data concern the modification of all neutral amino acid import. Recently, inhibitors of SLC6a19/B0AT1 were shown to block amino acid uptake in mouse intestine and kidneys (Q. Cheng et al. Citation2017; Yadav et al. Citation2020; Danthi et al. Citation2019). The metabolic outcomes of such intervention may resemble the effects observed in SLC6a19/B0AT1-depleted mice, such as greater GLP-1 and FGF21 release, reduced mTOR signaling in muscle, adipose tissue and liver, improved glycaemic control, reduced adiposity and hepatic glucose output, low levels of triacylglycerol and cholesterol, decreased steatosis in the liver, and resistance to diet-induced obesity (Yadav et al. Citation2020; Jiang et al. Citation2015). Mutations in human SLC6a19/B0AT1 result in Hartnup disorder, which is malabsorption of amino acids. Mostly asymptomatic course of the disease results possibly from high dietary protein intake in modern diets, that exceeds protein daily requirements (80-100 g), or a compensatory transport of amino acids by other carriers (Javed and Fairweather Citation2019). Chemical disruption of LAT1 has also been explored (Napolitano et al. Citation2017) and led to mTOR inhibition and Gcn2 activation (Scalise et al. Citation2018; Cormerais et al. Citation2016) as a promising anticancer effects (Cormerais et al. Citation2016). In rat insulinoma cells, knockdown of LAT1 inhibited mTOR signaling and leucine-mediated insulin over-secretion (Q. Cheng et al. Citation2016), being a novel therapeutic approach in type 2 diabetic patients.
Bcat modulation
The reliance on BCAA as metabolic substrates gradually enhances during cellular differentiation (Dhanani, Mann, and Adegoke Citation2019; Estrada-Alcalde et al. Citation2017; Kitsy et al. Citation2014; Sun et al. Citation2016) and, accordingly, depletion of BCAA-metabolizing enzymes may affect this process. For instance, BCATm deletion impaired myotube formation and induced apoptosis. However, these effects extended beyond the Bcat role in leucine metabolism since KIC, KIV or KMV addition did not return proper L6 cells differentiation (Dhanani, Mann, and Adegoke Citation2019). Concurrent knockdown of Bckdk was beneficial for the differentiation of cells suggesting that other downstream BCAA intermediates are of critical importance for appropriate cell growth (Dhanani, Mann, and Adegoke Citation2019). It can be also predicted that if perturbed BCAA oxidation in adipocytes affects lipid deposition in the tissue, then ectopic fatty acid accumulation occurs precluding insulin resistance development. The importance of Bcat activity was also supported by the transplantation of 750 mg of fat tissue to Bcat-/- mice. Two weeks after, the animals expressed lower postprandial circulating BCAA content combined with upregulated BCAA oxidation in adipocytes (i.e., elevated synthesis of alanine and glutamine from BCAA-derived nitrogen) (Herman et al. Citation2010). Most likely, the increased level of BCAA intermediates allosterically activated adipose Bckdh, thus compensating previously disturbed BCAA oxidation.
Metabolic phenotype of gastrocnemius muscle was evaluated in mice lacking BCATm by next-generation mRNA sequencing. Particularly, BCATm deletion led to muscular dystrophy along with the declined expression of multiple genes involved in anaerobic and oxidative disposal of carbohydrates, lipids and BCAA (Lynch et al. Citation2015). Both male and female mice bearing whole-body BCATm deletion were characterized by increased plasma BCAA, but not BCKA, levels, followed by higher food intake together with increased energy expenditure (i.e., higher oxygen consumption and respiratory quotient), augmented protein turnover, improved glucose and insulin tolerance, and ultimately ameliorated diet-induced obesity. BCATm-/- animals were protected against insulin signaling impairment independently of their locomotor activity, uncoupling proteins amount or autonomic nervous system activity (She, Reid, et al. Citation2007). Presumably, loss of BCATm affected the synthesis of gluconeogenic precursors for the liver. Moreover, slight increases in energy expenditure and carbohydrate usage (i.e., decreased RER value) at night, but not during the day, were observed in female mice devoid of BCATm (Neishabouri et al. 2015). Pharmacological inactivation of Bcat by LY3351337 administration for 10 days augmented circulating BCAA, but not BCKA, levels followed by reduced food intake and body mass of Zucker fatty rats, improved systemic glucose and insulin tolerance (White et al. Citation2020). It is also important that the effects of pharmacological Bcat modulation are less pronounced than after a global enzyme deletion and may be reminiscent of dietary BCAA supplementation.
Furthermore, BCATm-/- male mice had considerably higher rates of glucose contribution to ATP generation in the heart with no alterations in cardiac function and improved insulin response (Uddin, Pherwani, et al. Citation2019). Similarly, heart-restricted BCATm knockout did not impede cardiac function and improved insulin sensitivity of cardiomyocytes by accelerating insulin-dependent glucose oxidation, with no changes in glucose uptake or glycolytic rates, as well as by increasing Akt phosphorylation and Pdh expression (Karwi et al. Citation2020). It suggests that instead of BCAA accumulation, the raise in BCKA or their downstream intermediates content is more toxic and directly renders insulin resistance. In concordance with this theory, perfusion of the heart with high concentrations of BCKA abolished insulin-dependent glucose oxidation, abrogated translocation of Akt to the mitochondria and deactivated Pdh (Karwi et al. Citation2020). 3T3-L1 cells-based data underscored that BCKA can deteriorate insulin sensitivity by reduced basal Akt phosphorylation in a mTORC1-dependent mechanism (Zhou et al. Citation2019). Moreover, acute incubation with 0.05 mM sodium salts of KIC, KIV and KMV, or a combination of all three BCKA downregulated mitochondrial oxygen consumption, insulin signaling and glucose uptake in C2C12 cells, L6 myotubes and adult cardiomyocytes (Biswas, Dao, et al. Citation2020). The described effects can be restored by rapamycin supplementation, therefore highlighting the crucial role of mTOR signaling in the effects exerted by BCKA (Biswas, Dao, et al. Citation2020). Nevertheless, the induced metabolic abnormalities in the skeletal muscle can depend on BCKA transamination to BCAA since the abrogation of KIC-dependent mTORC1 activation and alleviation of insulin-mediated glucose uptake were demonstrated in Bcat2-deprived L6 myotubes (Moghei et al. Citation2016). Moreover, KIC-stimulated hypersecretion of insulin was suppressed by Bcat2 inhibition in a mice model of type 2 diabetes (Rabaglia et al. Citation2005).
As mentioned previously, hepatic BCATm activity is relatively low, therefore the liver cooperates with skeletal muscle to achieve an appropriate BCAA shuttle toward oxidation (Holeček Citation2020). Transgenic mice overexpressing BCATm specifically in the liver had lower hepatic, but not systemic, BCAA concentrations paralleled by the synthesis of nonessential amino acids. High fat diet challenge attenuated hepatic, but not muscular or cardiac, mTOR pathway affecting glycolysis rates as well as deteriorating glucose tolerance (Ananieva et al. Citation2017). Bearing in mind improved glucose tolerance after global BCATm deletion (She, Reid, et al. Citation2007), adaptation of liver BCAA catabolism to the alterations in whole-body metabolic background is crucial to preserve insulin sensitivity.
Long-term BCAA overabundance in mice with BCATm deficiency triggered also leptin-independent increase in hypothalamic orexigenic neuropeptide levels (i.e., Npy and Agrp). The authors speculated that the impaired BCAA metabolism within brain glia can be the cause for affected ability to sense amino acids. Consequently, BCATm-devoid animals had lower weight than control animals, despite similar rates of calorie intake, and exhibited preference toward low BCAA level in a diet (Purpera et al. Citation2012), indicating metabolic changes within the brain that limit requirements for BCAA transamination.
Bckdh modulation
Bckdha-E1α is indispensable for survival since mice harboring Bckdha-E1α knockout died within 24 h upon birth. Nonetheless, heterozygote animals with preserved half of Bckdha activity were viable, and after the administration of 60% protein diet displayed lower food intake, higher body mass and adiposity as compared to control mice (Taghavi and Hutson Citation2012). Clinically, residual Bckdh activity leads to maple sirup urine disease (MSUD), a hereditary disorder manifested with feeding problems, ketonuria, hypotonia and progressive neurological deterioration. Without early diagnosis and dietary BCAA restriction, the disease terminates in cerebral edema, central respiratory failure and death (Streck et al. Citation2021).
Cell culture experiments unveiled a strong association between Bckdh expression and energy substrate metabolism. Particularly, C2C12 myotubes and neonatal rat cardiomyocytes transfected with Bckdha adenovirus had a modestly diminished BCKA level with a concomitant increase in Pdh activity and insulin-dependent phosphorylation of Akt1 (Ser473) and Akt2 (Ser474), while largely reciprocal effects were caused by silencing Bckdh expression (Biswas, Dao, et al. Citation2020). Additionally, Bckdha depletion increased the phosphorylation of mTORC1 (Ser2448) and its targets, such as p70 S6K (Thr389) and eukaryotic translation initiation factor 4 G (eIF-4G) (Ser1108), therefore contributing to insulin resistance development (Biswas, Dao, et al. Citation2020). Knockdown of Bckdha in 3T3-L1 pre-adipocytes compromised lipid deposition (i.e., decreased abundance of C14:0, C:15:0, C16:0, C17:0, C18:0, C18:1 fatty acids and reduced PPARγ, Glut4, Plin4, Adipoq, Lep transcription) and adipogenic differentiation (i.e., impaired lipid droplet formation) (Green et al. Citation2016). Additionally, pancreatic tumor cells devoid of Bckdha had substantially impaired fatty acid synthesis and triacylglycerol accumulation, while mitochondrial function was not altered (i.e., sustained levels of TCA cycle intermediates and oxygen consumption) (Lee et al. Citation2019). The above data imply that Bckdh activity can be protective against lipotoxicity by the accumulation of excessive fatty acids in the form of triacylglycerol, and consequently this effect contributes to proper insulin signaling. Importantly, Bckdh activity was decreased in the liver and adipose tissue of Zucker fatty rats (White et al. Citation2016) and obese male mice (Zhang et al. Citation2016), while in the skeletal muscle either increased (White et al. Citation2016) or unchanged Bckdh phosphorylation (Zhang et al. Citation2016) was noticed (). Although models with a direct Bckdh overexpression/knockdown are rarely used, the regulation of Bckdh phosphorylation was a subject of several studies ().
Table 3. Metabolic characteristic of subjects undergone genetic or pharmacological modulation of enzymes responsible for Bckdh phosphorylation/dephosphorylation.
PP2Cm modulation
PP2Cm is a mitochondrial matrix molecule required for nutrient- and hormone-dependent control of Bckdh activity (Lu et al. Citation2009). For instance, PP2Cm-null mice were unable to enhance Bckdh activity after adiponectin administration (Lian et al. Citation2015). Mutations in human PP2Cm increased plasma BCAA and BCKA levels to those observed in intermittent (mild) form of MSUD (Oyarzabal et al. Citation2013). Genetic ablation of PP2Cm in murine models and defects in BCAA catabolism resulted in the moderate accumulation of BCAA and BCKA in the plasma, skeletal muscle, liver and white adipose tissue in lean mice (Wang et al. Citation2019), while in the setting of protein-enriched diet a metabolic phenotype reminiscent of human MSUD developed (Lu et al. Citation2009).
Abrogation of PP2Cm is combined with beneficial health changes in lean mice, including enhanced insulin sensitivity, glucose tolerance, reduced body weight and adiposity as well as the preference for carbohydrate over lipid utilization. Metabolomics analysis uncovered that most pronounced alterations in gluconeogenesis/glycolysis, glycogen metabolism, and TCA cycle due to PP2Cm deprivation appeared in the liver, instead of adipose tissue or skeletal muscle (Wang et al. Citation2019). In the heart, abnormal BCAA and BCKA concentrations in a mouse model of PP2Cm deficiency were connected with the reduced rate of glucose uptake and oxidation, glycogen accumulation and protein glycosylation (Sun et al. Citation2016; Li et al. Citation2017). Non-transcriptional mechanisms underlined these changes, including suppressed hexosamine biosynthesis and Pdh activity (Li et al. Citation2017). In turn, augmented fatty acid oxidation in the heart was evidenced by increased palmitate-induced mitochondrial oxygen consumption in cardiomyocytes isolated from PP2Cm knockout mice. It was associated with PPARα-mediated promotion of oxidative stress and lipid peroxidation (Sun et al. Citation2016; Li, Xiong, et al. Citation2020). As a consequence, PP2Cm deletion aggravated hypoxia/reoxygenation injury in cardiomyocytes, as well as the hearts from knockout mice were more susceptible to ischemia/reperfusion injury (Li, Xiong, et al. Citation2020; Li et al. Citation2017). Additionally, exacerbated ischemia/reperfusion damage to the heart was linked to caspase-3 activation, cellular apoptosis and lactate dehydrogenase (Ldh) release (Li, Xiong, et al. Citation2020). PP2Cm-/- mice eventually developed cardiac systolic dysfunction that aggravated with age and resulted in pressure overload-induced cardiomyopathy (Sun et al. Citation2016). Interestingly, PPARα knockdown (Li, Xiong, et al. Citation2020) or myocardial Glut1 overexpression in PP2Cm knockout hearts normalized the rate of glucose oxidation (Li et al. Citation2017) protecting the heart from ischemia/reperfusion-mediated dysfunction (Li, Xiong, et al. Citation2020; Li et al. Citation2017).
BCAA-derived carbons contribute to ∼20% of TCA cycle in the pancreatic islets (Neinast, Jang, et al. Citation2019) and are linked with their endocrine function. BCAA deprivation was shown to be the cause for reduced insulin secretion independently of plasma glucose clearance in rats (Horiuchi et al. Citation2017). It may be indicative of improved insulin response in peripheral tissues. Such outcomes were later supported by lowered insulin secretion from human pancreatic islets in response to PPM1K downregulation (Taneera et al. Citation2012). Generally, leucine exerts insulinotropic effect through allosteric activation of glutamate dehydrogenase (Gdh) and an increase in ATP pool (Neinast, Murashige, et al. Citation2019). Both the appropriate BCAA concentration and metabolic flux are involved in the control of insulin outflow, however excessive BCAA accumulation within β cells may be deleterious. Particularly, a 7.4-year follow-up study on METabolic Syndrome In Men (METSIM) cohort showed that leucine, isoleucine and valine contents were correlated with diminished insulin concentrations, elevated fasting and 2 h glucose levels in OGTT (Vangipurapu et al. Citation2019). Interventions based on restricted BCAA supply (-60%) to diabetic patients coincided with a reduced postprandial insulin release, but improved insulin sensitivity (Karusheva et al. Citation2019). In murine pancreatic islets, reducing BCAA supply also augmented glucagon content in conjunction with a diminished fasting insulin level and pancreas mass (Solon-Biet et al. Citation2019). These data suggest that reduced BCAA delivery to β cells in terms of obesity can optimize the organ function and improve postprandial glucose tolerance.
PP2Cm expression is downregulated in the liver and adipose tissue, but not skeletal muscle, of both ob/ob and type 2 diabetic mice as compared to the wild type. At the same time, a significantly reduced Bckdh activity as well as enhanced plasma BCAA and BCKA amount were observed (Lian et al. Citation2015). Moreover, genomic studies identified allelic variants of PPM1K associated with a higher weight gain and worsened insulin sensitivity in individuals on a high-fat diet (Xu, Qi, et al. Citation2013). Generally, the actions attempting to stimulate PP2Cm alleviated disturbances in BCAA catabolism during diabetes (Lian et al. Citation2015), while abrogation of PP2Cm induced changes associated with obesity or insulin resistance, such as oxidative stress, apoptosis, JNK and p38 activation in animals and cells (Oyarzabal et al. Citation2013; Lu et al. Citation2009). The contrast between lean and obese mice in the effects observed due to PP2Cm deprivation (Wang et al. Citation2019) is not yet resolved. Nonetheless, high fat diet challenge combined with PP2Cm knockout in mice did not affect beneficial impact of vertical sleeve gastrectomy, such as weight loss and glycaemic improvements (Bozadjieva Kramer et al. Citation2020), indicating a major role of other Bckdh regulators.
Bckdk modulation
Nutritional and hormonal signals influence Bckdk activity, thereby adapting BCAA catabolism to the current cellular metabolic status. Before entering the mitochondria, a 30-amino acid sequence is cleaved from cytosolic pool of Bckdk and then the enzyme suppresses Bckdh activity (White et al. Citation2018) (). Bckdk expression is significantly increased in murine diabetic tissues, including the liver and adipose tissue (Lian et al. Citation2015), what may contribute to insulin signaling impairment. In fact, C2C12 myotubes transfected with Bckdk adenovirus exhibited a substantial increase in BCKA content consistent with a decreased insulin-dependent phosphorylation of Akt1 (Ser473) and Akt2 (Ser474) (Biswas, Dao, et al. Citation2020). On the other hand, KIC-dependent Bckdk activity depression in C2C12 myotubes contributed to increases in complete fatty acid oxidation and cellular respiration (Lerin et al. Citation2016).
Restoration of Bckdh catabolic activity by Bckdk knockout in healthy mice led to an enhanced systemic BCAA oxidation, primarily in the skeletal muscle. However, the limited amount of nitrogen acceptors for transamination, such as α-ketoglutarate and pyruvate, may slow down the process and create a new metabolic equilibrium. Hence, no consequent differences in glucose clearance or circulating free fatty acids between knockout and control animals were demonstrated (Neinast, Jang, et al. Citation2019). Importantly, inadequate manipulation of BCAA metabolic fate can trigger deleterious outcomes. Systemic loss of Bckdk in mice lowered brain, muscle, adipose tissue and the whole-body mass, but most importantly caused neurological defects (Ishikawa et al. Citation2017). Bckdk deficit and abnormal BCAA levels within the brain were associated with neurobehavioral deficits such as autism, epilepsy, and intellectual impairment (Novarino et al. Citation2012). On the other hand, the heart and skeletal muscle-specific Bckdk-depleted mice exhibited normal food intake, growth rate and tissue weights, thereby implying a crucial role of sustained BCAA supply to organs other than the striated muscles for preserving body and tissue mass (Ishikawa et al. Citation2017). Bckdk loss in striated muscles resulted also in the impaired synthesis of myofibrillar proteins under reduced protein feeding through a diminished mTORC1 activity without an impact on autophagy (Ishikawa et al. Citation2017).
Recently, an inhibitor of Bckdk activity was revealed, namely 3,6-dichlorobenzo[b]thiophene-2-carboxylic acid (BT2) (Tso et al. Citation2014). A single intravenous BT2 injection (40 mg/kg) to mice augmented isoleucine oxidation specifically within the skeletal muscle, despite BT2 presence in all tested tissues (i.e., pancreas, heart, quadriceps and soleus muscle, liver, brown and white adipose tissue) and the significant reduction in Bckdha-E1α phosphorylation (Ser293) in the pancreas, liver and heart (Neinast, Jang, et al. Citation2019). It is no surprising, since the skeletal muscle is the main tissue engaged in BCAA catabolism. Moreover, it evidences that BCAA oxidation depends not only on Bckdh phosphorylation, but also on the modulation of Bckdh activity by variable substrates (e.g., NADH, acyl-CoA) or the rate of other metabolites incorporation into TCA cycle (e.g., products of glycolysis, fatty acid β-oxidation) (Neinast, Jang, et al. Citation2019). Prolonged BT2 administration (7 days, intraperitoneal injection in a volume of 0.2 ml BT2 at 20 mg/kg/day) increased Bckdh activity in the heart, skeletal muscle, kidneys and the liver of mice (Tso et al. Citation2014). Bckdk inactivation with BT2 effectively inhibited tissue Bckdh phosphorylation (Ser293), retrieved the expression of several downstream genes in BCAA catabolism and lowered circulating BCAA and BCKA levels also in obese Zucker rats (20 mg/kg i.p. for one week) (White et al. Citation2018), mice receiving high fat diet (40 mg/kg/day for 8-10 weeks) (Zhou et al. Citation2019) as well as PP2Cm-/- rodents (oral gavage at 40 mg/kg/day) (Sun et al. Citation2016). Nonetheless, it should be noticed that BT2 administration has an inhibitory effect on cellular proliferation and differentiation as observed in C2C12 myotubes with doses ranging from 10 to 100 µM (Sato et al. Citation2020), being in agreement with the gradually elevating necessity for BCAA during cell growth.
What is more, healthy mice treated with BT2 showed improved lipid profile, including declined levels of circulating free fatty acids (Neinast, Jang, et al. Citation2019) and elevated respiration capacity in isolated cardiac mitochondria (i.e., increased JNADH specific for Bckdh) (Goldberg et al. Citation2019). Furthermore, pharmacological modulation of Bckdh activity through BT2 treatment potentiated the rate of glucose oxidation (i.e., increased Glut1 mRNA level and Pdh activity) and exerted an insulin sensitizing effect in C2C12 myotubes and neonatal rat cardiomyocytes (Biswas, Dao, et al. Citation2020). High fat diet combined with BT2 treatment did not modify food intake or body weight in ob/ob mice (Zhou et al. Citation2019) and Zucker fatty rats (White et al. Citation2018), although attenuated glucose intolerance, alleviated insulin resistance and diminished insulin release. These observations may be ascribed to a BT2-mediated suppression in mTORC1 activity (i.e., decreased p70 S6K (Thr389)/p70 S6K ratio) in the skeletal muscle, adipose tissue and liver (Zhou et al. Citation2019). Additionally, BT2 administration blocked hepatic steatosis by reducing the production of substrates for de novo lipogenesis and declined triacylglycerol accumulation in the Zucker fatty rats liver through the down-regulation of Acl phosphorylation (Ser454) and induced fatty acid oxidation (White et al. Citation2018). These data clearly state that BCAA catabolic defects may underlie the pathology of insulin resistance and BT2 potentially alleviates this disorder.
Positive consequences of BT2 administration were also proved by improvements in the contractile function of failing heart. This disorder is associated with a coordinated decline in cardiac BCAA-related enzymes and amino acid overabundance paralleled by mTOR activation, lower KLF15 expression and impaired insulin signaling (Uddin, Zhang, et al. Citation2019). Moreover, myocardial ischemia/reperfusion injury caused by either excessive BCAA supplementation or PP2Cm knockout in mice can be restrained by increased BCAA catabolism through BT2 treatment. These improvements included decreased infarct size and restored parameters of cardiac function, including retained ejection fraction in the left ventricle and diminished chamber dilation (Sun et al. Citation2016; Li et al. Citation2017). However, BT2 did not enhance myocardial function in the control animals, suggesting that high level of BCAA, instead of a defect in their catabolism, was a primary cause for the adverse heart function (Li et al. Citation2017).
The studies conducted on C2C12 myotubes with the use of phenylbutyrate, another Bckdk inhibitor, supported a link between BCAA metabolism and augmented glucose uptake and glycogen synthesis (Hu et al. Citation2014), while under elevated BCAA provision increased Akt and AS160 phosphorylations and reduced glycogen content were detected (Crossland et al. Citation2020). However, the above data may be linked to the nonspecific phenylbutyrate actions, since it can also control other biological pathways, including modulation of Pdh complex (Ferriero and Brunetti-Pierri Citation2013), or inhibition of histone deacetylases (Khan, Komarya, and Jena Citation2017). Nonetheless, phenylbutyrate role in insulin sensitivity is poorly explored, since studies concentrate rather on its therapeutic potential toward urea cycle disorders (Iannitti and Palmieri Citation2011; Oishi and Diaz Citation2014).
Hibch modulation
Among other BCAA-related enzymes novel research focus on the action of Hibch that converts valine to 3-HIB, which is the only BCAA metabolite not trapped within the cells by covalent linkage to CoA () (Kamei et al. Citation2020). The secretion of 3-HIB is similar between white and brown adipocytes, and intensifies with the increase of BCAA catabolism during the differentiation of 3T3-L1 cells and primary human adipocytes (Nilsen et al. Citation2020). Importantly, 3-HIB may act in autocrine/paracrine manner to control glucose and lipid homeostasis. In cultured primary human umbilical vein endothelial cells (HUVEC), the addition of 3-HIB substantially augmented fatty acid uptake, while Hibch deletion prevented the increase in fatty acid uptake in PGC-1α overexpressing C2C12 myotubes (Jang et al. Citation2016). Consequently, augmented muscular secretion of 3-HIB promoted fatty acid transport through endothelium to the skeletal muscle, ultimately enforcing lipotoxicity (i.e., muscular triacylglycerol and diacylglycerols accumulation), protein kinase C-θ membrane translocation, reducing Akt1 phosphorylation (Ser473) and evoking glucose intolerance (Jang et al. Citation2016). The effect on fatty acid transport was not uniform among the used models. Although substantially increased fatty acid uptake was demonstrated in differentiated white and brown adipocytes already after ∼5 min of 3-HIB administration (Nilsen et al. Citation2020), in HUVEC and human cardiac microvascular endothelial cells, these observations were not recapitulated in human adipose tissue-derived endothelial cells (Mardinoglu et al. Citation2018).
Another studies aimed to establish a role of 3-HIB in glucose metabolism, and, based on two weeks of 3-HIB administration to wild-type mice, no effect on hepatic carbohydrate turnover was established (i.e., gluconeogenesis gene expression or glucose output from the liver) (Jang et al. Citation2016). However, incubation with supraphysiological amounts of 3-HIB (24 h) induced insulin-stimulated glucose uptake in white and brown adipocytes. Moreover, in white adipose tissue declined maximal mitochondrial respiration, spared respiratory capacity as well as ROS synthesis were noticed, in opposite to the effects in brown adipocytes (Nilsen et al. Citation2020). Therefore, it seems that either fatty acid biosynthesis or degradation may be mediated by 3-HIB in various cells. When Hibch was knocked down in the mature white and brown adipocytes, decreased 3-HIB release from the cells coincided with a diminished lipid accumulation by approximately 25% (Nilsen et al. Citation2020). Of note, in colorectal cancer cells, abnormally high Hibch level upregulated the metabolism of TCA cycle as well as oxidative phosphorylation (Shan et al. Citation2019). Collectively, alterations in 3-HIB may acutely correspond to systemic metabolic disturbances, and contribute to metabolic cross-talk in various tissues.
Interestingly, 3-HIB secretion was diminished during glucose and insulin infusion in the hyperinsulinemic-euglycemic clamp procedure in women, most likely reflecting antiproteolytic properties of insulin, while protein ingestion reversed this effect (Harris et al. Citation2017). On the other side, similarly to BCAA and BCKA, 3-HIB concentrations were significantly elevated in the skeletal muscle from db/db mice and from human patients with type 2 diabetes (Jang et al. Citation2016) as well as in plasma of obese subjects in a population of around 10,000 humans (Mardinoglu et al. Citation2018). Moreover, 3-HIB can prognosticate future risk of type 2 diabetes, although this association was strongly impacted by BMI (Mardinoglu et al. Citation2018). In a cohort of 4942 men and women, circulating 3-HIB amount mirrored the degree of hyperglycemia being most elevated in the individuals with type 2 diabetes (Nilsen et al. Citation2020) and exhibited stronger association with diabetes than other insulin resistance indicators (i.e., BMI, triacylglycerol, HDL-C, and valine) (Nilsen et al. Citation2020). Because circulating 3-HIB concentrations are ∼10-fold lower than valine (Nilsen et al. Citation2020), changes in its level can be more readily evident than other BCAA metabolites and serve as markers of metabolic disturbances.
Methylmalonyl-CoA mutase (mut) modulation
Mut catalyzes a distal step in oxidative metabolism of valine and isoleucine, but also threonine, methionine, odd chain fatty acids and cholesterol, as it drives methylmalonyl-CoA conversion into succinyl-CoA (Ghoraba, Mohammed, and Zaki Citation2015). Homozygotic loss of Mut and methylmalonic acid accumulation impeded mitochondrial function and was lethal in early neonatal state (Chandler et al. Citation2009). In humans, methylmalonic acidemia (MMA) is a rare inherited disorder characterized by severe acidosis, respiratory distress, renal and neurological impairment, and ketosis (Costanzo et al. Citation2020). Importantly, muscular Mut level, among the tested BCAA catabolic genes, was most strongly correlated with insulin resistance in humans in line with its reduced expression in diabetic individuals (Lerin et al. Citation2016). In experimental conditions, heterozygous Mut+/− mice exhibited normal growth, although several metabolic defects were revealed, including elevated weight gain due to high fat diet, fasting hyperinsulinemia and declined glucose tolerance. In the skeletal muscle, BCAA level did not change, while augmented acylcarnitines and triacylglycerol contents were noticed, thereby reflecting impaired fatty acid oxidation (Lerin et al. Citation2016).
Physical activity and BCAA metabolism
Exercise are key components of healthy lifestyle and are considered as a therapy for the prevention and treatment of insulin resistance and its co-morbidities. Training-mediated effects include an increase in BCAA oxidation (Kujala et al. Citation2016) through a decline in Bckdk activity (Xu et al. Citation2001) and elevated Bckdh activity, as was evidenced in human and rat skeletal muscle (Wagenmakers Citation1998), as well as the remodeling of gut microbiota (i.e., diminished replication rate of BCAA-synthesizing bacteria Prevotella copri and an increase in microbial genes responsible for BCAA oxidation) (Liu et al. Citation2020). Exercise, and associated PGC-1α activation, augment valine metabolism to generate β-aminoisobutyric acid (BAIBA) (Stautemas et al. Citation2019; Roberts et al. Citation2014), while physical inactivity in patients undergoing hemodialysis reduces its plasma concentration (Molfino et al. Citation2019). BAIBA is considered a myokine that contributes to exercise-dependent improvements in metabolic profile. Indeed, BAIBA supplementation upregulated oxidative potential of the liver, induced browning of white adipose tissue and improved insulin sensitivity in mice fed standard diet (Roberts et al. Citation2014). Moreover, BAIBA inhibited inflammation, increased glucose uptake and the expression of free fatty acid oxidation enzymes as well as attenuated insulin resistance in palmitate-treated C2C12 myotubes and lipopolysaccharide-exposed 3T3-L1 adipocytes (Jung et al. Citation2015; Jung et al. Citation2018). In type 2 diabetic mice, oral administration of BAIBA reduced blood glucose level, decreased circulating triacylglycerol, cholesterol and free fatty acids amount, downregulated the expression of enzymes involved in gluconeogenesis and lipogenesis in the liver, declined hepatic lipid accumulation as well as restored insulin signaling (Shi et al. Citation2016). These data provide novel insights linking exercise, BCAA metabolism and glucose/lipid interplay, indicating therapeutic options for diabetic patients. Nonetheless, 12 weeks training-mediated reduction in intrahepatic lipids and alleviated insulin resistance in patients with NAFLD did not coincide with diminished plasma BCAA levels (Vanweert et al. 2021). These observations imply differential regulatory mechanisms related with BCAA catabolism and lipid degradation in the liver upon physical activity.
The efficient catabolism of fatty acids and BCAA is one of the conditions for high intrinsic exercise capacity in rats as compared to the low training capacity animals. Such metabolic adaptations enable to maintain glycogen reservoirs and lower the accumulation of short- and medium-chain acylcarnitines (Overmyer et al. Citation2015). Chronic BCAA or their metabolite (i.e., HMB) supplementation improved exercise performance presumably through increased protein synthesis, induced mitochondrial biogenesis, replenished TCA cycle anaplerotic reactions and blunted immunological response (Kephart et al. Citation2015; Townsend et al. Citation2013). Accordingly, supplements for athletes are often enriched in BCAA and, combined with exercise-dependent promotion of the complete oxidation of amino acids, can reduce the amount of produced deleterious intermediates, such as acylcarnitines (Shou, Chen, and Xiao Citation2019). Uncontrolled BCAA metabolism in mice with muscle-targeted Bckdk knockout evoked lower adaptability to exercise training in these animals as a result of acylcarnitine derivatives production, reduced mitochondrial enzyme activities (i.e., citrate synthase, cytochrome c oxidase) and diminished muscle glycogen stores (Xu et al. Citation2017).
Exercise are associated with elevated provision of substrates to build muscle mass (Xu et al. Citation2001) and BCAA may exert an additive effect on protein synthesis and muscle functional capacity through the activation of mTORC1 signaling cascade (Jackman et al. Citation2017). Consistently, leucine-enriched diet consumption retarded age-related decline in muscle mass as amino acid, alone or combined with resistive exercise, improved muscle anabolism (Devries et al. Citation2018). Other data also point to a positive impact of BCAA supplementation on muscle regeneration after exercise-induced damage, especially when a high daily BCAA intake (>200 mg/kg/day) lasted for over 10 days prior training (Fouré and Bendahan Citation2017). BCAA are suggested to extend time to exhaustion during physical activity also by neuronal mechanisms. Hyperstimulation of BCAA catabolism due to Bckdk deficiency in cerebral cortex neurons (BckdkEmx1 knockout mice) was related with the increased endurance capacity following training likely because of downregulated central and peripheral fatigue caused by running (Mizusawa et al. Citation2020). In fact, the consumption of BCAA-rich beverage 1 hour prior physical training constrained neuronal transport of tryptophan and further reduced serotonin synthesis, thus preventing central fatigue (Abumoh’D, Matalqah, and Al-Abdulla Citation2020). However, a declined serotonin level can be counterbalanced by the diminished uptake of tyrosine and reduced catecholamine production, the mediators of enhanced physical performance. So that, eventually no improvement in training capacity was observed in humans supplemented with BCAA (Choi et al. Citation2013). Furthermore, exercise induce ammonemia, in an intensity-dependent manner, by both BCAA and AMP deamination, which promotes fatigue by affected NAD+/NADH ratio and cellular permeability to ions (Coqueiro, Rogero, and Tirapegui Citation2019). Additionally, the resultant exacerbated intracerebral influx of large neutral amino acids and aromatic amino acids, through their exchange with glutamine, alters intracerebral synthesis of catecholamines (serotonin and dopamine) and enhances ‘false neurotransmitters’ generation (octopamine and phenylethylamine) (Bassini et al. Citation2013). Therefore, hyperammonemia is another reason for BCAA supplementation in training, further supported by the endurance impairment in mice with BCATm deletion (i.e., in part by reduced mitochondrial glutamate quantity and high ammonia accumulation) (She et al. Citation2010). The BCAA byproduct, glutamine, enhances muscle glycogen stores, declines muscle damage, reduces muscle AMP deamination, heightenes urea disposal and protects against ammonia rise during exercise (Bassini-Cameron et al. Citation2008; Coqueiro, Rogero, and Tirapegui Citation2019). However, the BCAA-mediated beneficial effects are dose-dependent and higher BCAA ingestion augmented hyperammonemia and reduced time to exhaustion in trained rats (Falavigna et al. Citation2012). In another studies BCAA also fail to improve exercise performance, muscle soreness or mass loss, thereby the BCAA supplementation in athletes needs further evaluation (Master and Macedo Citation2021). In this regard, the altered amino acid balance in the skeletal muscle should be considered as a consequence of long-term alone dietary BCAA provision. Muscle protein synthesis is then restricted by insufficiency in other essential amino acids, what leads to protein breakdown as their source. The released essential amino acids that will not be reincorporated into protein synthesis, can be oxidized or released to the blood, thereby during prolonged BCAA supplementation a decrease in protein synthesis can be observed (Wolfe Citation2017). It is worth emphasizing that physical activity cannot be replaced by exogenously administered BCAA, as it was recently confirmed that exercise more effectively reduced adiposity, serum insulin concentrations and TNF-α production than leucine administration in rats (Torres-Leal et al. Citation2011).
Conclusions and future perspectives
BCAA are fundamental constituents of the diet and condition the maintenance of proper organ and tissue function (). Excessive accumulation of BCAA and BCKA is a widely-proved metabolic feature associated with obesity and an early risk factor for insulin resistance, NAFLD or type 2 diabetes development. Several processes contribute to this phenotype, including higher energy substrate intake that interfere with BCAA degradation, disturbed relationship between BCAA-catabolizing tissues (i.e., mitochondrial dysfunction and insufficient flux through the TCA cycle prevents interorgan compensation for amino acid turnover), impaired insulin-mediated suppression of protein breakdown and/or attenuated amino acid-mediated protein synthesis. Simultaneous alterations in glycolysis and fatty acid oxidation in the insulin resistant state limit substrate availability for Bcat and generate Bckdh inhibitors. Likewise, hormonal dysregulation (i.e., attenuated insulin and adiponectin levels) reduces Bckdh activity. In turn, BCAA accumulation impairs insulin signaling through mTORC1 activation (), which elicits hyperinsulinemia that, along with the accumulation of toxic amino acid metabolites, leads to pancreatic β cell dysfunction. Moreover, BCAA and their metabolites (e.g., acylcarnitines, 3-HIB) alter mitochondrial function and transendothelial fatty acid transport, what may promote ectopic lipid storage together with inflammation and oxidative stress induction that affect both insulin signal transduction and BCAA metabolism. Moreover, high rates of BCAA oxidation impair lipid export, in the form of acyl-glycine complexes, from the skeletal muscle, thus resulting in acyl-CoA accumulation. Another negative outcome is the competition between BCAA and other essential amino acids to cross blood-brain barrier that alters neurotransmitters synthesis (i.e., serotonin, dopamine) and increases the risk of depression during obesity (Coppola et al. Citation2013). However, largely inconsistent results were obtained in the used models of BCAA deprivation or over-administration. Several studies revealed alternative phenotype wherein elevated BCAA delivery was linked with improved glucose homeostasis due to elevated mitochondrial biogenesis and energy expenditure, reduced gluconeogenesis and/or improved hormone release (e.g., insulin, adiponectin, GLP-1). Based on the literature screening, excessive BCAA administration itself does not fulfill the criteria for metabolic disease development, since in healthy individuals this can be compensated by increased oxidative metabolism and higher energy expenditure. In such circumstances, BCAA may enhance, e.g., glucose tolerance and stimulate skeletal muscle mass gain. When the energy balance is no longer maintained (e.g., obesity), cellular mitochondria cannot cope with upregulated substrate availability which leads to enhanced BCAA, glucose or fatty acids secretion to the circulation and their deposition in tissues, that is reliable predictive factor for insulin signal impairment. Therefore, genetic and/or metabolic background of an individual may discriminate between the phenotype developed in response to BCAA level modulation. Current research also do not sufficiently mimic human dietary behaviors, daily physical activity and environmental factors, while safety and efficacy of BCAA-related interventions are only beginning to be unraveled in human population. Therefore, future randomized clinical trials should clarify which amino acid or amino acids mixture are most salutary for human health, and what are the physiological BCAA concentrations that do not induce harmful effects.
Disclosure statement
No potential competing interest was reported by the authors.
Funding
This work was supported by the National Science Center (Poland) under Grant 2020/04/X/NZ3/00406; and Medical University of Bialystok under Grant SUB/1/DN/21/010/1118.
References
- Adams, S. H. 2011. Emerging perspectives on essential amino acid metabolism in obesity and the insulin-resistant state. Advances in Nutrition 2 (6):445–56. doi: 10.3945/an.111.000737.
- Allam-Ndoul, B., F. Guénard, V. Garneau, O. Barbier, L. Pérusse, and M.-C. Vohl. 2015. Associations between branched chain amino acid levels, obesity and cardiometabolic complications. Integrative Obesity and Diabetes 1 (6):157–62. doi: 10.15761/IOD.1000134.
- Allman, B. R., E. C. Diaz, A. Andres, and E. Børsheim. 2020. Divergent changes in serum branched-chain amino acid concentrations and estimates of insulin resistance throughout gestation in healthy women. The Journal of Nutrition 150 (7):1757–64. doi: 10.1093/jn/nxaa096.
- Allman, B. R., B. J. Spray, K. E. Mercer, A. Andres, and E. Børsheim. 2021. Markers of branched-chain amino acid catabolism are not affected by exercise training in pregnant women with obesity. Journal of Applied Physiology 130 (3):651–9. doi: 10.1152/japplphysiol.00673.2020.
- Ananieva, E. A., C. G. Van Horn, M. R. Jones, and S. M. Hutson. 2017. Liver BCATm transgenic mouse model reveals the important role of the liver in maintaining BCAA homeostasis. The Journal of Nutritional Biochemistry 40 (February):132–40. doi: 10.1016/j.jnutbio.2016.10.014.
- Andersson-Hall, U., C. Gustavsson, A. Pedersen, D. Malmodin, L. Joelsson, and A. Holmäng. 2018. Higher concentrations of BCAAs and 3-HIB are associated with insulin resistance in the transition from gestational diabetes to type 2 diabetes. Journal of Diabetes Research 2018:4207067. doi:10.1155/2018/4207067
- Arakawa, M., T. Masaki, J. Nishimura, M. Seike, and H. Yoshimatsu. 2011. The effects of branched-chain amino acid granules on the accumulation of tissue triglycerides and uncoupling proteins in diet-induced obese mice. Endocrine Journal 58 (3):161–70. doi: 10.1507/endocrj.K10E-221.
- Arany, Z., and M. Neinast. 2018. Branched chain amino acids in metabolic disease. Current Diabetes Reports 18 (10):76. doi: 10.1007/s11892-018-1048-7.
- Arrieta-Cruz, I., Y. Su, and R. Gutiérrez-Juárez. 2016. Suppression of endogenous glucose production by isoleucine and valine and impact of diet composition. Nutrients 8 (2):79. doi: 10.3390/nu8020079.
- Aumoh’D, M. F., L. Matalqah, and Z. Al-Abdulla. 2020. Effects of oral branched-chain amino acids (BCAAs) intake on muscular and central fatigue during an incremental exercise. Journal of Human Kinetics 72 (1):69–78. doi: 10.2478/hukin-2019-0099.
- Averous, J., S. Lambert-Langlais, F. Mesclon, V. Carraro, L. Parry, C. Jousse, A. Bruhat, A.-C. Maurin, P. Pierre, C. G. Proud, et al. 2016. GCN2 contributes to MTORC1 inhibition by leucine deprivation through an ATF4 independent mechanism. Scientific Reports 6 (1):27698. doi: 10.1038/srep27698.
- Bai, J., E. Greene, W. Li, M. T. Kidd, and S. Dridi. 2015. Branched-chain amino acids modulate the expression of hepatic fatty acid metabolism-related genes in female broiler chickens. Molecular Nutrition & Food Research 59 (6):1171–81. doi: 10.1002/mnfr.201400918.
- Bar-Peled, L., and D. M. Sabatini. 2014. Regulation of MTORC1 by amino acids. Trends in Cell Biology 24 (7):400–6. doi: 10.1016/j.tcb.2014.03.003.
- Bassini, A., A. M. Magalhães-Neto, E. Sweet, A. Bottino, C. Veiga, M. B. Tozzi, M. B. Pickard, and L. C. Cameron. 2013. Caffeine decreases systemic urea in elite soccer players during intermittent exercise. Medicine & Science in Sports & Exercise 45 (4):683–90. doi: 10.1249/MSS.0b013e3182797637.
- Bassini-Cameron, A., A. Monteiro, A. Gomes, J. P. S. Werneck-de-Castro, and L. Cameron. 2008. Glutamine protects against increases in blood ammonia in football players in an exercise intensity-dependent way. British Journal of Sports Medicine 42 (4):260–6. doi: 10.1136/bjsm.2007.040378.
- Binder, E., F. J. Bermúdez-Silva, M. Elie, T. Leste-Lasserre, I. Belluomo, S. Clark, A. Duchampt, G. Mithieux, and D. Cota. 2014. Leucine supplementation modulates fuel substrates utilization and glucose metabolism in previously obese mice. Obesity 22 (3):713–20. doi: 10.1002/oby.20578.
- Biswas, D., K. T. Dao, A. Mercer, A. M. Cowie, L. Duffley, Y. El Hiani, P. C. Kienesberger, and T. Pulinilkunnil. 2020. Branched-chain ketoacid overload inhibits insulin action in the muscle. The Journal of Biological Chemistry 295 (46):15597–621. doi: 10.1074/jbc.RA120.013121.
- Biswas, D., L. Duffley, and T. Pulinilkunnil. 2019. Role of branched-chain amino acid-catabolizing enzymes in intertissue signaling, metabolic remodeling, and energy homeostasis. FASEB Journal 33 (8):8711–31. doi: 10.1096/fj.201802842RR.
- Biswas, D., K. Tozer, K. T. Dao, L. J. Perez, A. Mercer, A. Brown, I. Hossain, A. M. Yip, C. Aguiar, H. Motawea, et al. 2020. Adverse outcomes in obese cardiac surgery patients correlates with altered branched-chain amino acid catabolism in adipose tissue and heart. Frontiers in Endocrinology 11:534. doi: 10.3389/fendo.2020.00534.
- Blanchard, P.-G., R. J. Moreira, É. Castro, A. Caron, M. Côté, M. L. Andrade, T. E. Oliveira, M. Ortiz-Silva, A. S. Peixoto, F. A. Dias, et al. 2018. PPARγ is a major regulator of branched-chain amino acid blood levels and catabolism in white and brown adipose tissues. Metabolism 89:27–38. doi: 10.1016/j.metabol.2018.09.007.
- Blouet, C., Y. Hwan Jo, X. Li, and G. J. Schwartz. 2009. Mediobasal hypothalamic leucine sensing regulates food intake through activation of a hypothalamus-brainstem circuit. The Journal of Neuroscience 29 (26):8302–11. doi: 10.1523/JNEUROSCI.1668-09.2009.
- Boden, G., C. Homko, C. A. Barrero, T. P. Stein, X. Chen, P. Cheung, C. Fecchio, S. Koller, and S. Merali. 2015. Excessive caloric intake acutely causes oxidative stress, GLUT4 carbonylation, and insulin resistance in healthy men. Science Translational Medicine 7 (304):304re7. doi: 10.1126/scitranslmed.aac4765.
- Bolster, D. R., M. Rahn, A. G. Kamil, L. T. Bristol, S. R. Goltz, H. J. Leidy, M. Blaze Mt, M. A. Nunez, E. Guo, J. Wang, et al. 2018. Consuming lower-protein nutrition bars with added leucine elicits postprandial changes in appetite sensations in healthy women. The Journal of Nutrition 148 (5):693–701. doi: 10.1093/jn/nxy023.
- Borghi, L., R. Lugari, A. Montanari, P. Dall’Argine, G. F. Elia, V. Nicolotti, I. Simoni, A. Parmeggiani, A. Novarini, and A. Gnudi. 1985. Plasma and skeletal muscle free amino acids in type I, insulin-treated diabetic subjects. Diabetes 34 (8):812–815. doi: 10.2337/diab.34.8.812.
- Boulangé, C. L., S. P. Claus, C. J. Chou, S. Collino, I. Montoliu, S. Kochhar, E. Holmes, S. Rezzi, J. K. Nicholson, M. E. Dumas, et al. 2013. Early metabolic adaptation in C57BL/6 mice resistant to high fat diet induced weight gain involves an activation of mitochondrial oxidative pathways. Journal of Proteome Research 12 (4):1956–1968. doi: 10.1021/pr400051s.
- Boulet, M. M., G. Chevrier, T. Grenier-Larouche, M. Pelletier, M. Nadeau, J. Scarpa, C. Prehn, A. Marette, J. Adamski, and A. Tchernof. 2015. Alterations of plasma metabolite profiles related to adipose tissue distribution and cardiometabolic risk. American Journal of Physiology-Endocrinology and Metabolism 309 (8):E736–E746. doi: 10.1152/ajpendo.00231.2015.
- Bozadjieva Kramer, N., S. S. Evers, J. H. Shin, S. Silverwood, Y. Wang, C. F. Burant, D. A. Sandoval, and R. J. Seeley. 2020. The role of elevated branched-chain amino acids in the effects of vertical sleeve gastrectomy to reduce weight and improve glucose regulation. Cell Reports 33 (2):108239. doi: 10.1016/j.celrep.2020.108239.
- Brietzke, S. A. 2015. Oral antihyperglycemic treatment options for type 2 diabetes mellitus. The Medical Clinics of North America 99 (1):87–106. doi: 10.1016/j.mcna.2014.08.012.
- Bruckbauer, A., and M. B. Zemel. 2013. Synergistic effects of metformin, resveratrol, and hydroxymethylbutyrate on insulin sensitivity. Diabetes, Metabolic Syndrome and Obesity 6:93–102. doi: 10.2147/DMSO.S40840.
- Bruckbauer, A., and M. B. Zemel. 2014. Synergistic effects of polyphenols and methylxanthines with leucine on AMPK/sirtuin-mediated metabolism in muscle cells and adipocytes. PLoS One 9 (2):e89166. doi: 10.1371/journal.pone.0089166.
- Cancello, R., J. Tordjman, C. Poitou, G. Guilhem, J. L. Bouillot, D. Hugol, C. Coussieu, A. Basdevant, A. B. Hen, P. Bedossa, et al. 2006. Increased infiltration of macrophages in omental adipose tissue is associated with marked hepatic lesions in morbid human obesity. Diabetes 55 (6):1554–1561. doi: 10.2337/db06-0133.
- Chalvon-Demersay, T., J. Moro, P. C. Even, C. Chaumontet, D. Tomé, J. Averous, J. Piedcoq, C. Gaudichon, A.-C. Maurin, P. Fafournoux, et al. 2019. Liver GCN2 controls hepatic FGF21 secretion and modulates whole body postprandial oxidation profile under a low-protein diet. American Journal of Physiology. Endocrinology and Metabolism 317 (6):E1015–E1021. doi: 10.1152/ajpendo.00022.2019.
- Chandler, R. J., P. M. Zerfas, S. Shanske, J. Sloan, V. Hoffmann, S. DiMauro, and C. P. Venditti. 2009. Mitochondrial dysfunction in mut methylmalonic acidemia. FASEB Journal 23 (4):1252–1261. doi: 10.1096/fj.08-121848.
- Chen, Q., and R. A. Reimer. 2009. Dairy protein and leucine alter GLP-1 release and MRNA of genes involved in intestinal lipid metabolism in vitro. Nutrition 25 (3):340–349. doi: 10.1016/j.nut.2008.08.012.
- Cheng, Q., V. D. Beltran, S. M. H. Chan, J. R. Brown, A. Bevington, and T. P. Herbert. 2016. System-L Amino acid transporters play a key role in pancreatic β-cell signalling and function. Journal of Molecular Endocrinology 56 (3):175–187. doi: 10.1530/JME-15-0212.
- Cheng, Q., N. Shah, A. Bröer, S. Fairweather, Y. Jiang, D. Schmoll, B. Corry, and S. Bröer. 2017. Identification of novel inhibitors of the amino acid transporter B0 AT1 (SLC6A19), a potential target to induce protein restriction and to treat type 2 diabetes. British Journal of Pharmacology 174 (6):468–482. doi: 10.1111/bph.13711.
- Cheng, Y., Q. Meng, C. Wang, H. Li, Z. Huang, S. Chen, F. Xiao, and F. Guo. 2010. Leucine deprivation decreases fat mass by stimulation of lipolysis in white adipose tissue and upregulation of uncoupling protein 1 (UCP1) in brown adipose tissue. Diabetes 59 (1):17–25. doi: 10.2337/db09-0929.
- Choi, S., B. Disilvio, M. H. Fernstrom, and J. D. Fernstrom. 2013. Oral branched-chain amino acid supplements that reduce brain serotonin during exercise in rats also lower brain catecholamines. Amino Acids 45 (5):1133–1142. doi: 10.1007/s00726-013-1566-1.
- Cifarelli, V., S. C. Beeman, G. I. Smith, J. Yoshino, D. Morozov, J. W. Beals, B. D. Kayser, J. D. Watrous, M. Jain, B. W. Patterson, et al. 2020. Decreased adipose tissue oxygenation associates with insulin resistance in individuals with obesity. The Journal of Clinical Investigation 130 (12):6688–6699. doi: 10.1172/jci141828.
- Connelly, M. A., J. Wolak-Dinsmore, and R. P. F. Dullaart. 2017. Branched chain amino acids are associated with insulin resistance independent of leptin and adiponectin in subjects with varying degrees of glucose tolerance. Metabolic Syndrome and Related Disorders 15 (4):183–186. doi: 10.1089/met.2016.0145.
- Coppola, A., B. R. Wenner, O. Ilkayeva, R. D. Stevens, M. Maggioni, T. A. Slotkin, E. D. Levin, and C. B. Newgard. 2013. Branched-chain amino acids alter neurobehavioral function in rats. American Journal of Physiology. Endocrinology and Metabolism 304 (4):E405–E413. doi: 10.1152/ajpendo.00373.2012.
- Coqueiro, A. Y., M. M. Rogero, and J. Tirapegui. 2019. Glutamine as an anti-fatigue amino acid in sports nutrition. Nutrients 11 (4):863. doi: 10.3390/nu040863.
- Cormerais, Y., S. Giuliano, R. LeFloch, B. Front, J. Durivault, E. Tambutté, P.-A. Massard, L. R. de la Ballina, H. Endou, M. F. Wempe, et al. 2016. Genetic disruption of the multifunctional CD98/LAT1 complex demonstrates the key role of essential amino acid transport in the control of MTORC1 and tumor growth. Cancer Research 76 (15):4481–4492. doi: 10.1158/0008-5472.CAN-15-3376.
- Cornu, M., W. Oppliger, V. Albert, A. M. Robitaille, F. Trapani, L. Quagliata, T. Fuhrer, U. Sauer, L. Terracciano, and M. N. Hall. 2014. Hepatic MTORC1 controls locomotor activity, body temperature, and lipid metabolism through FGF21. Proceedings of the National Academy of Sciences of the United States of America 111 (32):11592–11599. doi: 10.1073/pnas.1412047111.
- Costanzo, M., M. Caterino, A. Cevenini, V. Jung, C. Chhuon, J. Lipecka, R. Fedele, I. C. Guerrera, and M. Ruoppolo. 2020. Proteomics reveals that methylmalonyl-coa mutase modulates cell architecture and increases susceptibility to stress. International Journal of Molecular Sciences 21 (14):4998. doi: 10.3390/ijms21144998.
- Crossland, H., K. Smith, I. Idris, B. E. Phillips, P. J. Atherton, and D. J. Wilkinson. 2020. Exploring mechanistic links between extracellular BCAA & muscle insulin resistance: An in vitro approach. American Journal of Physiology-Cell Physiology 319 (6):C1151–C1157. doi: 10.1152/ajpcell.00377.2020.
- Cummings, N. E., E. M. Williams, I. Kasza, E. N. Konon, M. D. Schaid, B. A. Schmidt, C. Poudel, D. S. Sherman, D. Yu, S. I. Arriola Apelo, et al. 2018. Restoration of metabolic health by decreased consumption of branched-chain amino acids. The Journal of Physiology 596 (4):623–645. doi: 10.1113/JP275075.
- Curtis, J. M., W. S. Hahn, M. D. Stone, J. J. Inda, D. J. Droullard, J. P. Kuzmicic, M. A. Donoghue, E. K. Long, A. G. Armien, S. Lavandero, et al. 2012. Protein carbonylation and adipocyte mitochondrial function. The Journal of Biological Chemistry 287 (39):32967–32980. doi: 10.1074/jbc.M112.400663.
- D’Antona, G., M. Ragni, A. Cardile, L. Tedesco, M. Dossena, F. Bruttini, F. Caliaro, G. Corsetti, R. Bottinelli, M. O. Carruba, et al. 2010. Branched-chain amino acid supplementation promotes survival and supports cardiac and skeletal muscle mitochondrial biogenesis in middle-aged mice. Cell Metabolism 12 (4):362–372. doi: 10.1016/j.cmet.2010.08.016.
- Dam, G., P. Ott, N. K. Aagaard, and H. Vilstrup. 2013. Branched-chain amino acids and muscle ammonia detoxification in cirrhosis. Metabolic Brain Disease 28 (2):217–220. doi: 10.1007/s11011-013-9377-3.
- Danthi, S. J., B. Liang, O. Smicker, B. Coupland, J. Gregory, E. Gefteas, D. Tietz, H. Klodnitsky, K. Randall, A. Belanger, et al. 2019. Identification and characterization of inhibitors of a neutral amino acid transporter, SLC6A19, using two functional cell-based assays. SLAS Discovery 24 (2):111–120. doi: 10.1177/2472555218794627.
- David, J., D. Dardevet, L. Mosoni, I. Savary-Auzeloux, and S. Polakof. 2019. Impaired skeletal muscle branched-chain amino acids catabolism contributes to their increased circulating levels in a non-obese insulin-resistant fructose-fed rat model. Nutrients 11 (2):355. doi: 10.3390/nu11020355.
- Del Coco, L., D. Vergara, S. De Matteis, E. Mensà, J. Sabbatinelli, F. Prattichizzo, A. R. Bonfigli, et al. 2019. NMR-based metabolomic approach tracks potential serum biomarkers of disease progression in patients with type 2 diabetes mellitus. Journal of Clinical Medicine 8 (5):720. doi: 10.3390/jcm8050720.
- Devries, M. C., C. McGlory, D. R. Bolster, A. Kamil, M. Rahn, L. Harkness, S. K. Baker, and S. M. Phillips. 2018. Leucine, not total protein, content of a supplement is the primary determinant of muscle protein anabolic responses in healthy older women. The Journal of Nutrition 148 (7):1088–1095. doi: 10.1093/jn/nxy091.
- Dewulf, E. M., P. D. Cani, S. P. Claus, S. Fuentes, P. G. B. Puylaert, A. M. Neyrinck, L. B. Bindels, W. M. de Vos, G. R. Gibson, J.-P. Thissen, et al. 2013. Insight into the prebiotic concept: Lessons from an exploratory, double blind intervention study with inulin-type fructans in obese women. Gut 62 (8):1112–1121. doi: 10.1136/gutjnl-2012-303304.
- Dhanani, Z. N., G. Mann, and O. A. J. Adegoke. 2019. Depletion of branched-chain aminotransferase 2 (BCAT2) enzyme impairs myoblast survival and myotube formation. Physiological Reports 7 (23):e14299. doi: 10.14814/phy2.14299.
- Dickinson, J. M., C. S. Fry, M. J. Drummond, D. M. Gundermann, D. K. Walker, E. L. Glynn, K. L. Timmerman, S. Dhanani, E. Volpi, and B. B. Rasmussen. 2011. Mammalian target of rapamycin complex 1 activation is required for the stimulation of human skeletal muscle protein synthesis by essential amino acids. The Journal of Nutrition 141 (5):856–862. doi: 10.3945/jn.111.139485.
- Doisaki, M., Y. Katano, I. Nakano, Y. Hirooka, A. Itoh, M. Ishigami, K. Hayashi, H. Goto, Y. Fujita, Y. Kadota, et al. 2010. Regulation of hepatic branched-chain alpha-keto acid dehydrogenase kinase in a rat model for type 2 diabetes mellitus at different stages of the disease. Biochemical and Biophysical Research Communications 393 (2):303–307. doi: 10.1016/j.bbrc.2010.02.004.
- Drummond, M. J., P. T. Reidy, L. M. Baird, B. K. Dalley, and M. T. Howard. 2017. Leucine differentially regulates gene-specific translation in mouse skeletal muscle. The Journal of Nutrition 147 (9):jn251181. doi: 10.3945/jn.117.251181.
- Du, Y., Q. Meng, Q. Zhang, and F. Guo. 2012. Isoleucine or valine deprivation stimulates fat loss via increasing energy expenditure and regulating lipid metabolism in WAT. Amino Acids 43 (2):725–734. doi: 10.1007/s00726-011-1123-8.
- Elshorbagy, A. K., D. Samocha-Bonet, F. Jernerén, C. Turner, H. Refsum, and L. K. Heilbronn. 2018. Food overconsumption in healthy adults triggers early and sustained increases in serum branched-chain amino acids and changes in cysteine linked to fat gain. The Journal of Nutrition 148 (7):1073–1080. doi: 10.1093/jn/nxy062.
- Engin, A. 2017. Adipose tissue hypoxia in obesity and its impact on preadipocytes and macrophages: Hypoxia hypothesis. Advances in Experimental Medicine and Biology 960:305–326. doi: 10.1007/978-3-319-48382-5_13.
- Estrada-Alcalde, I., M. R. Tenorio-Guzman, A. R. Tovar, D. Salinas-Rubio, I. Torre-Villalvazo, N. Torres, and L. G. Noriega. 2017. Metabolic fate of branched-chain amino acids during adipogenesis, in adipocytes from obese mice and C2C12 myotubes. Journal of Cellular Biochemistry 118 (4):808–818. doi: 10.1002/jcb.25755.
- Everman, S., C. Meyer, L. Tran, N. Hoffman, C. C. Carroll, W. L. Dedmon, and C. S. Katsanos. 2016. Insulin does not stimulate muscle protein synthesis during increased plasma branched-chain amino acids alone but still decreases whole body proteolysis in humans. American Journal of Physiology-Endocrinology and Metabolism 311 (4):E671–E677. doi: 10.1152/ajpendo.00120.2016.
- Falavigna, G., J. Alves de Araújo, M. M. Rogero, I. S. d O. Pires, R. G. Pedrosa, E. Martins, I. Alves de Castro, and J. Tirapegui. 2012. Effects of diets supplemented with branched-chain amino acids on the performance and fatigue mechanisms of rats submitted to prolonged physical exercise. Nutrients 4 (11):1767–1780. doi: 10.3390/nu4111767.
- Feng, B., C. Banner, and S. R. Max. 1990. Effect of diabetes on glutamine synthetase expression in rat skeletal muscles. American Journal of Physiology - Endocrinology and Metabolism 258 (5 Pt 1):E762–E766. doi: 10.1152/ajpendo.1990.258.5.e762.
- Feng, X., A. Sureda, S. Jafari, Z. Memariani, D. Tewari, G. Annunziata, L. Barrea, S. T. S. Hassan, K. Šmejkal, M. Malaník, et al. 2019. Berberine in cardiovascular and metabolic diseases: From mechanisms to therapeutics. Theranostics 9 (7):1923–1951. doi: 10.7150/thno.30787.
- Ferriero, R., and N. Brunetti-Pierri. 2013. Phenylbutyrate increases activity of pyruvate dehydrogenase complex. Oncotarget 4 (6):804–805. doi: 10.18632/oncotarget.1000.
- Festa, A., K. Williams, R. P. Tracy, L. E. Wagenknecht, and S. M. Haffner. 2006. Progression of plasminogen activator inhibitor-1 and fibrinogen levels in relation to incident type 2 diabetes. Circulation 113 (14):1753–1759. doi: 10.1161/CIRCULATIONAHA.106.616177.
- Fiehn, O., W. T. Garvey, J. W. Newman, K. H. Lok, C. L. Hoppel, and S. H. Adams. 2010. Plasma metabolomic profiles reflective of glucose homeostasis in non-diabetic and type 2 diabetic obese African-American women. PLoS One 5 (12):e15234. doi: 10.1371/journal.pone.0015234.
- Fontana, L., N. E. Cummings, S. I. Arriola Apelo, J. C. Neuman, I. Kasza, B. A. Schmidt, E. Cava, F. Spelta, V. Tosti, F. A. Syed, et al. 2016. Decreased consumption of branched-chain amino acids improves metabolic health. Cell Reports 16 (2):520–530. doi: 10.1016/j.celrep.2016.05.092.
- Foster, D. W. 2012. Malonyl-CoA: The regulator of fatty acid synthesis and oxidation. Journal of Clinical Investigation 122 (6):1958–1959. doi: 10.1172/JCI63967.
- Fouré, A., and D. Bendahan. 2017. Is branched-chain amino acids supplementation an efficient nutritional strategy to alleviate skeletal muscle damage? A systematic review. Nutrients 9 (10):1047. doi: 10.3390/nu9101047.
- Freudenberg, A., K. J. Petzke, and S. Klaus. 2013. Dietary L-leucine and L-alanine supplementation have similar acute effects in the prevention of high-fat diet-induced obesity. Amino Acids 44 (2):519–528. doi: 10.1007/s00726-012-1363-2.
- Fryburg, D. A., E. J. Barrett, R. J. Louard, and R. A. Gelfand. 1990. Effect of starvation on human muscle protein metabolism and its response to insulin. The American Journal of Physiology 259 (4 Pt 1):E477–E482. doi: 10.1152/ajpendo.1990.259.4.e477.
- Fu, L., A. Bruckbauer, F. Li, Q. Cao, X. Cui, R. Wu, H. Shi, M. B. Zemel, and B. Xue. 2015. Leucine amplifies the effects of metformin on insulin sensitivity and glycemic control in diet-induced obese mice. Metabolism 64 (7):845–856. doi: 10.1016/j.metabol.2015.03.007.
- Fu, W., and M. N. Hall. 2020. Regulation of MTORC2 signaling. Genes 11 (9):1045. doi: 10.3390/genes11091045.
- Gannaban, R. B., C. NamKoong, H. H. Ruiz, H. J. Choi, and A. C. Shin. 2021. Central regulation of branched-chain amino acids is mediated by AgRP neurons. Diabetes 70 (1):62–75. doi: 10.2337/db20-0510.
- Gawedzka, A., M. Grandys, K. Duda, J. Zapart-Bukowska, J. A. Zoladz, and J. Majerczak. 2020. Plasma BCAA concentrations during exercise of varied intensities in young healthy men-the impact of endurance training. PeerJ 8 (December):e10491. doi: 10.7717/peerj.10491.
- Ghoraba, D. A., M. M. Mohammed, and O. K. Zaki. 2015. Mutation analysis of methylmalonyl CoA mutase gene exon 2 in Egyptian families: Identification of 25 novel allelic variants. Meta Gene 3 (February):71–88. doi: 10.1016/j.mgene.2014.02.001.
- Gibson, R., Y. Zhao, J. Jaskiewicz, S. E. Fineberg, and R. A. Harris. 1993. Effects of diabetes on the activity and content of the branched-chain alpha-ketoacid dehydrogenase complex in liver. Archives of Biochemistry and Biophysics 306 (1):22–28. doi: 10.1006/abbi.1993.1475.
- Gluud, L. L., G. Dam, M. Borre, I. Les, J. Cordoba, G. Marchesini, N. K. Aagaard, and H. Vilstrup. 2013. Lactulose, rifaximin or branched chain amino acids for hepatic encephalopathy: What is the evidence?Metabolic Brain Disease 28 (2):221–225. doi: 10.1007/s11011-012-9372-0.
- Gluud, L. L., G. Dam, I. Les, G. Marchesini, M. Borre, N. K. Aagaard, and H. Vilstrup. 2017. Branched-chain amino acids for people with hepatic encephalopathy. The Cochrane Database of Systematic Reviews 5:CD001939. doi: 10.1002/14651858.CD001939.pub4.
- Goldberg, E. J., K. A. Buddo, K. L. McLaughlin, R. F. Fernandez, A. S. Pereyra, C. E. Psaltis, C.-T. Lin, J. T. Hagen, I. N. Boykov, T. K. Nguyen, et al. 2019. Tissue-specific characterization of mitochondrial branched-chain keto acid oxidation using a multiplexed assay platform. The Biochemical Journal 476 (10):1521–1537. doi: 10.1042/BCJ20190182.
- Gray, S., B. Wang, Y. Orihuela, E.-G. Hong, S. Fisch, S. Haldar, G. W. Cline, J. K. Kim, O. D. Peroni, B. B. Kahn, et al. 2007. Regulation of gluconeogenesis by Krüppel-like factor 15. Cell Metabolism 5 (4):305–312. doi: 10.1016/j.cmet.2007.03.002.
- Green, C. R., M. Wallace, A. S. Divakaruni, S. A. Phillips, A. N. Murphy, T. P. Ciaraldi, and C. M. Metallo. 2016. Branched-chain amino acid catabolism fuels adipocyte differentiation and lipogenesis. Nature Chemical Biology 12 (1):15–21. doi: 10.1038/nchembio.1961.
- Grimsrud, P. A., M. J. Picklo, T. J. Griffin, and D. A. Bernlohr. 2007. Carbonylation of adipose proteins in obesity and insulin resistance: Identification of adipocyte fatty acid-binding protein as a cellular target of 4-hydroxynonenal. Molecular & Cellular Proteomics 6 (4):624–637. doi: 10.1074/mcp.M600120-MCP200.
- Guo, F., and D. R. Cavener. 2007. The GCN2 eIF2alpha kinase regulates fatty-acid homeostasis in the liver during deprivation of an essential amino acid. Cell Metabolism 5 (2):103–114. doi: 10.1016/j.cmet.2007.01.001.
- Halford, J. C. G., J. A. Harrold, E. J. Boyland, C. L. Lawton, and J. E. Blundell. 2007. Serotonergic drugs: Effects on appetite expression and use for the treatment of obesity. Drugs 67 (1):27–55. doi: 10.2165/00003495-200767010-00004.
- Han, J. L., and H. L. Lin. 2014. Intestinal microbiota and type 2 diabetes: From mechanism insights to therapeutic perspective. World Journal of Gastroenterology 20 (47):17737–17745. doi: 10.3748/wjg.v20.i47.17737.
- Harris, L., L. S. Ann, G. I. Smith, B. W. Patterson, R. S. Ramaswamy, A. L. Okunade, S. C. Kelly, L. C. Porter, S. Klein, J. Yoshino, et al. 2017. Alterations in 3-hydroxyisobutyrate and FGF21 metabolism are associated with protein ingestion-induced insulin resistance. Diabetes 66 (7):1871–1878. doi: 10.2337/db16-1475.
- Harris, R. A., R. Kobayashi, T. Murakami, and Y. Shimomura. 2001. Regulation of branched-Chain alpha-keto acid dehydrogenase kinase expression in rat liver. The Journal of Nutrition 131 (3):841S–845S. doi: 10.1093/jn/131.3.841S.
- Hayase, S., K. Kumamoto, K. Saito, Y. Kofunato, Y. Sato, H. Okayama, K. Miyamoto, S. Ohki, and S. Takenoshita. 2017. L-type amino acid transporter 1 expression is upregulated and associated with cellular proliferation in colorectal cancer. Oncology Letters 14 (6):7410–7416. doi: 10.3892/ol.2017.7148.
- Herman, M. A., P. She, O. D. Peroni, C. J. Lynch, and B. B. Kahn. 2010. Adipose tissue branched chain amino acid (BCAA) metabolism modulates circulating BCAA levels. The Journal of Biological Chemistry 285 (15):11348–11356. doi: 10.1074/jbc.M109.075184.
- Hernández-Alvarez, M. I., A. Díaz-Ramos, M. Berdasco, J. Cobb, E. Planet, D. Cooper, A. Pazderska, K. Wanic, D. O’Hanlon, A. Gomez, et al. 2017. Early-onset and classical forms of type 2 diabetes show impaired expression of genes involved in muscle branched-chain amino acids metabolism. Scientific Reports 7 (1):13850. doi: 10.1038/s41598-017-14120-6.
- Hewton, K. G., A. S. Johal, and S. J. Parker. 2021. Transporters at the interface between cytosolic and mitochondrial amino acid metabolism. Metabolites 11(2):112. doi: 10.3390/metabo11020112.
- Higuchi, N., M. Kato, M. Miyazaki, M. Tanaka, M. Kohjima, T. Ito, M. Nakamuta, M. Enjoji, K. Kotoh, and R. Takayanagi. 2011. Potential role of branched-chain amino acids in glucose metabolism through the accelerated induction of the glucose-sensing apparatus in the liver. Journal of Cellular Biochemistry 112 (1):30–38. doi: 10.1002/jcb.22688.
- Holecek, M. 2013. Branched-chain amino acids and ammonia metabolism in liver disease: Therapeutic implications. Nutrition 29 (10):1186–1191. doi: 10.1016/j.nut.2013.01.022.
- Holeček, M. 2018. Branched-chain amino acids in health and disease: Metabolism, alterations in blood plasma, and as supplements. Nutrition & Metabolism 15 (1):33. doi: 10.1186/s12986-018-0271-1.
- Holeček, M. 2020. Why are branched-chain amino acids increased in starvation and diabetes?Nutrients 12 (10):3087. doi: 10.3390/nu12103087.
- Holecek, M., R. Kandar, L. Sispera, and M. Kovarik. 2011. Acute hyperammonemia activates branched-chain amino acid catabolism and decreases their extracellular concentrations: Different sensitivity of red and white muscle. Amino Acids 40 (2):575–584. doi: 10.1007/s00726-010-0679-z.
- Horiuchi, M., T. Takeda, H. Takanashi, Y. Ozaki-Masuzawa, Y. Taguchi, Y. Toyoshima, L. Otani, H. Kato, M. Sone-Yonezawa, F. Hakuno, et al. 2017. Branched-chain amino acid supplementation restores reduced insulinotropic activity of a low-protein diet through the vagus nerve in rats. Nutrition & Metabolism 14 (1):59. doi: 10.1186/s12986-017-0215-1.
- Howard, J. K., and J. S. Flier. 2006. Attenuation of leptin and insulin signaling by SOCS proteins. Trends in Endocrinology and Metabolism 17 (9):365–371. doi: 10.1016/j.tem.2006.09.007.
- Howell, J. J., K. Hellberg, M. Turner, G. Talbott, M. J. Kolar, D. S. Ross, G. Hoxhaj, A. Saghatelian, R. J. Shaw, and B. D. Manning. 2017. Metformin inhibits hepatic MTORC1 signaling via dose-dependent mechanisms involving AMPK and the TSC complex. Cell Metabolism 25 (2):463–471. doi: 10.1016/j.cmet.2016.12.009.
- Hsiao, G., J. Chapman, J. M. Ofrecio, J. Wilkes, J. L. Resnik, D. Thapar, S. Subramaniam, and D. D. Sears. 2011. Multi-tissue, selective PPARγ modulation of insulin sensitivity and metabolic pathways in obese rats. American Journal of Physiology. Endocrinology and Metabolism 300 (1):E164–E174. doi: 10.1152/ajpendo.00219.2010.
- Hu, H., L. Li, C. Wang, H. He, K. Mao, X. Ma, R. Shi, Y. Oh, FWei Zhang, Y. Lu, et al. 2014. 4-Phenylbutyric acid increases GLUT4 gene expression through suppression of HDAC5 but not endoplasmic reticulum stress. Cellular Physiology and Biochemistry 33 (6):1899–1910. doi: 10.1159/000362967.
- Iannitti, T., and B. Palmieri. 2011. Clinical and experimental applications of sodium phenylbutyrate. Drugs in R&D 11 (3):227–249. doi: 10.2165/11591280-000000000-00000.
- Isanejad, M., A. Z. LaCroix, C. A. Thomson, L. Tinker, J. C. Larson, Q. Qi, L. Qi, R. M. Cooper-DeHoff, L. S. Phillips, R. L. Prentice, et al. 2017. Branched-chain amino acid, meat intake and risk of type 2 diabetes in the women’s health initiative. The British Journal of Nutrition 117 (11):1523–30. doi: 10.1017/S0007114517001568.
- Ishikawa, T., Y. Kitaura, Y. Kadota, Y. Morishita, M. Ota, F. Yamanaka, M. Xu, M. Ikawa, N. Inoue, F. Kawano, et al. 2017. Muscle-specific deletion of BDK amplifies loss of myofibrillar protein during protein undernutrition. Scientific Reports 7 (1):39825. doi: 10.1038/srep39825.
- Iwasa, M., T. Ishihara, R. Mifuji-Moroka, N. Fujita, Y. Kobayashi, H. Hasegawa, K. Iwata, M. Kaito, and Y. Takei. 2015. Elevation of branched-chain amino acid levels in diabetes and NAFL and changes with antidiabetic drug treatment. Obesity Research & Clinical Practice 9 (3):293–7. doi: 10.1016/j.orcp.2015.01.003.
- Jackman, S. R., O. C. Witard, A. Philp, G. A. Wallis, K. Baar, and K. D. Tipton. 2017. Branched-chain amino acid ingestion stimulates muscle myofibrillar protein synthesis following resistance exercise in humans. Frontiers in Physiology 8 (JUN):390. doi: 10.3389/fphys.2017.00390.
- Jang, C., S. F. Oh, S. Wada, G. C. Rowe, L. Liu, M. C. Chan, J. Rhee, A. Hoshino, B. Kim, A. Ibrahim, et al. 2016. A branched-chain amino acid metabolite drives vascular fatty acid transport and causes insulin resistance. Nature Medicine 22 (4):421–6. doi: 10.1038/nm.4057.
- Javed, K., and S. J. Fairweather. 2019. Amino acid transporters in the regulation of insulin secretion and signalling. Biochemical Society Transactions 47 (2):571–90. doi: 10.1042/BST20180250.
- Jennings, A., A. MacGregor, T. Pallister, T. Spector, and A. Cassidy. 2016. Associations between branched chain amino acid intake and biomarkers of adiposity and cardiometabolic health independent of genetic factors: A twin study. International Journal of Cardiology 223 (November):992–8. doi: 10.1016/j.ijcard.2016.08.307.
- Jhanwar-Uniyal, M., J. V. Wainwright, A. L. Mohan, M. E. Tobias, R. Murali, C. D. Gandhi, and M. H. Schmidt. 2019. Diverse signaling mechanisms of MTOR complexes: MTORC1 and MTORC2 in forming a formidable relationship. Advances in Biological Regulation 72 (May):51–62. doi: 10.1016/j.jbior.2019.03.003.
- Jiang, Y., A. J. Rose, T. P. Sijmonsma, A. Bröer, A. Pfenninger, S. Herzig, D. Schmoll, and S. Bröer. 2015. Mice lacking neutral amino acid transporter B(0)AT1 (Slc6a19) have elevated levels of FGF21 and GLP-1 and improved glycaemic control. Molecular Metabolism 4 (5):406–17. doi: 10.1016/j.molmet.2015.02.003.
- Jiao, J., S.-F. Han, W. Zhang, J.-Y. Xu, X. Tong, X.-B. Yin, L.-X. Yuan, and L.-Q. Qin. 2016. Chronic leucine supplementation improves lipid metabolism in C57BL/6J mice fed with a high-fat/cholesterol diet. Food & Nutrition Research 60 (September):31304. doi: 10.3402/fnr.v60.31304.
- Jung, T. W., H.-J. Hwang, H. C. Hong, H. J. Yoo, S. H. Baik, and K. M. Choi. 2015. BAIBA attenuates insulin resistance and inflammation induced by palmitate or a high fat diet via an AMPK-PPARδ-dependent pathway in mice. Diabetologia 58 (9):2096–105. doi: 10.1007/s00125-015-3663-z.
- Jung, T. W., H. S. Park, G. H. Choi, D. Kim, and T. Lee. 2018. β-aminoisobutyric acid attenuates LPS-induced inflammation and insulin resistance in adipocytes through AMPK-mediated pathway. Journal of Biomedical Science 25 (1):27. doi: 10.1186/s12929-018-0431-7.
- Kadota, Y., S. Kazama, G. Bajotto, Y. Kitaura, and Y. Shimomura. 2012. Clofibrate-induced reduction of plasma branched-chain amino acid concentrations impairs glucose tolerance in rats. JPEN. Journal of Parenteral and Enteral Nutrition 36 (3):337–43. doi: 10.1177/0148607111414578.
- Kadota, Y., T. Toyoda, Y. Kitaura, S. H. Adams, and Y. Shimomura. 2013. Regulation of hepatic branched-chain α-ketoacid dehydrogenase complex in rats fed a high-fat diet. Obesity Research & Clinical Practice 7 (6):e439–e444. doi: 10.1016/j.orcp.2013.07.003.
- Kainulainen, H., J. J. Hulmi, and U. M. Kujala. 2013. Potential role of branched-chain amino acid catabolism in regulating fat oxidation. Exercise and Sport Sciences Reviews 41 (4):194–200. doi: 10.1097/JES.0b013e3182a4e6b6.
- Kalavalapalli, S., F. Bril, J. P. Koelmel, K. Abdo, J. Guingab, P. Andrews, W.-Y. Li, D. Jose, R. A. Yost, R. F. Frye, et al. 2018. Pioglitazone improves hepatic mitochondrial function in a mouse model of nonalcoholic steatohepatitis. American Journal of Physiology. Endocrinology and Metabolism 315 (2):E163–E173. doi: 10.1152/ajpendo.00023.2018.
- Kamei, Y., Y. Hatazawa, R. Uchitomi, R. Yoshimura, and S. Miura. 2020. Regulation of skeletal muscle function by amino acids. Nutrients 12 (1):261. doi: 10.3390/nu12010261.
- Kang, S., E. R. Chemaly, R. J. Hajjar, and D. Lebeche. 2011. Resistin promotes cardiac hypertrophy via the AMP-activated protein kinase/mammalian target of rapamycin (AMPK/MTOR) and c-Jun N-terminal kinase/insulin receptor substrate 1 (JNK/IRS1) pathways. The Journal of Biological Chemistry 286 (21):18465–73. doi: 10.1074/jbc.M110.200022.
- Kappel, B. A., M. Lehrke, K. Schütt, A. Artati, J. Adamski, C. Lebherz, and N. Marx. 2017. Effect of empagliflozin on the metabolic signature of patients with type 2 diabetes mellitus and cardiovascular disease. Circulation 136 (10):969–72. doi: 10.1161/CIRCULATIONAHA.117.029166.
- Karakas, S. E., B. Perroud, T. Kind, M. Palazoglu, and O. Fiehn. 2016. Changes in plasma metabolites and glucose homeostasis during omega-3 polyunsaturated fatty acid supplementation in women with polycystic ovary syndrome. BBA Clinical 5:179–85. doi: 10.1016/j.bbacli.2016.04.003.
- Karusheva, Y., T. Koessler, K. Strassburger, D. Markgraf, L. Mastrototaro, T. Jelenik, M.-C. Simon, D. Pesta, O.-P. Zaharia, K. Bódis, et al. 2019. Short-term dietary reduction of branched-chain amino acids reduces meal-induced insulin secretion and modifies microbiome composition in type 2 diabetes: A randomized controlled crossover trial. The American Journal of Clinical Nutrition 110 (5):1098–107. doi: 10.1093/ajcn/nqz191.
- Karusheva, Y., K. Strassburger, D. F. Markgraf, O.-P. Zaharia, K. Bódis, T. Kössler, A. Tura, G. Pacini, V. Burkart, M. Roden, et al. 2021. Branched-chain amino acids associate negatively with postprandial insulin secretion in recent-onset diabetes. Journal of the Endocrine Society 5 (6):1–9. doi: 10.1210/jendso/bvab067.
- Karwi, Q., G. Mezbah Uddin, C. S. Wagg, and G. D. Lopaschuk. 2020. Branched-chain keto acids, not branched-chain amino acids, impairs cardiac insulin sensitivity by disrupting insulin signaling in the mitochondria. Circulation Research 127 (Suppl_1):AMP125. doi: 10.1161/res.127.suppl_1.MP125.
- Katagiri, R., A. Goto, S. Budhathoki, T. Yamaji, H. Yamamoto, Y. Kato, M. Iwasaki, and S. Tsugane. 2018. Association between plasma concentrations of branched-chain amino acids and adipokines in Japanese adults without diabetes. Scientific Reports 8 (1):1043–8. doi: 10.1038/s41598-018-19388-w.
- Kawaguchi, T., Y. Nagao, H. Matsuoka, T. Ide, and M. Sata. 2008. Branched-chain amino acid-enriched supplementation improves insulin resistance in patients with chronic liver disease. International Journal of Molecular Medicine 22 (1):105–12. doi: 10.3892/ijmm.22.1.105.
- Kephart, W. C., T. D. Wachs, R. M. Thompson, C. B. Mobley, C. D. Fox, J. R. McDonald, B. S. Ferguson, K. C. Young, B. Nie, J. S. Martin, et al. 2015. Ten weeks of branched chain amino acid supplementation improves select performance and immunological variables in trained cyclists. Journal of the International Society of Sports Nutrition 12 (S1):P20. doi: 10.1186/1550-2783-12-S1-P20.
- Khan, S., S. K. Komarya, and G. Jena. 2017. Phenylbutyrate and β-cell function: Contribution of histone deacetylases and ER stress inhibition. Epigenomics 9 (5):711–20. doi: 10.2217/epi-2016-0160.
- Kitsy, A., S. Carney, J. C. Vivar, M. S. Knight, M. A. Pointer, J. K. Gwathmey, and S. Ghosh. 2014. Effects of leucine supplementation and serum withdrawal on branched-chain amino acid pathway gene and protein expression in mouse adipocytes. PLoS One 9 (7):e102615. doi: 10.1371/journal.pone.0102615.
- Knebel, B., K. Strassburger, J. Szendroedi, J. Kotzka, M. Scheer, B. Nowotny, K. Müssig, S. Lehr, G. Pacini, H. Finner, et al. 2016. Specific metabolic profiles and their relationship to insulin resistance in recent-onset type 1 and type 2 diabetes. The Journal of Clinical Endocrinology and Metabolism 101 (5):2130–40. doi: 10.1210/jc.2015-4133.
- Kobayashi, R., T. Murakami, M. Obayashi, N. Nakai, J. Jaskiewicz, Y. Fujiwara, Y. Shimomura, and R. A. Harris. 2002. Clofibric acid stimulates branched-chain amino acid catabolism by three mechanisms. Archives of Biochemistry and Biophysics 407 (2):231–40. doi: 10.1016/S0003-9861(02)00472-1.
- Kondo, H., Y. Minegishi, Y. Komine, T. Mori, I. Matsumoto, K. Abe, I. Tokimitsu, T. Hase, and T. Murase. 2006. Differential regulation of intestinal lipid metabolism-related genes in obesity-resistant A/J vs. obesity-prone C57BL/6J mice. American Journal of Physiology. Endocrinology and Metabolism 291 (5):E1092–E1099. doi: 10.1152/ajpendo.00583.2005.
- Kovatcheva-Datchary, P., A. Nilsson, R. Akrami, Y. S. Lee, F. De Vadder, T. Arora, A. Hallen, E. Martens, I. Björck, and F. Bäckhed. 2015. Dietary fiber-induced improvement in glucose metabolism is associated with increased abundance of prevotella. Cell Metabolism 22 (6):971–82. doi: 10.1016/j.cmet.2015.10.001.
- Koves, T. R., J. R. Ussher, R. C. Noland, D. Slentz, M. Mosedale, O. Ilkayeva, J. Bain, R. Stevens, J. R. B. Dyck, C. B. Newgard, et al. 2008. Mitochondrial overload and incomplete fatty acid oxidation contribute to skeletal muscle insulin resistance. Cell Metabolism 7 (1):45–56. doi: 10.1016/j.cmet.2007.10.013.
- Krebs, M., M. Krssak, E. Bernroider, C. Anderwald, A. Brehm, M. Meyerspeer, P. Nowotny, E. Roth, W. Waldhausl, and M. Roden. 2002. Mechanism of amino acid-induced skeletal muscle insulin resistance in humans. Diabetes 51 (3):599–605. doi: 10.2337/diabetes.51.3.599.
- Kujala, U. M., M. Peltonen, M. K. Laine, J. Kaprio, O. J. Heinonen, J. Sundvall, J. G. Eriksson, A. Jula, S. Sarna, and H. Kainulainen. 2016. Branched-chain amino acid levels are related with surrogates of disturbed lipid metabolism among older men. Frontiers in Medicine 3:57. doi: 10.3389/fmed.2016.00057.
- Lackey, D. E., C. J. Lynch, K. C. Olson, R. Mostaedi, M. Ali, W. H. Smith, F. Karpe, S. Humphreys, D. H. Bedinger, T. N. Dunn, et al. 2013. Regulation of adipose branched-chain amino acid catabolism enzyme expression and cross-adipose amino acid flux in human obesity. American Journal of Physiology. Endocrinology and Metabolism 304 (11):E1175–E1187. doi: 10.1152/ajpendo.00630.2012.
- Laeger, T., S. D. Reed, T. M. Henagan, D. H. Fernandez, M. Taghavi, A. Addington, H. Münzberg, R. J. Martin, S. M. Hutson, and C. D. Morrison. 2014. Leucine acts in the brain to suppress food intake but does not function as a physiological signal of low dietary protein. American Journal of Physiology-Regulatory, Integrative and Comparative Physiology 307 (3):R310–R320. doi: 10.1152/ajpregu.00116.2014.
- Laferrère, B., D. Reilly, S. Arias, N. Swerdlow, P. Gorroochurn, B. Bawa, M. Bose, J. Teixeira, R. D. Stevens, B. R. Wenner, et al. 2011. Differential metabolic impact of gastric bypass surgery versus dietary intervention in obese diabetic subjects despite identical weight loss. Science Translational Medicine 3 (80):80re2. doi: 10.1126/scitranslmed.3002043.
- Lee, C. C., Watkins, S. M. C. Lorenzo, L. E. Wagenknecht, D. Il’Yasova, Yii, D. I. Chen, S. M. Haffner, and A. J. Hanley. 2016. Branched-chain amino acids and insulin metabolism: The insulin resistance atherosclerosis study (IRAS). Diabetes Care 39 (4):582–8. doi: 10.2337/dc15-2284.
- Lee, J., A. Vijayakumar, P. J. White, Y. Xu, O. Ilkayeva, C. J. Lynch, C. B. Newgard, and B. B. Kahn. 2021. BCAA supplementation in mice with diet-induced obesity alters the metabolome without impairing glucose homeostasis. Endocrinology 162 (7):bqab062. doi: 10.1210/endocr/bqab062.
- Lee, J. H., Y.-R. Cho, J. H. Kim, J. Kim, H. Y. Nam, S. W. Kim, and J. Son. 2019. Branched-chain amino acids sustain pancreatic cancer growth by regulating lipid metabolism. Experimental & Molecular Medicine 51 (11):1–11. doi: 10.1038/s12276-019-0350-z.
- Lerin, C., A. B. Goldfine, T. Boes, M. Liu, S. Kasif, J. M. Dreyfuss, A. L. De Sousa-Coelho, G. Daher, I. Manoli, J. R. Sysol, et al. 2016. Defects in muscle branched-chain amino acid oxidation contribute to impaired lipid metabolism. Molecular Metabolism 5 (10):926–36. doi: 10.1016/j.molmet.2016.08.001.
- Li, D., S. Li, Q. Pan, H. Zhai, M. Peng, X. Wang, and G. Xu. 2018. Gastric mammalian target of rapamycin signaling contributes to inhibition of ghrelin expression induced by Roux-En-Y gastric bypass. Cellular Physiology and Biochemistry 51 (2):664–80. doi: 10.1159/000495325.
- Li, T., Z. Zhang, S. C. Kolwicz, L. Abell, N. D. Roe, M. Kim, B. Zhou, Y. Cao, J. Ritterhoff, H. Gu, et al. 2017. Defective branched-chain amino acid catabolism disrupts glucose metabolism and sensitizes the heart to ischemia-reperfusion injury. Cell Metabolism 25 (2):374–85. doi: 10.1016/j.cmet.2016.11.005.
- Li, X., X. Wang, R. Liu, Y. Ma, H. Guo, L. Hao, P. Yao, L. Liu, X. Sun, K. He, et al. 2013. Chronic leucine supplementation increases body weight and insulin sensitivity in rats on high-fat diet likely by promoting insulin signaling in insulin-target tissues. Molecular Nutrition & Food Research 57 (6):1067–79. doi: 10.1002/mnfr.201200311.
- Li, Y., Wang, B. J. Shen, M. Bai, E. Xu. 2020. Berberine attenuates fructose-induced insulin resistance by stimulating the hepatic LKB1/AMPK/PGC-1α pathway in mice. Pharmaceutical Biology 58 (1):385–92. doi: 10.1080/13880209.2020.1756349.
- Li, Y., Z. Xiong, W. Yan, E. Gao, H. Cheng, G. Wu, Y. Liu, L. Zhang, C. Li, S. Wang, et al. 2020. Branched chain amino acids exacerbate myocardial ischemia/reperfusion vulnerability via enhancing GCN2/ATF6/PPAR-α pathway-dependent fatty acid oxidation. Theranostics 10 (12):5623–40. doi: 10.7150/thno.44836.
- Lian, K., C. Du, Y. Liu, D. Zhu, W. Yan, H. Zhang, Z. Hong, P. Liu, L. Zhang, H. Pei, et al. 2015. Impaired adiponectin signaling contributes to disturbed catabolism of branched-chain amino acids in diabetic mice. Diabetes 64 (1):49–59. doi: 10.2337/db14-0312.
- Liao, X., B. Liu, H. Qu, L. Zhang, Y. Lu, Y. Xu, Z. Lyu, and H. Zheng. 2019. A high level of circulating valine is a biomarker for type 2 diabetes and associated with the hypoglycemic effect of sitagliptin. Mediators of Inflammation 2019:1–7. doi: 10.1155/2019/824019.
- Lin, H. V., A. Frassetto, E. J. Kowalik, A. R. Nawrocki, M. M. Lu, J. R. Kosinski, J. A. Hubert, D. Szeto, X. Yao, G. Forrest, et al. 2012. Butyrate and propionate protect against diet-induced obesity and regulate gut hormones via free fatty acid receptor 3-independent mechanisms. PLoS One 7 (4):e35240. doi: 10.1371/journal.pone.0035240.
- Liu, H., R. Liu, Y. Xiong, X. Li, X. Wang, Y. Ma, H. Guo, L. Hao, P. Yao, L. Liu, et al. 2014. Leucine facilitates the insulin-stimulated glucose uptake and insulin signaling in skeletal muscle cells: Involving MTORC1 and MTORC2. Amino Acids 46 (8):1971–9. doi: 10.1007/s00726-014-1752-9.
- Liu, R., H. Li, W. Fan, Q. Jin, T. Chao, Y. Wu, J. Huang, L. Hao, and X. Yang. 2017. Leucine supplementation differently modulates branched-chain amino acid catabolism, mitochondrial function and metabolic profiles at the different stage of insulin resistance in rats on high-fat diet. Nutrients 9 (6):565. doi: 10.3390/nu9060565.
- Liu, Y., W. Dong, J. Shao, Y. Wang, M. Zhou, and H. Sun. 2017. Branched-chain amino acid negatively regulates KLF15 expression via PI3K-AKT pathway. Frontiers in Physiology 8 (OCT):853. doi: 10.3389/fphys.2017.00853.
- Liu, Y., Y. Wang, Y. Ni, C. K. Y. Cheung, K. S. L. Lam, Y. Wang, Z. Xia, D. Ye, J. Guo, M. A. Tse, et al. 2020. Gut microbiome fermentation determines the efficacy of exercise for diabetes prevention. Cell Metabolism 31 (1):77–91.e5. doi: 10.1016/j.cmet.2019.11.001.
- Long, J., Z. Yang, L. Wang, Y. Han, C. Peng, C. Yan, and D. Yan. 2020. Metabolite biomarkers of type 2 diabetes mellitus and pre-diabetes: A systematic review and meta-analysis. BMC Endocrine Disorders 20 (1):174. doi: 10.1186/s12902-020-00653-x.
- Lu, G., H. Sun, P. She, J.-Y. Youn, S. Warburton, P. Ping, T. M. Vondriska, H. Cai, C. J. Lynch, and Y. Wang. 2009. Protein phosphatase 2Cm is a critical regulator of branched-chain amino acid catabolism in mice and cultured d cells. The Journal of Clinical Investigation 119 (6):1678–87. doi: 10.1172/JCI38151.
- Lynch, C. J., and S. H. Adams. 2014. Branched-chain amino acids in metabolic signalling and insulin resistance. Nature Reviews. Endocrinology 10 (12):723–36. doi: 10.1038/nrendo.2014.171.
- Lynch, C. J., S. R. Kimball, Y. Xu, A. C. Salzberg, and Y. I. Kawasawa. 2015. Global deletion of BCATm increases expression of skeletal muscle genes associated with protein turnover. Physiological Genomics 47 (11):569–80. doi: 10.1152/physiolgenomics.00055.2015.
- Ma, Q., X. Zhou, L. Hu, J. Chen, J. Zhu, and A. Shan. 2020. Leucine and isoleucine have similar effects on reducing lipid accumulation, improving insulin sensitivity and increasing the browning of WAT in high-fat diet-induced obese mice. Food & Function 11 (3):2279–90. doi: 10.1039/c9fo03084k.
- Macotela, Y., B. Emanuelli, A. M. Bång, D. O. Espinoza, J. Boucher, K. Beebe, W. Gall, and C. R. Kahn. 2011. Dietary leucine-an environmental modifier of insulin resistance acting on multiple levels of metabolism. PLoS One 6 (6):e21187. doi: 10.1371/journal.pone.0021187.
- Mahendran, Y., A. Jonsson, C. T. Have, K. H. Allin, D. R. Witte, M. E. Jørgensen, N. Grarup, O. Pedersen, T. O. Kilpeläinen, and T. Hansen. 2017. Genetic evidence of a causal effect of insulin resistance on branched-chain amino acid levels. Diabetologia 60 (5):873–8. doi: 10.1007/s00125-017-4222-6.
- Maida, A., J. S. K. Chan, K. A. Sjøberg, A. Zota, D. Schmoll, B. Kiens, S. Herzig, and A. J. Rose. 2017. Repletion of branched chain amino acids reverses MTORC1 signaling but not improved metabolism during dietary protein dilution. Molecular Metabolism 6 (8):873–81. doi: 10.1016/j.molmet.2017.06.009.
- Maida, A., A. Zota, K. A. Sjøberg, J. Schumacher, T. P. Sijmonsma, A. Pfenninger, M. M. Christensen, T. Gantert, J. Fuhrmeister, U. Rothermel, et al. 2016. A liver stress-endocrine nexus promotes metabolic integrity during dietary protein dilution. The Journal of Clinical Investigation 126 (9):3263–78. doi: 10.1172/JCI85946.
- Mangge, H., S. Zelzer, F. Prüller, W. J. Schnedl, D. Weghuber, D. Enko, P. Bergsten, J. Haybaeck, and A. Meinitzer. 2016. Branched-chain amino acids are associated with cardiometabolic risk profiles found already in lean, overweight and obese young. The Journal of Nutritional Biochemistry 32 (June):123–7. doi: 10.1016/j.jnutbio.2016.02.007.
- Marafie, S. K., E. M. Al-Shawaf, J. Abubaker, and H. Arefanian. 2019. Palmitic acid-induced lipotoxicity promotes a novel interplay between Akt-MTOR, IRS-1, and FFAR1 signaling in pancreatic β-cells. Biological Research 52 (1):44. doi: 10.1186/s40659-019-0253-4.
- Mardinoglu, A., S. Gogg, L. A. Lotta, A. Stančáková, A. Nerstedt, J. Boren, M. Blüher, E. Ferrannini, C. Langenberg, N. J. Wareham, et al. 2018. Elevated plasma levels of 3-hydroxyisobutyric acid are associated with incident type 2 diabetes. EBioMedicine 27 (January):151–5. doi: 10.1016/j.ebiom.2017.12.008.
- Martin, N. R. W., M. C. Turner, R. Farrington, D. J. Player, and M. P. Lewis. 2017. Leucine elicits myotube hypertrophy and enhances maximal contractile force in tissue engineered skeletal muscle in vitro. Journal of Cellular Physiology 232 (10):2788–97. doi: 10.1002/JCP.25960.
- Master, P. B. Z., and R. C. O. Macedo. 2021. Effects of dietary supplementation in sport and exercise: A review of evidence on milk proteins and amino acids. Critical Reviews in Food Science and Nutrition 61 (7):1225–39. doi: 10.1080/10408398.2020.1756216.
- Mayers, J. R., M. E. Torrence, L. V. Danai, T. Papagiannakopoulos, S. M. Davidson, M. R. Bauer, A. N. Lau, B. W. Ji, P. D. Dixit, A. M. Hosios, et al. 2016. Tissue of origin dictates branched-chain amino acid metabolism in mutant kras-driven cancers. Science 353 (6304):1161–5. doi: 10.1126/science.aaf5171.
- McGarrah, R. W., Zhang, G.-F. B. A. Christopher, Y. Deleye, J. M. Walejko, S. Page, O. Ilkayeva, P. J. White, and Christopher, and B. Newgard. 2020. Dietary branched-chain amino acid restriction alters fuel selection and reduces triglyceride stores in hearts of zucker fatty rats. American Journal of Physiology. Endocrinology and Metabolism 318 (2):E216–E223. doi: 10.1152/ajpendo.00334.2019.
- Miyake, T., M. Abe, S. Furukawa, Y. Tokumoto, K. Toshimitsu, T. Ueda, S. Yamamoto, M. Hirooka, T. Kumagi, Y. Hiasa, et al. 2012. Long-term branched-chain amino acid supplementation improves glucose tolerance in patients with nonalcoholic steatohepatitis-related cirrhosis. Internal Medicine 51 (16):2151–5. doi: 10.2169/internalmedicine.51.7578.
- Mizusawa, A., A. Watanabe, M. Yamada, R. Kamei, Y. Shimomura, and Y. Kitaura. 2020. BDK deficiency in cerebral cortex neurons causes neurological abnormalities and affects endurance capacity. Nutrients 12 (8):1–10. doi: 10.3390/nu12082267.
- Moberg, M., W. Apró, B. Ekblom, G. Van Hall, H. Christer Holmberg, and E. Blomstrand. 2016. Activation of MTORC1 by leucine is potentiated by branched-chain amino acids and even more so by essential amino acids following resistance exercise. American Journal of Physiology-Cell Physiology 310 (11):C874–C884. doi: 10.1152/ajpcell.00374.2015.
- Moghei, M., P. Tavajohi-Fini, B. Beatty, and O. A. J. Adegoke. 2016. Ketoisocaproic acid, a metabolite of leucine, suppresses insulin-stimulated glucose transport in skeletal muscle cells in a BCAT2-dependent manner. American Journal of Physiology-Cell Physiology 311 (3):C518–C527. doi: 10.1152/ajpcell.00062.2016.
- Molfino, A., M. I. Amabile, T. Ammann, S. Lai, A. Grosso, L. Lionetto, A. Spagnoli, M. Simmaco, M. Monti, A. Laviano, et al. 2019. Longitudinal physical activity change during hemodialysis and its association with body composition and plasma BAIBA levels. Frontiers in Physiology 10:805. doi: 10.3389/fphys.2019.00805.
- Mu, W.-C., E. VanHoosier, C. M. Elks, and R. W. Grant. 2018. Long-term effects of dietary protein and branched-chain amino acids on metabolism and inflammation in mice. Nutrients 10 (7):918. doi: 10.3390/nu10070918.
- Nagao, K., and M. Yamakado. 2016. The role of amino acid profiles in diabetes risk assessment. Current Opinion in Clinical Nutrition and Metabolic Care 19 (5):328–35. doi: 10.1097/MCO.0000000000000305.
- Nakanishi, K., T. Namisaki, T. Mashitani, K. Kaji, K. Ozaki, S. Saikawa, S. Sato, T. Inoue, Y. Sawada, K. Kitagawa, et al. 2019. Late-evening snack with branched-chain amino acid-enriched nutrients does not always inhibit overt diabetes in patients with cirrhosis: A pilot study. Nutrients 11 (9):2140. doi: 10.3390/nu11092140.
- Napolitano, L., M. Scalise, M. Koyioni, P. Koutentis, M. Catto, I. Eberini, C. Parravicini, L. Palazzolo, L. Pisani, M. Galluccio, et al. 2017. Potent inhibitors of human LAT1 (SLC7A5) transporter based on dithiazole and dithiazine compounds for development of anticancer drugs. Biochemical Pharmacology 143:39–52. doi: 10.1016/j.bcp.2017.07.006.
- Neinast, M., D. Murashige, and Z. Arany. 2019. Branched chain amino acids. Annual Review of Physiology 81:139–64. doi: 10.1146/annurev-physiol-020518-114455.
- Neinast, M. D., C. Jang, S. Hui, D. S. Murashige, Q. Chu, R. J. Morscher, X. Li, L. Zhan, E. White, T. G. Anthony, et al. 2019. Quantitative analysis of the whole-body metabolic fate of branched-chain amino acids. Cell Metabolism 29 (2):417–29.e4. doi: 10.1016/j.cmet.2018.10.013.
- Neishabouri, S., Hallaj, S. M. Hutson, and J. Davoodi. 2015. Chronic activation of MTOR complex 1 by branched chain amino acids and organ hypertrophy. Amino Acids 47 (6):1167–82. doi: 10.1007/s00726-015-1944-y.
- Newgard, C. B., J. An, J. R. Bain, M. J. Muehlbauer, R. D. Stevens, L. F. Lien, A. M. Haqq, S. H. Shah, M. Arlotto, C. A. Slentz, et al. 2009. A branched-chain amino acid-related metabolic signature that differentiates obese and lean humans and contributes to insulin resistance. Cell Metabolism 9 (4):311–26. doi: 10.1016/j.cmet.2009.02.002.
- Nie, C., He, T. W. Zhang, and G. Zhang, Xi Ma. 2018. Branched chain amino acids: Beyond nutrition metabolism. International Journal of Molecular Sciences 19 (4):954. doi: 10.3390/ijms19040954.
- Nilsen, M. S., R. Å. Jersin, A. Ulvik, A. Madsen, A. McCann, P.-A. Svensson, M. K. Svensson, B. G. Nedrebø, O. A. Gudbrandsen, G. S. Tell, et al. 2020. 3-hydroxyisobutyrate, a strong marker of insulin resistance in type 2 diabetes and obesity that modulates white and brown adipocyte metabolism. Diabetes 69 (9):1903–16. doi: 10.2337/db19-1174.
- Novarino, G., P. El-Fishawy, H. Kayserili, N. A. Meguid, E. M. Scott, J. Schroth, J. L. Silhavy, M. Kara, R. O. Khalil, T. Ben-Omran, et al. 2012. Mutations in BCKD-kinase lead to a potentially treatable form of autism with epilepsy. Science 338 (6105):394–7. doi: 10.1126/science.1224631.
- Oishi, K., and G. A. Diaz. 2014. Glycerol phenylbutyrate for the chronic management of urea cycle disorders. Expert Review of Endocrinology & Metabolism 9 (5):427–34. doi: 10.1586/17446651.2014.945908.
- Ooi, D. S. Q., J. Q. R. Ling, S. A. Sadananthan, S. S. Velan, F. Y. Ong, C. M. Khoo, E. S. Tai, C. J. Henry, M. K. S. Leow, E. Y. H. Khoo, et al. 2021. Branched-chain amino acid supplementation does not preserve lean mass or affect metabolic profile in adults with overweight or obesity in a randomized controlled weight loss intervention. The Journal of Nutrition 151 (4):911–20. doi: 10.1093/jn/nxaa414.
- Overmyer, K. A., C. R. Evans, N. R. Qi, C. E. Minogue, J. J. Carson, C. J. Chermside-Scabbo, L. G. Koch, S. L. Britton, D. J. Pagliarini, J. J. Coon, et al. 2015. Maximal oxidative capacity during exercise is associated with skeletal muscle fuel selection and dynamic changes in mitochondrial protein acetylation. Cell Metabolism 21 (3):468–78. doi: 10.1016/j.cmet.2015.02.007.
- Oyarzabal, A., M. Martínez-Pardo, B. Merinero, R. Navarrete, L. R. Desviat, M. Ugarte, and P. Rodríguez-Pombo. 2013. A novel regulatory defect in the branched-chain α-keto acid dehydrogenase complex due to a mutation in the PPM1K gene causes a mild variant phenotype of maple syrup urine disease. Human Mutation 34 (2):355–62. doi: 10.1002/humu.22242.
- Pajvani, U. B., X. Du, T. P. Combs, A. H. Berg, M. W. Rajala, T. Schulthess, J. Engel, M. Brownlee, and P. E. Scherer. 2003. Structure-function studies of the adipocyte-secreted hormone Acrp30/adiponectin. Implications fpr metabolic regulation and bioactivity. The Journal of Biological Chemistry 278 (11):9073–85. doi: 10.1074/jbc.M207198200.
- Pakiet, A., M. Wilczynski, O. Rostkowska, J. Korczynska, P. Jabłonska, L. Kaska, M. Proczko-Stepaniak, E. Sobczak, P. Stepnowski, F. Magkos, et al. 2020. The effect of one anastomosis gastric bypass on branched-chain fatty acid and branched-chain amino acid metabolism in subjects with morbid obesity. Obesity Surgery 30 (1):304–12. doi: 10.1007/s11695-019-04157-z.
- Pedersen, H. K., V. Gudmundsdottir, H. B. Nielsen, T. Hyotylainen, T. Nielsen, B. A. H. Jensen, K. Forslund, F. Hildebrand, E. Prifti, G. Falony, et al. 2016. Human gut microbes impact host serum metabolome and insulin sensitivity. Nature 535 (7612):376–81. doi: 10.1038/nature18646.
- Peyrollier, K., E. Hajduch, A. S. Blair, R. Hyde, and H. S. Hundal. 2000. L-leucine availability regulates phosphatidylinositol 3-kinase, P70 S6 kinase and glycogen synthase kinase-3 activity in L6 muscle cells: Evidence for the involvement of the mammalian target of rapamycin (MTOR) pathway in the L-leucine-induced up-regulation of System A amino acid transport. Biochemical Journal 350 (2):361–8. doi: 10.1042/0264-6021:3500361.
- Piccolo, B. D., K. B. Comerford, S. E. Karakas, T. A. Knotts, O. Fiehn, and S. H. Adams. 2015. Whey protein supplementation does not alter plasma branched-chained amino acid profiles but results in unique metabolomics patterns in obese women enrolled in an 8-week weight loss trial. The Journal of Nutrition 145 (4):691–700. doi: 10.3945/jn.114.203943.
- Polis, B., and A. O. Samson. 2020. Role of the metabolism of branched-chain amino acids in the development of Alzheimer’s disease and other metabolic disorders. Neural Regeneration Research 15 (8):1460–70. doi: 10.4103/1673-5374.274328.
- Prosdocimo, D. A., P. Anand, X. Liao, H. Zhu, S. Shelkay, P. Artero-Calderon, L. Zhang, J. Kirsh, D. Moore, M. G. Rosca, et al. 2014. Kruppel-like factor 15 is a critical regulator of cardiac lipid metabolism. The Journal of Biological Chemistry 289 (9):5914–24. doi: 10.1074/jbc.M113.531384.
- Purpera, M. N., L. Shen, M. Taghavi, H. Münzberg, R. J. Martin, S. M. Hutson, and C. D. Morrison. 2012. Impaired branched chain amino acid metabolism alters feeding behavior and increases orexigenic neuropeptide expression in the hypothalamus. Journal of Endocrinology 212 (1):85–94. doi: 10.1530/JOE-11-0270.
- Qin, L.‐Q., P. Xun, D. Bujnowski, M. L. Daviglus, L. Van Horn, J. Stamler, and K. He. 2011. Higher branched-chain amino acid intake is associated with a lower prevalence of being overweight or obese in middle-aged East Asian and Western adults. The Journal of Nutrition 141 (2):249–54. doi: 10.3945/jn.110.128520.
- Rabaglia, M. E., M. P. Gray-Keller, B. L. Frey, M. R. Shortreed, L. M. Smith, and A. D. Attie. 2005. Alpha-ketoisocaproate-induced hypersecretion of insulin by islets from diabetes-susceptible mice. American Journal of Physiology. Endocrinology and Metabolism 289 (2):E218–E224. doi: 10.1152/ajpendo.00573.2004.
- Ramzan, I., M. Taylor, B. Phillips, D. Wilkinson, K. Smith, I. Idris, P. Atherton, and K. Hession. 2020. A novel dietary intervention reduces circulatory branched-chain amino acids by 50%: A pilot study of relevance for obesity and diabetes. Nutrients 13 (1):95. doi: 10.3390/nu13010095.
- Rawat, A. K., V. Korthikunta, S. Gautam, S. Pal, N. Tadigoppula, A. K. Tamrakar, and A. K. Srivastava. 2014. 4-hydroxyisoleucine improves insulin resistance by promoting mitochondrial biogenesis and act through AMPK and Akt dependent pathway. Fitoterapia 99 (December):307–17. doi: 10.1016/j.fitote.2014.10.006.
- Ricoult, S. J. H., and B. D. Manning. 2013. The multifaceted role of MTORC1 in the control of lipid metabolism. EMBO Reports 14 (3):242–51. doi: 10.1038/embor.2013.5.
- Ridaura, V. K., J. J. Faith, F. E. Rey, J. Cheng, A. E. Duncan, A. L. Kau, N. W. Griffin, V. Lombard, B. Henrissat, J. R. Bain, et al. 2013. Gut microbiota from twins discordant for obesity modulate metabolism in mice. Science 341 (6150):1241214. doi:10.1126/science.
- Rivera, M. E., E. S. Lyon, M. A. Johnson, and R. A. Vaughan. 2020. Leucine increases mitochondrial metabolism and lipid content without altering insulin signaling in myotubes. Biochimie 168 (January):124–33. doi: 10.1016/j.biochi.2019.10.017.
- Roberts, L. D., P. Boström, J. F. O’Sullivan, R. T. Schinzel, G. D. Lewis, A. Dejam, Y.-K. Lee, M. J. Palma, S. Calhoun, A. Georgiadi, et al. 2014. β-aminoisobutyric acid induces browning of white fat and hepatic β-oxidation and is inversely correlated with cardiometabolic risk factors. Cell Metabolism 19 (1):96–108. doi: 10.1016/j.cmet.2013.12.003.
- Roquetto, A. R., C. S. Moura, V. de Almeida Santos-Junior, P. O. S. Oliveira, K. I. A. Machado, G. C. B. C. Carvalho, E. M. Risso, and J. Amaya-Farfan. 2020. Moderate intake of BCAA-rich protein improves glucose homeostasis in high-fat-fed mice. The Journal of Nutritional Biochemistry 80 (June):108332. doi: 10.1016/j.jnutbio.2019.108332.
- Ruderman, N. B., X. J. Xu, L. Nelson, J. M. Cacicedo, A. K. Saha, F. Lan, and Y. Ido. 2010. AMPK and SIRT1: A long-standing partnership?American Journal of Physiology 298 (4):E751–E760. doi: 10.1152/ajpendo.00745.2009.
- Ruskovska, T., and D. A. Bernlohr. 2013. Oxidative stress and protein carbonylation in adipose tissue—Implications for insulin resistance and diabetes mellitus. Journal of Proteomics 92 (October):323–34. doi: 10.1016/j.jprot.2013.04.002.
- Saha, A., K. Coughlan, R. Valentine, and N. Ruderman. 2014. AMPK activation: A therapeutic target for type 2 diabetes?Diabetes, Metabolic Syndrome and Obesity 7:241–53. doi: 10.2147/DMSO.S43731.
- Sánchez-González, C., C. Nuevo-Tapioles, J. C. Herrero Martín, M. P. Pereira, S. Serrano Sanz, A. Ramírez de Molina, J. M. Cuezva, and L. Formentini. 2020. Dysfunctional oxidative phosphorylation shunts branched-chain amino acid catabolism onto lipogenesis in skeletal muscle. The EMBO Journal 39 (14):e103812. doi: 10.15252/embj.2019103812.
- Sans, R. M., W. W. Jolly, and R. A. Harris. 1980. Studies on the regulation of leucine catabolism. Mechanism Responsible for oxidizable substrate inhibition and dichloroacetate stimulation of leucine oxidation by the heart. Archives of Biochemistry and Biophysics 200 (2):336–45. doi: 10.1016/0003-9861(80)90363-X.
- Sato, Y., H. Tate, F. Yoshizawa, and Y. Sato. 2020. Data on the proliferation and differentiation of C2C12 myoblast treated with branched-chain ketoacid dehydrogenase kinase inhibitor. Data Brief 31 (August):105766. 105766. doi: 10.1016/j.dib.2020.105766.
- Satomi, S., A. Morio, H. Miyoshi, R. Nakamura, R. Tsutsumi, H. Sakaue, T. Yasuda, N. Saeki, and Y. M. Tsutsumi. 2020. Branched-chain amino acids-induced cardiac protection against ischemia/reperfusion injury. Life Sciences 245 (March):117368. doi: 10.1016/j.lfs.2020.117368.
- Saxton, R. A., and D. M. Sabatini. 2017. MTOR signaling in growth, metabolism, and disease. Cell 168 (6):960–76. doi: 10.1016/j.cell.2017.02.004.
- Scalise, M., M. Galluccio, L. Console, L. Pochini, and C. Indiveri. 2018. The human SLC7A5 (LAT1): The intriguing histidine/large neutral amino acid transporter and its relevance to human health. Frontiers in Chemistry 6 (JUN):243. doi: 10.3389/fchem.2018.00243.
- Scott, L. J. 2017. Sitagliptin: A review in type 2 diabetes. Drugs 77 (2):209–24. doi: 10.1007/s40265-016-0686-9.
- Sears, D. D., G. Hsiao, A. Hsiao, J. G. Yu, C. H. Courtney, J. M. Ofrecio, J. Chapman, and S. Subramaniam. 2009. Mechanisms of human insulin resistance and thiazolidinedione-mediated insulin sensitization. Proceedings of the National Academy of Sciences of the United States of America 106 (44):18745–50. doi: 10.1073/pnas.0903032106.
- Serralde-Zúñiga, A. E., M. Guevara-Cruz, A. R. Tovar, M. F. Herrera-Hernández, L. G. Noriega, O. Granados, and N. Torres. 2014. Omental adipose tissue gene expression, gene variants, branched-chain amino acids, and their relationship with metabolic syndrome and insulin resistance in humans. Genes & Nutrition 9 (6):1–10. doi: 10.1007/s12263-014-0431-5.
- Seyfried, F., J. Phetcharaburanin, M. Glymenaki, A. Nordbeck, M. Hankir, J. K. Nicholson, E. Holmes, J. R. Marchesi, and J. V. Li. 2021. Roux-En-Y gastric bypass surgery in zucker rats induces bacterial and systemic metabolic changes independent of caloric restriction-induced weight loss. Gut Microbes 13 (1):1–20. doi: 10.1080/19490976.2021.1875108.
- Shan, Y., Y. Gao, W. Jin, M. Fan, Y. Wang, Y. Gu, C. Shan, L. Sun, X. Li, B. Yu, et al. 2019. Targeting HIBCH to reprogram valine metabolism for the treatment of colorectal cancer. Cell Death & Disease 10 (8):618. doi: 10.1038/s41419-019-1832-6.
- Shao, D., O. Villet, Z. Zhang, S. W. Choi, J. Yan, J. Ritterhoff, H. Gu, D. Djukovic, D. Christodoulou, S. C. Kolwicz, et al. 2018. Glucose promotes cell growth by suppressing branched-chain amino acid degradation. Nature Communications 9 (1):2935. doi: 10.1038/s41467-018-05362-7.
- She, P., K. C. Olson, Y. Kadota, A. Inukai, Y. Shimomura, C. L. Hoppel, S. H. Adams, Y. Kawamata, H. Matsumoto, R. Sakai, et al. 2013. Leucine and protein metabolism in obese zucker rats. PLoS One 8 (3):e59443. doi: 10.1371/journal.pone.0059443.
- She, P., T. M. Reid, S. K. Bronson, T. C. Vary, A. Hajnal, C. J. Lynch, and S. M. Hutson. 2007. Disruption of BCATm in mice leads to increased energy expenditure associated with the activation of a futile protein turnover cycle. Cell Metabolism 6 (3):181–94. doi: 10.1016/j.cmet.2007.08.003.
- She, P., C. Van Horn, T. Reid, S. M. Hutson, R. N. Cooney, and C. J. Lynch. 2007. Obesity-related elevations in plasma leucine are associated with alterations in enzymes involved in branched-chain amino acid metabolism. American Journal of Physiology. Endocrinology and Metabolism 293 (6):E1552–E1563. doi: 10.1152/ajpendo.00134.2007.
- She, P., Y. Zhou, Z. Zhang, K. Griffin, K. Gowda, and C. J. Lynch. 2010. Disruption of BCAA metabolism in mice impairs exercise metabolism and endurance. Journal of Applied Physiology 108 (4):941–9. doi: 10.1152/japplphysiol.01248.2009.
- Shi, C.-X., M.-X. Zhao, X.-D. Shu, X.-Q. Xiong, J.-J. Wang, X.-Y. Gao, Q. Chen, Y.-H. Li, Y.-M. Kang, and G.-Q. Zhu. 2016. β-aminoisobutyric acid attenuates hepatic endoplasmic reticulum stress and glucose/lipid metabolic disturbance in mice with type 2 diabetes. Scientific Reports 6:21924. doi: 10.1038/srep21924.
- Shin, A. C., M. Fasshauer, N. Filatova, L. A. Grundell, E. Zielinski, J.-Y. Zhou, T. Scherer, C. Lindtner, P. J. White, A. L. Lapworth, et al. 2014. Brain insulin lowers circulating BCAA levels by inducing hepatic BCAA catabolism. Cell Metabolism 20 (5):898–909. doi: 10.1016/j.cmet.2014.09.003.
- Shou, J., P.-J. Chen, and W.-H. Xiao. 2019. The effects of BCAAs on insulin resistance in athletes. Journal of Nutritional Science and Vitaminology 65 (5):383–9. doi: 10.3177/jnsv.65.383.
- Siddik, M. A. B., and A. C. Shin. 2019. Recent progress on branched-chain amino acids in obesity, diabetes, and beyond. Endocrinology and Metabolism 34 (3):234–46. doi: 10.3803/EnM.2019
- Simpson, S. J., and D. Raubenheimer. 2005. Obesity: The protein leverage hypothesis. Obesity Reviews 6 (2):133–42. doi: 10.1111/j.1467-789X.2005.00178.x.
- Sohn, J.-W. 2015. Network of hypothalamic neurons that control appetite. BMB Reports 48 (4):229–33. doi: 10.5483/BMBRep.2015.48.4.272.
- Solerte, S. B., M. Fioravanti, E. Locatelli, R. Bonacasa, M. Zamboni, C. Basso, A. Mazzoleni, V. Mansi, N. Geroutis, and C. Gazzaruso. 2008. Improvement of blood glucose control and insulin sensitivity during a long-term (60 weeks) randomized study with amino acid dietary supplements in elderly subjects with type 2 diabetes mellitus. The American Journal of Cardiology 101 (11A):82E–88E. doi: 10.1016/j.amjcard.2008.03.006.
- Solon-Biet, S. M., V. C. Cogger, T. Pulpitel, D. Wahl, X. Clark, E. Bagley, G. C. Gregoriou, A. M. Senior, Q.-P. Wang, A. E. Brandon, et al. 2019. Branched chain amino acids impact health and lifespan indirectly via amino acid balance and appetite control. Nature Metabolism 1 (5):532–45. doi: 10.1038/s42255-019-0059-2.
- Stautemas, J., A. B. P. Van Kuilenburg, L. Stroomer, F. Vaz, L. Blancquaert, F. B. D. Lefevere, I. Everaert, and W. Derave. 2019. Acute aerobic exercise leads to increased plasma levels of R- and S-β-aminoisobutyric acid in humans. Frontiers in Physiology 10 (SEP):1240. doi: 10.3389/fphys.2019.01240.
- Streck, E. L., F. P. Bussular, L. B. Wessler, M. B. Duarte, V. L. Rezende, M. S. Rodrigues, C. A. Torres, I. S. Lemos, G. Candiotto, F. F. Gava, et al. 2021. Administration of branched-chain amino acids alters epigenetic regulatory enzymes in an animal model of maple syrup urine disease. Metabolic Brain Disease 36 (2):247–54. doi: 10.1007/s11011-020-00631-1.
- Su, X., F. Magkos, D. Zhou, J. Christopher Eagon, E. Fabbrini, A. L. Okunade, and S. Klein. 2015. Adipose tissue monomethyl branched-chain fatty acids and insulin sensitivity: Effects of obesity and weight loss. Obesity 23 (2):329–34. doi: 10.1002/oby.20923.
- Sugawara, T., Y. Ito, N. Nishizawa, and T. Nagasawa. 2009. Regulation of muscle protein degradation, not synthesis, by dietary leucine in rats fed a protein-deficient diet. Amino Acids 37 (4):609–16. doi: 10.1007/s00726-008-0180-0.
- Sun, H., K. C. Olson, C. Gao, D. A. Prosdocimo, M. Zhou, Z. Wang, D. Jeyaraj, J.-Y. Youn, S. Ren, Y. Liu, et al. 2016. Catabolic defect of branched-chain amino acids promotes heart failure. Circulation 133 (21):2038–49. doi: 10.1161/CIRCULATIONAHA.115.020226.
- Sunny, N. E., S. Kalavalapalli, F. Bril, T. J. Garrett, M. Nautiyal, J. T. Mathew, C. M. Williams, and K. Cusi. 2015. Cross-talk between branched-chain amino acids and hepatic mitochondria is compromised in nonalcoholic fatty liver disease. American Journal of Physiology. Endocrinology and Metabolism 309 (4):E311–E319. doi: 10.1152/ajpendo.00161.2015.
- Supruniuk, E., A. Mikłosz, and A. Chabowski. 2020. Pyrroloquinoline quinone modifies lipid profile, but not insulin sensitivity, of palmitic acid-treated L6 myotubes. International Journal of Molecular Sciences 21 (21):8382. doi: 10.3390/ijms21218382.
- Szkudelska, K., L. Nogowski, and T. Szkudelski. 2014. Adipocyte dysfunction in rats with streptozotocin-nicotinamide-induced diabetes. International Journal of Experimental Pathology 95 (2):86–94. doi: 10.1111/iep.12073.
- Taghavi, M., and S. Hutson. 2012. Generation of branched chain Α‐keto acid dehydrogenase enzyme complex E1α knockout mouse models. The FASEB Journal 26 (S1):1013.24. doi: 10.1096/fasebj.26.1_supplement.1013.24.
- Tamanna, N., and N. Mahmood. 2014. Emerging roles of branched-chain amino acid supplementation in human diseases. International Scholarly Research Notices 2014 (November):235619. doi: 10.1155/2014/235619.
- Tan, H. C., J. W. Hsu, C. M. Khoo, E. Shyong Tai, S. Yu, S. Chacko, O. F. Lai, and F. Jahoor. 2018. Alterations in branched-chain amino acid kinetics in nonobese but insulin-resistant Asian men. The American Journal of Clinical Nutrition 108 (6):1220–8. doi: 10.1093/ajcn/nqy208.
- Tan, H. C., J. W. Hsu, J.-P. Kovalik, A. Eng, W. H. Chan, C. M. Khoo, E. S. Tai, S. Chacko, and F. Jahoor. 2020. Branched-chain amino acid oxidation is elevated in adults with morbid obesity and decreases significantly after sleeve gastrectomy. The Journal of Nutrition 150 (12):3180–9. doi: 10.1093/jn/nxaa298.
- Taneera, J., S. Lang, A. Sharma, J. Fadista, Y. Zhou, E. Ahlqvist, A. Jonsson, V. Lyssenko, P. Vikman, O. Hansson, et al. 2012. A systems genetics approach identifies genes and pathways for type 2 diabetes in human islets. Cell Metabolism 16 (1):122–34. doi: 10.1016/j.cmet.2012.06.006.
- Terakura, D., M. Shimizu, J. Iwasa, A. Baba, T. Kochi, T. Ohno, M. Kubota, Y. Shirakami, M. Shiraki, K. Takai, et al. 2012. Preventive effects of branched-chain amino acid supplementation on the spontaneous development of hepatic preneoplastic lesions in C57BL/KsJ-Db/Db obese mice. Carcinogenesis 33 (12):2499–506. doi: 10.1093/carcin/bgs303.
- Tobias, D. K., A. Hazra, P. R. Lawler, P. D. Chandler, D. I. Chasman, J. E. Buring, I. Min Lee, S. Cheng, A. E. Jo, S. Manson, et al. 2020. Circulating branched-chain amino acids and long-term risk of obesity-related cancers in women. Scientific Reports 10 (1):16534. doi: 10.1038/s41598-020-73499-x.
- Tobias, D. K., S. Mora, S. Verma, F. Billia, J. E. Buring, and P. R. Lawler. 2021. Fasting status and metabolic health in relation to plasma branched chain amino acid concentrations in women. Metabolism 117 (April):154391. doi: 10.1016/j.metabol.2020.154391.
- Torres-Leal, F. L., M. H. Fonseca-Alaniz, G. F. Teodoro, M. D. De Capitani, D. Vianna, L. C. Pantaleão, E. M. Matos-Neto, M. M. Rogero, J. Donato, and J. Tirapegui. 2011. Leucine supplementation improves adiponectin and total cholesterol concentrations despite the lack of changes in adiposity or glucose homeostasis in rats previously exposed to a high-fat diet. Nutrition & Metabolism 8 (1):62. doi: 10.1186/1743-7075-8-62.
- Townsend, J. R., M. S. Fragala, A. R. Jajtner, A. M. Gonzalez, A. J. Wells, G. T. Mangine, E. H. Robinson, W. P. McCormack, K. S. Beyer, G. J. Pruna, et al. 2013. β-hydroxy-β-methylbutyrate (HMB)-free acid attenuates circulating TNF-α and TNFR1 expression postresistance exercise. Journal of Applied Physiology 115 (8):1173–82. doi: 10.1152/japplphysiol.00738.2013.
- Tso, S.-C., W.-J. Gui, C.-Y. Wu, J. L. Chuang, X. Qi, K. J. Skvora, K. Dork, A. L. Wallace, L. K. Morlock, B. H. Lee, et al. 2014. Benzothiophene carboxylate derivatives as novel allosteric inhibitors of branched-chain α-ketoacid dehydrogenase kinase. The Journal of Biological Chemistry 289 (30):20583–93. doi: 10.1074/jbc.M114.569251.
- Uddin, G. M., S. Pherwani, C. S. Wagg, K. Gopal, R. A. Batran, L. Zhang, Y. Wu, N. Hussaini, S. Rawat, J. R. Ussher, et al. 2019. A cardiac specific branched chain aminotransferase deletion increases insulin stimulated glucose oxidation in the mouse heart. Circulation Research 125 (Suppl_1):868. doi: 10.1161/res.125.suppl_1.868.
- Uddin, G. M., L. Zhang, S. Shah, A. Fukushima, C. S. Wagg, K. Gopal, R. Al Batran, S. Pherwani, K. L. Ho, J. Boisvenue, et al. 2019. Impaired branched chain amino acid oxidation contributes to cardiac insulin resistance in heart failure. Cardiovascular Diabetology 18 (1):86. doi: 10.1186/s12933-019-0892-3.
- Valerio, A., G. D’Antona, and E. Nisoli. 2011. Branched-chain amino acids, mitochondrial biogenesis, and healthspan: An evolutionary perspective. Aging 3 (5):464–78. doi: 10.18632/aging.100322.
- Vangipurapu, J., A. Stancáková, U. Smith, J. Kuusisto, and M. Laakso. 2019. Nine amino acids are associated with decreased insulin secretion and elevated glucose levels in a 7.4-year follow-up study of 5,181 Finnish men. Diabetes 68 (6):1353–8. doi: 10.2337/db18-1076.
- Vanweert, F., S. C. Boone, B. Brouwers, D. O. Mook-Kanamori, R. de Mutsert, F. R. Rosendaal, H. J. Lamb, V. B. Schrauwen-Hinderling, P. Schrauwen, M. K. C. Hesselink, et al. 2021. The effect of physical activity level and exercise training on the association between plasma branched-chain amino acids and intrahepatic lipid content in participants with obesity. International Journal of Obesity 45 (7):1510–20. doi: 10.1038/s41366-021-00815-4.
- Wagenmakers, A. J. M. 1998. Muscle amino acid metabolism at rest and during exercise: Role in human physiology and metabolism. Exercise and Sport Sciences Reviews 26:287–314. https://pubmed.ncbi.nlm.nih.gov/9696993/. doi: 10.1249/00003677-199800260-00013.
- Walejko, J. M., B. A. Christopher, S. B. Crown, G.-F. Zhang, A. Pickar-Oliver, T. Yoneshiro, M. W. Foster, S. Page, S. van Vliet, O. Ilkayeva, et al. 2021. Branched-chain α-ketoacids are preferentially reaminated and activate protein synthesis in the heart. Nature Communications 12 (1):1680–14. doi: 10.1038/s41467-021-21962-2.
- Walford, G. A., J. Davis, A. S. Warner, R. J. Ackerman, L. K. Billings, B. Chamarthi, R. R. Fanelli, A. M. Hernandez, C. Huang, S. Q. Khan, et al. 2013. Branched chain and aromatic amino acids change acutely following two medical therapies for type 2 diabetes mellitus. Metabolism 62 (12):1772–8. doi: 10.1016/j.metabol.2013.07.003.
- Walker, D. K., M. J. Drummond, J. M. Dickinson, M. S. Borack, K. Jennings, E. Volpi, and B. B. Rasmussen. 2014. Insulin increases MRNA abundance of the amino acid transporter SLC7A5/LAT1 via an MTORC1-dependent mechanism in skeletal muscle cells. Physiological Reports 2 (3):e00238. doi: 10.1002/phy2.238.
- Wallace, M., C. R. Green, L. S. Roberts, Y. M. Lee, J. L. McCarville, J. Sanchez-Gurmaches, N. Meurs, J. M. Gengatharan, J. D. Hover, S. A. Phillips, et al. 2018. Enzyme promiscuity drives branched-chain fatty acid synthesis in adipose tissues. Nature Chemical Biology 14 (11):1021–31. doi: 10.1038/s41589-018-0132-2.
- Wanders, D., K. P. Stone, K. Dille, J. Simon, A. Pierse, and T. W. Gettys. 2015. Metabolic responses to dietary leucine restriction involve remodeling of adipose tissue and enhanced hepatic insulin signaling. BioFactors 41 (6):391–402. doi: 10.1002/biof.1240.
- Wang, J., Y. Liu, K. Lian, X. Shentu, J. Fang, J. Shao, M. Chen, Y. Wang, M. Zhou, and H. Sun. 2019. BCAA catabolic defect alters glucose metabolism in lean mice. Frontiers in Physiology 10 (September):1140. doi: 10.3389/fphys.2019.01140.
- Wang, T. J., M. G. Larson, R. S. Vasan, S. Cheng, E. P. Rhee, E. McCabe, G. D. Lewis, C. S. Fox, P. F. Jacques, C. Fernandez, et al. 2011. Metabolite profiles and the risk of developing diabetes. Nature Medicine 17 (4):448–53. doi: 10.1038/nm.2307.
- Watterson, K. R., D. Bestow, J. Gallagher, D. L. Hamilton, F. B. Ashford, P. J. Meakin, and M. L. J. Ashford. 2013. Anorexigenic and orexigenic hormone modulation of mammalian target of rapamycin complex 1 activity and the regulation of hypothalamic agouti-related protein MRNA expression. Neuro-Signals 21 (1-2):28–41. doi: 10.1159/000334144.
- Wei, S., J. Zhao, S. Wang, M. Huang, Y. Wang, and Y. Chen. 2018. Intermittent administration of a leucine-deprived diet is able to intervene in type 2 diabetes in Db/Db mice. Heliyon 4 (9):e00830. doi: 10.1016/j.heliyon.2018.e00830.
- Weickert, M. O., M. Roden, F. Isken, D. Hoffmann, P. Nowotny, M. Osterhoff, M. Blaut, C. Alpert, O. Gögebakan, C. Bumke-Vogt, et al. 2011. Effects of supplemented isoenergetic diets differing in cereal fiber and protein content on insulin sensitivity in overweight humans. The American Journal of Clinical Nutrition 94 (2):459–71. doi: 10.3945/ajcn.110.004374.
- White, P. J., A. L. Lapworth, J. An, L. Wang, R. W. McGarrah, R. D. Stevens, O. Ilkayeva, T. George, M. J. Muehlbauer, J. R. Bain, et al. 2016. Branched-chain amino acid restriction in zucker-fatty rats improves muscle insulin sensitivity by enhancing efficiency of fatty acid oxidation and Acyl-glycine export. Molecular Metabolism 5 (7):538–51. doi: 10.1016/j.molmet.2016.04.006.
- White, P. J., A. L. Lapworth, R. W. McGarrah, L. C. Kwee, S. B. Crown, O. Ilkayeva, J. An, M. W. Carson, B. A. Christopher, J. R. Ball, et al. 2020. Muscle-liver trafficking of BCAA-derived nitrogen underlies obesity-related glycine depletion. Cell Reports 33 (6):108375. doi: 10.1016/j.celrep.2020.108375.
- White, P. J., R. W. McGarrah, P. A. Grimsrud, S.-C. Tso, W.-H. Yang, J. M. Haldeman, T. Grenier-Larouche, J. An, A. L. Lapworth, I. Astapova, et al. 2018. The BCKDH kinase and phosphatase integrate BCAA and lipid metabolism via regulation of ATP-citrate lyase. Cell Metabolism 27 (6):1281–93.e7. doi: 10.1016/j.cmet.2018.04.015.
- Williamson, J. R., E. Wałajtys-Rode, and K. E. Coll. 1979. Effects of branched chain α-ketoacids on the metabolism of isolated rat liver cells. I. Regulation of branched chain α-ketoacid metabolism. Journal of Biological Chemistry 254 (22):11511–20. doi: 10.1016/S0021-9258(19)86514-6.
- Wolfe, R. R. 2017. Branched-chain amino acids and muscle protein synthesis in humans: Myth or reality?Journal of the International Society of Sports Nutrition 14:30. doi: 10.1186/s12970-017-0184-9.
- Wondmkun, Y. T. 2020. Obesity, insulin resistance, and type 2 diabetes: Associations and therapeutic implications. Diabetes, Metabolic Syndrome and Obesity 13 (October):3611–6. doi: 10.2147/DMSO.S275898.
- Wu, J., M. Zhao, C. Li, Y. Zhang, and D. W. Wang. 2021. The SARS-CoV-2 induced targeted amino acid profiling in patients at hospitalized and convalescent stage. Bioscience Reports 41 (3):BSR20204201. doi: 10.1042/BSR20204201.
- Würtz, P., Q. Wang, A. J. Kangas, R. C. Richmond, J. Skarp, M. Tiainen, T. Tynkkynen, P. Soininen, A. S. Havulinna, M. Kaakinen, et al. 2014. Metabolic signatures of adiposity in young adults: Mendelian randomization analysis and effects of weight change. PLoS Medicine 11 (12):e1001765. doi: 10.1371/journal.pmed.1001765.
- Xiao, F., Z. Huang, H. Li, J. Yu, C. Wang, S. Chen, Q. Meng, Y. Cheng, X. Gao, J. Li, et al. 2011. Leucine deprivation increases hepatic insulin sensitivity via GCN2/MTOR/S6K1 and AMPK pathways. Diabetes 60 (3):746–56. doi: 10.2337/db10-1246.
- Xiao, F., J. Yu, Y. Guo, J. Deng, K. Li, Y. Du, S. Chen, J. Zhu, H. Sheng, and F. Guo. 2014. Effects of individual branched-chain amino acids deprivation on insulin sensitivity and glucose metabolism in mice. Metabolism 63 (6):841–50. doi: 10.1016/j.metabol.2014.03.006.
- Xu, F., S. Tavintharan, C. Fang Sum, K. Woon, S. C. Lim, and C. N. Ong. 2013. Metabolic signature shift in type 2 diabetes mellitus revealed by mass spectrometry-based metabolomics. Journal of Clinical Endocrinology and Metabolism 98 (6):1060–5. doi: 10.1210/jc.2012-4132.
- Xu, M., Y. Kitaura, T. Ishikawa, Y. Kadota, C. Terai, D. Shindo, T. Morioka, M. Ota, Y. Morishita, K. Ishihara, et al. 2017. Endurance performance and energy metabolism during exercise in mice with a muscle-specific defect in the control of branched-chain amino acid catabolism. PLoS One 12 (7):e0180989. doi: 10.1371/journal.pone.0180989.
- Xu, M., M. Nagasaki, M. Obayashi, Y. Sato, T. Tamura, and Y. Shimomura. 2001. Mechanism of activation of branched-chain alpha-keto acid dehydrogenase complex by exercise. Biochemical and Biophysical Research Communications 287 (3):752–6. doi: 10.1006/bbrc.2001.5647.
- Xu, M., Q. Qi, J. Liang, G. A. Bray, F. B. Hu, F. M. Sacks, and L. Qi. 2013. Genetic determinant for amino acid metabolites and changes in body weight and insulin resistance in response to weight-loss diets: The preventing overweight using novel dietary strategies (POUNDS LOST) trial. Circulation 127 (12):1283–9. doi: 10.1161/CIRCULATIONAHA.112.000586.
- Yadav, A., N. Shah, P. Kumar Tiwari, K. Javed, Q. Cheng, I. S. Aidhen, and S. Bröer. 2020. Novel chemical scaffolds to inhibit the neutral amino acid transporter B0AT1 (SLC6A19), a potential target to treat metabolic diseases. Frontiers in Pharmacology 11 (February)::140. doi: 10.3389/fphar.2020.00140.
- Yang, Z., S. Huang, D. Zou, D. Dong, X. He, N. Liu, W. Liu, and L. Huang. 2016. Metabolic shifts and structural changes in the gut microbiota upon branched-chain amino acid supplementation in middle-aged mice. Amino Acids 48 (12):2731–45. doi: 10.1007/s00726-016-2308-y.
- Yao, J., J. P. Kovalik, O. F. Lai, P. Ching Lee, A. Eng, W. Hoong Chan, K. W. Tham, E. Lim, Y. Mong Bee, and H. C. Tan. 2019. Comprehensive assessment of the effects of sleeve gastrectomy on glucose, lipid, and amino acid metabolism in Asian individuals with morbid obesity. Obesity Surgery 29 (1):149–58. doi: 10.1007/s11695-018-3487-2.
- Yoneshiro, T., Q. Wang, K. Tajima, M. Matsushita, H. Maki, K. Igarashi, Z. Dai, P. J. White, R. W. McGarrah, O. R. Ilkayeva, et al. 2019. BCAA catabolism in brown fat controls energy homeostasis through SLC25A44. Nature 572 (7771):614–9. doi: 10.1038/s41586-019-1503-x.
- Yu, D., N. E. Richardson, C. L. Green, A. B. Spicer, M. E. Murphy, V. Flores, C. Jang, I. Kasza, M. Nikodemova, M. H. Wakai, et al. 2021. The adverse metabolic effects of branched-chain amino acids are mediated by isoleucine and valine. Cell Metabolism 33 (5):905–22.e6. doi: 10.1016/j.cmet.2021.03.025.
- Yuan, X. W., S. F. Han, J. W. Zhang, J. Y. Xu, and L. Q. Qin. 2015. Leucine supplementation improves leptin sensitivity in high-fat diet fed rats. Food & Nutrition Research 59 (June):27373. doi: 10.3402/fnr.v59.27373.
- Yue, S.-J., J. Liu, A.-T. Wang, X.-T. Meng, Z.-R. Yang, C. Peng, H.-S. Guan, C.-Y. Wang, and D. Yan. 2019. Berberine alleviates insulin resistance by reducing peripheral branched-chain amino acids. American Journal of Physiology. Endocrinology and Metabolism 316 (1):E73–E85. doi: 10.1152/ajpendo.00256.2018.
- Zarfeshani, A., S. Ngo, and A. M. Sheppard. 2014. Leucine alters hepatic glucose/lipid homeostasis via the myostatin-AMP-activated protein kinase pathway: Potential implications for nonalcoholic fatty liver disease. Clinical Epigenetics 6 (1):27. doi: 10.1186/1868-7083-6-27.
- Zhang, B., Y. Chen, X. Shi, M. Zhou, L. Bao, K. J. Hatanpaa, T. Patel, R. J. DeBerardinis, Y. Wang, and W. Luo. 2021. Regulation of branched-chain amino acid metabolism by hypoxia-inducible factor in glioblastoma. Cellular and Molecular Life Sciences 78 (1):195–206. doi: 10.1007/s00018-020-03483-1.
- Zhang, F., S. Zhao, W. Yan, Y. Xia, X. Chen, W. Wang, J. Zhang, C. Gao, C. Peng, F. Yan, et al. 2016. Branched chain amino acids cause liver injury in obese/diabetic mice by promoting adipocyte lipolysis and inhibiting hepatic autophagy. EBioMedicine 13 (November):157–67. doi: 10.1016/j.ebiom.2016.10.013.
- Zhang, Y., K. Guo, R. E. LeBlanc, D. Loh, G. J. Schwartz, and Y. H. Yu. 2007. Increasing dietary leucine intake reduces diet-induced obesity and improves glucose and cholesterol metabolism in mice via multimechanisms. Diabetes 56 (6):1647–54. doi: 10.2337/db07-0123.
- Zhao, H., H. Li, A. C. K. Chung, L. Xiang, X. Li, Y. Zheng, H. Luan, L. Zhu, W. Liu, Y. Peng, et al. 2019. Large-scale longitudinal metabolomics study reveals different trimester-specific alterations of metabolites in relation to gestational diabetes mellitus. Journal of Proteome Research 18 (1):292–300. doi: 10.1021/acs.jproteome.8b00602.
- Zhao, H., F. Zhang, D. Sun, X. Wang, X. Zhang, J. Zhang, F. Yan, C. Huang, H. Xie, C. Lin, et al. 2020. Branched-chain amino acids exacerbate obesity-related hepatic glucose and lipid metabolic disorders via attenuating Akt2 signaling. Diabetes 69 (6):1164–77. doi: 10.2337/db19-0920.
- Zhao, J., B. Zhai, S. P. Gygi, and A. L. Goldberg. 2015. MTOR inhibition activates overall protein degradation by the ubiquitin proteasome system as well as by autophagy. Proceedings of the National Academy of Sciences of the United States of America 112 (52):15790–7. doi: 10.1073/pnas.1521919112.
- Zhao, L., M. Wang, J. Li, Y. Bi, M. Li, and J. Yang. 2019. Association of circulating branched-chain amino acids with gestational diabetes mellitus: A meta-analysis. International Journal of Endocrinology and Metabolism 17 (3):85413. doi: 10.5812/ijem.85413.
- Zhao, X., Q. Han, Y. Liu, C. Sun, X. Gang, and G. Wang. 2016. The relationship between branched-chain amino acid related metabolomic signature and insulin resistance: A systematic review. Journal of Diabetes Research 2016:2794591. doi: 10.1155/2016/2794591.
- Zheng, Y., Y. Li, Q. Qi, A. Hruby, J. E. Manson, W. C. Willett, B. M. Wolpin, F. B. Hu, and L. Qi. 2016. Cumulative consumption of branched-chain amino acids and incidence of type 2 diabetes. International Journal of Epidemiology 45 (5):1482–92. doi: 10.1093/ije/dyw143.
- Zhou, J., J. Wu, F. Zheng, M. Jin, and H. Li. 2015. Glucagon-like peptide-1 analog-mediated protection against cholesterol-induced apoptosis via mammalian target of rapamycin activation in pancreatic ΒTC-6 cells-1mTORβTC-6. Journal of Diabetes 7 (2):231–9. doi: 10.1111/1753-0407.12177.
- Zhou, M., J. Shao, C.-Y. Wu, L. Shu, W. Dong, Y. Liu, M. Chen, R. M. Wynn, J. Wang, J. Wang, et al. 2019. Targeting BCAA catabolism to treat obesity-associated insulin resistance. Diabetes 68 (9):1730–46. doi: 10.2337/db18-0927.