Abstract
Lipid oxidation is a complex process in muscle-based foods (red meat, poultry and fish) causing severe quality deterioration, e.g., off-odors, discoloration, texture defects and nutritional loss. The complexity of muscle tissue -both composition and structure- poses as a formidable challenge in directly clarifying the mechanisms of lipid oxidation in muscle-based foods. Therefore, different in vitro model systems simulating different aspects of muscle have been used to study the pathways of lipid oxidation. In this review, we discuss the principle, preparation, implementation as well as advantages and disadvantages of seven commonly-studied model systems that mimic either compositional or structural aspects of actual meat: emulsions, fatty acid micelles, liposomes, microsomes, erythrocytes, washed muscle mince, and muscle homogenates. Furthermore, we evaluate the prospects of stem cells, tissue cultures and three-dimensional printing for future model system development. Based on this reviewing of oxidation models, tailoring correct model to different study aims could be facilitated, and readers are becoming acquainted with advantages and shortcomings. In addition, insight into recent technology developments, e.g., stem cell- and tissue-cultures as well as three-dimensional printing could provide new opportunities to overcome the current bottlenecks of lipid oxidation studies in muscle.
1. Introduction
Lipid oxidation of muscle foods (fish, poultry, and red meat) is a large research area with around 70 years of history which relates to degradation of various parameters of quality, such as odor, flavor, color, texture, and nutritional value (Dominguez et al. Citation2019; Undeland Citation2016; Wu, Richards, and Undeland Citation2022b). Research trends have changed according to the development of analysis methods and food scientists’ efforts. In the 1950s–1980s, many studies focused on detecting links between lipid oxidation and fatty acid patterns, lipid classes/contents and endogenous pro- or antioxidants (Lee et al. Citation1975; Tappel Citation1955; Wills Citation1966). Then the focus shifted to investigation of free radical chain reactions (Bielski et al. Citation1985; Boveris, Galatro, and Puntarulo Citation2000; Puppo and Halliwell Citation1988). In the last 20 years, the greatest steps forward have been taken within the area of heme protein- (hemoglobin, Hb and myoglobin, Mb) mediated lipid oxidation (Richards et al. Citation2007; Undeland, Hultin, and Richards Citation2002; Undeland, Kristinsson, and Hultin Citation2004; Wu et al. Citation2017), as well as within the area of oxidation prevention in muscle foods using different natural antioxidants.
Muscle tissue is a complex system that contains many components, e.g., lipid substrates in the form of triacylglycerols and phospholipids, pro- and antioxidants, as well as different muscle proteins; sarcoplasmic reticulum, myofibrillar proteins and connective tissues (Medina et al. Citation2012). The membrane of a muscle cell contains phospholipids which are vulnerable to lipid oxidation due to their large surface area and degrees of unsaturation (Cui and Decker Citation2016). Muscle microstructure can affect lipid-pro-oxidant interactions, which has been shown to cause varying oxidation rates depending on the animal species from which the muscle was obtained (Wu et al. Citation2021b).
Because of the complexity of muscle tissues, in vitro model systems, e.g., emulsions, fatty acid micelles, liposomes, microsomes, erythrocyte, washed muscle mince and muscle homogenates have been used to study the mechanisms of lipid oxidation. However, one should exercise caution when extrapolating findings from model systems to postmortem muscle tissue, and it is important to discuss pros and cons of the different models used over the years to simulate muscle foods. To the best of our knowledge, there are so far no attempts to review, summarize, or compare the different model systems used in muscle lipid oxidation research. Thus, we here review the seven most commonly applied model systems and discuss their principle, preparation, and application. The advantages and drawbacks of each model are summarized, and we provide some comments about future prospects.
2. Endogenous muscle factors associated with lipid oxidation
Before discussing individual model systems and how they can be used to study the influence of exogenous factors, we will address the critical endogenous factors relevant to lipid oxidation because that knowledge is the basis for model system design. In this review, we discuss muscle model systems that are used mainly to study lipid oxidation pathways, mechanisms, and the influence of exogenous factors; thus, we will not discuss the endogenous antioxidants in muscle foods. Many excellent reviews about endogenous antioxidants have already been published, with examples being those of Dominguez et al. (Citation2019), Undeland (Citation2016) and Wu, Richards, and Undeland (Citation2022b).
2.1. Muscle structure
Although there are more than 600 different muscle types that vary in shape, size, and activity, at the cellular level, there is close resemblance among muscles from a variety of vertebrate animals (Erickson Citation2008). However, fish muscle structure is largely different from that of avian and mammalian muscles. For example, fibers diameter from fish muscle (>50 µm) tend to be bigger than muscle fibers from red meat (<50 µm), and fish muscle generally contains fewer mitochondria, a thinner sarcolemma, and more sarcoplasm (Erickson Citation2008). Also, there is significantly less of connective tissue proteins in fish given the fact that fish is supported by the surrounding water, i.e., does not need to carry its own weight.
The variability in muscle structure could contribute to the different oxidative stability between fish and red meat (Wu et al. Citation2021b). The complex morphologies of distinct layers of muscle are indeed difficult to replicate in a model system. Comparisons of the microstructures between washed cod muscle mince and washed pig muscle mince showed that the washed mammalian muscle had denser and wrapped structures, which was hypothesized to hinder accessibility of pro-oxidants to lipid substrates (Wu et al. Citation2021b). For the structure of fish muscle, myocommata is a special structure to link single muscle cell or fiber. Furthermore, myocommata has a large surface/volume ratio and is closed to fat cells, which are easier to access the pro-oxidants (e.g., Hb, and Mb) compared those wrapped fat cells in mammalian muscle (Sharifian et al. Citation2014). For example, it has been reported that myocommata started to deteriorate after the fish dies and then generate gaping owing to the isolation of myotomes (Chéret et al. Citation2005). The generating gaping in fish could better facilitate fat cells or membrane of muscle cells access oxygen and Hb. Thus, muscle structure has an important influence on lipid oxidation.
2.2. Lipid substrates
Unsaturated fatty acids are abundant substrates for lipid oxidation in muscle foods (Vieira, Zhang, and Decker Citation2017). Thus, free unsaturated fatty acids have sometimes been used as lipid substrates in different models (Hajimohammadi Citation2020; Hajimohammadi and Bagheri Citation2017; Hajimohammadi and Nosrati Citation2018). In muscle food, lipids are primarily referred to triacylglycerols and phospholipids, with small amounts of free fatty acids (FFAs), cholesterol, and tocopherols (Pereira and Abreu Citation2018). Although triacylglycerol contribute to a large proportion to the total lipid in muscle food, particularly in more fatty cuts or species, membrane phospholipids have been regarded as the most important substrates in lipid oxidation of muscle, meaning that the total lipid content seems to play very little role (Undeland, Hultin, and Richards Citation2002; Wu, Abdollahi, and Undeland Citation2021a). Both the degree of unsaturation and the concentration of phospholipids have generally been considered as crucial factors in lipid oxidation in muscle food (Amaral, Da Silva, and Lannes Citation2018). However, we (Wu et al. Citation2021b) found that differently unsaturated phospholipids (polyenoic indexes of 282 vs 24) at the same concentration had a similar rate of oxidation when Mb was the pro-oxidant in a washed pig muscle mince system. Our study also suggested that the accessibility of pro-oxidants to phospholipids may be more important than their degree of saturation (Wu et al. Citation2021b). Similarly, lipid oxidation results retrieved from ice storage of three types of minced fish backbones derived from herring, cod, salmon did not show any significant correlation with the degree of unsaturation and the content of polyunsaturated fatty acids (PUFA), but significant correlation with Hb levels (Wu, Abdollahi, and Undeland Citation2021a). In addition, Richards and Hultin (Citation2001) reported that trout Hb with 0.01% lipids generated a strong rancid odor and significantly increased TBARS values in washed cod model during ice storage, but the rate and extent of rancidity were not increased by the presence of >6 times more membrane phospholipids. These results indicated the endogenous pro-oxidants (such as Hb, and Mb) played more important roles in lipid oxidation than the degree of unsaturation and the concentration of phospholipids.
Another important point is that fatty acids exist as both free and esterified forms although the FFA concentration is low in fresh muscle food (Ahmmed et al. Citation2021). The classic theory asserts that FFAs oxidize more readily than their esterified counterparts (Labuza and Dugan Citation1971). However, we have found that the hydrolysis of lipids or the addition of FFAs appears to inhibit oxidation in turkey mince (Wu et al. Citation2021c) and washed cod muscle (Tatiyaborworntham and Richards Citation2018; Tatiyaborworntham, Yin, and Richards Citation2021). Thus, the choice of fatty acid form -free or esterified- is important when a model muscle system is designed.
2.3 Endogenous pro-oxidants
The presence of transition metals, e.g., Fe and Cu (in either free or complex forms) is an important parameter that affects the oxidative stability of muscle food (Chaijan and Panpipat Citation2017). The primary sources of Fe in muscle are heme proteins, Hb and Mb (Wu, Ghirmai, and Undeland Citation2020; Wu et al. Citation2017). Heme protein concentrations in meat depend on animal species, muscle type, and anatomical location of muscle (Min and Ahn Citation2005) as well as slaughtering and bleeding techniques (Alvarado et al. Citation2007; Sabow et al. Citation2016). Hemin release from these heme proteins is thought to be responsible for oxidation of lipids due to ability to decompose pre-formed lipid hydroperoxides (LOOHs) into lipid free radicals and secondary products (Wu, Richards, and Undeland Citation2022b). The contribution of free iron to lipid and protein oxidation was found to be predominant during cooking (Zhang et al. Citation2022).
Oxidoreductase enzymes also have impact on lipid oxidation. Lipoxygenase (LOX) has been identified as an important pro-oxidant in fish muscle (Tolasa Yılmaz et al. Citation2018; Wu et al. Citation2022a). In addition, microsomal peroxidases and dioxygenases can initiate lipid oxidation in meat (Min et al. Citation2008). In the presence of LOX, conjugated diene hydroperoxy products may be generated with hydrogen abstraction from the allylic methylene position of PUFA (Hui Citation2006). Muscle myeloperoxidase is an enzyme that catalyzes hypochlorous acid generation from H2O2 and chloride. Subsequently, hypochlorous acid reacts with O2•− to produce •OH (Folkes, Candeias, and Wardman Citation1995). Interestingly, myeloperoxidase generates •OH six times faster than the Fenton reaction (Hui Citation2006).
3. Model systems
It is important to note that most models used to simulate muscle were developed from the perspective of the lipid substrate; thus, assuming the lipids are the most important principal component of muscle models. Muscle oxidation models are normally prepared by the following four approaches: 1) selecting relevant lipids of muscle and mix them to obtain a model, e.g., emulsion, fatty acid micelles, or liposomes; 2) select cells that contain both oxidants and lipid substrate, e.g., erythrocytes; 3) extract components of interest, exclude components of less interest, to simulate actual muscle structure, e.g., microsomes and washed muscle mince; 4) modify the actual muscle, e.g., diluting it such as in muscle homogenates.
3.1. Emulsions
An emulsion is a system that contains two immiscible liquids as shown in . In general, oil-in-water emulsions consist of three different components: water (the dispersing phase), lipids (the dispersed phase), and surface-active agent (the interface) (Hu, Decker, and Mcclements Citation2019). Emulsion models for oxidation studies are prepared by mixing a source of unsaturated lipids (e.g., triacylglycerols from oils, oils stripped of their antioxidants, fatty acid esters or FFAs) with an aqueous phase containing surface-active agents (e.g., Tween-20, proteins or sodium dodecyl sulfate) into an oil-in-water (O/W) emulsion (Wills Citation1964; Yin et al. Citation1992). The mixture is typically made into a coarse emulsion with a low mixer and then particle size is further reduced using equipment such as homogenizers or sonicators. After emulsification, the prooxidants and/or antioxidants of interest can be added. The molecule of interest will partition to various degrees into the hydrophobic emulsion droplet core, the aqueous phase or it will interact with the surfactants at the emulsion droplet interface. Since some of the surfactant partitions into the aqueous phase once the droplet interface is saturated, surfactant micelles will also commonly exist in the aqueous phase. These surfactant micelles can solubilize the prooxidants and antioxidants of interest which can impact their reactivity (Inchingolo et al. Citation2021).
Figure 1. Fatty acid micelle and emulsion models. Above their critical micellar concentration, amphiphilic compounds tend to spontaneously self-aggregate to form lipid micelles by hiding the hydrophobic part inside and exposing the hydrophilic part to the aqueous medium. Amphiphilic emulsifiers can surround and stabilize oil droplets that are formed by physical agitation of the mixture between oil and aqueous medium. In this figure the roles of water-soluble, oil-soluble, and amphiphilic antioxidants on lipid oxidation catalyzed by free iron in the Fenton reaction scheme are presented. Due to their hydrophilic part, fatty acids and lipid hydroperoxides may jump in and out between lipid micelles and interact with aqueous compounds, e.g., Mb and Hb.
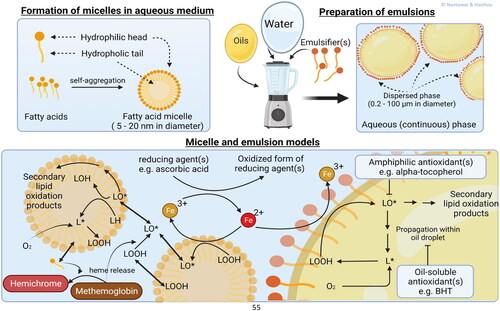
In early research on lipid oxidation of meat or fish (1955–1975), many food scientists studied oxidation from only a chemical point of view (Lee et al. Citation1975; Liu Citation1970; Tappel Citation1955; Wills Citation1966). Identification of the catalytic activity of pro-oxidants (e.g., Mb, Hb, nonheme iron and copper) and the reaction products were a large focus (Burri et al. Citation2020; Lee et al. Citation1975). Emulsion models are however also useful for studying the partitioning of oxidized lipids into environments of different polarity. Nuchi et al. (Citation2002) reported that the polarity of LOOHs causes them to migrate to the interface of the emulsion droplet where they can interact with water soluble prooxidants, which is in accordance to what was observed in lipid bilayer modes (Junqueira et al. Citation2021).
The disadvantage of using oil-in-water emulsions as a model system is that they differ from cell membranes, the primary location of oxidation in muscle. For example, oil-in-water emulsions will have a larger lipid core that could host more lipid soluble components. They will also have less surface area than cell membranes at an equal lipid concentration and they could differ in charge depending on the surfactants used (Mcclements and Decker Citation2000). Emulsion models may be more applicable to certain muscle-derived food products, e.g., emulsion-type sausages and emulsified surimi products. However, the roles of muscle proteins in stabilizing lipid droplets should then be considered as well (Jiang and Xiong Citation2015).
3.2. Fatty acid micelles
Fatty acid micelles have been used in many studies on the fundamental aspects of lipid oxidation due to ease of preparation and high oxidizability thanks to high surface/volume (). Unlike emulsion models, micelles can spontaneously form upon reaching a certain concentration, the critical micelle concentration, of surface active molecules as shown in (Mohajeri and Noudeh Citation2012). Co-surfactants, e.g., sodium dodecyl sulfate or Triton X may be included to increase micelle stability (Hauville et al. Citation1998). Typically, fatty acid micelles are between 5 and 20 nm in diameter, i.e., a thousand times smaller than emulsion droplets (Mohajeri and Noudeh Citation2012).
Table 1. Main characteristics, advantages and disadvantages of emulsions, fatty acid micelle, erythrocyte, meat homogenate and washed muscle models.
The extent to which fatty acid micelles exists in meat and meat products is unclear, but they can still provide information to monitor processes associated with lipid oxidation (Vitrac et al. Citation2005). Several micellar model studies have been used to define factors that affect lipid oxidation, such as the radiation dose rate (Mekhloufi et al. Citation2005), the concentration of lipids (Scollo et al. Citation2018), the fatty acid nature and the number of double bonds (Pliss et al. Citation2021), the ionic strength of the medium (Raleigh and Kremers Citation1978) and the pH (Johnson, Inchingolo, and Decker Citation2018). The role of muscle food pro-oxidants, e.g., Mb and Hb in stimulating lipid oxidation has also been studied in fatty acid micelles Baron, Skibsted, and Andersen (Citation2000).
3.3. Liposomes
Liposomes are spherical phospholipid vesicles with at least one concentric bilayer encircling a hydrophilic space. Liposomes can be used as carriers for both water-soluble and lipid-soluble compounds (Akbarzadeh et al. Citation2013). A visualization of liposomes was shown in . In muscle tissues, membrane phospholipids in sarcoplasmic reticulum and sarcolemma are susceptible to oxidative degradation because of the high content of unsaturated fatty acids required to maintain membrane fluidity and the large surface area in direct contact with oxidants and pro-oxidants (Heden, Neufer, and Funai Citation2016). Therefore, being structurally similar to phospholipid membranes, liposomes have a long history for studying kinetics and mechanisms of lipid oxidation in meat systems (Medina et al. Citation2012; Schnitzer, Pinchuk, and Lichtenberg Citation2007).
Figure 2. Liposome models. A liposome preparation scheme shows how water- and lipid- soluble additives can be incorporated into the lipid vesicles. The lipid-soluble additives stay within the lipid bilayers, while the water-soluble additives in the aqueous medium come into contact with the lipid bilayers via different modes of interactions, e.g., electrostatic attraction, hydrophobic interaction. Liposomal models for studying lipid oxidation by free iron and Mb are presented.
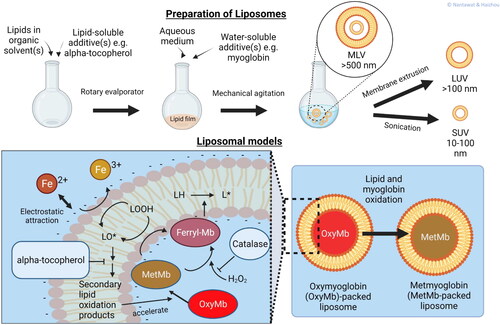
Liposomes can be prepared by several different methods. Unilamellar liposomes are prepared by hydration of dried lipid films with an aqueous medium. Multilamellar liposomes are prepared by dispersing phospholipids in aqueous media and then decreasing their size by sonication membrane extrusion, or homogenization (Frenzel and Steffen-Heins Citation2015; Guldiken et al. Citation2018). Other available methods to make liposomes include are solvent dispersion methods, e.g., ethanol injection, reverse phase evaporation vesicles, and detergent removal methods (Akbarzadeh et al. Citation2013). Membrane extrusion is employed for obtaining uniformly-sized lipid vesicles.
Liposomes can be prepared from synthetic or natural phospholipids. The major advantage of synthetic phospholipids is that the particular fatty acids esterified to the glycerol backbone are well defined and customizable to meet experimental requirements. Phosphatidylcholine and phosphatidylethanolamine are commonly used, being the most abundant phospholipids in cell membranes (Alberts et al. Citation2002; Salgo, Corongiu, and Sevanian Citation1992). Natural phospholipids can be obtained from muscle tissues (Genot et al. Citation1992). Lipid-soluble additives, e.g., alpha-tocopherol (Yin et al. Citation2012), cholesterol (Mosca, Ceglie, and Ambrosone Citation2011), and azo-radical initiator (Culbertson and Porter Citation2000), may also be incorporated into the bilayers during the preparation of dried lipid films. Water-soluble components, e.g., Mb may be packed within the void inside liposomes during the hydration of the lipid films (Yin and Faustman Citation1993).
In addition to the degree of unsaturation, size and surface charge (zeta potential) are important physicochemical characteristics that influence oxidation of liposomes (Schnitzer, Pinchuk, and Lichtenberg Citation2007). Varying by size and number of lamellae, the three most common types of liposomes are small unilamellar vesicles (SUV; size 20–100 nm), large unilamellar vesicles (LUV; size > 100 nm), and multilamellar vesicles (MLV; size > 500 nm) (Chinnagounder Periyasamy et al. Citation2012). Compared with LUV, the small size of SUV not only increases the surface area, but also surface curvature and permeability of the bilayers which ultimately destabilize the lipid vesicles against transition metal-promoted lipid oxidation (Li, Yeo, and Tan Citation2000). Genot et al. (Citation1992) compared different liposomal preparation methods and suggested that LUV are suitable models for lipid oxidation studies because of uniform size, structural integrity upon oxidation, and low spectrophotometric interference.
Surface charge of liposomes is influenced by ionic strength, and pH and may affect oxidative stability of liposomes (Mozuraityte, Rustad, and Storr⊘ Citation2006). Negatively charged phospholipids attract transition metal ions, e.g., iron and copper, in close proximity to oxidizable lipids (Dacaranhe and Terao Citation2001; Gal, Pinchuk, and Lichtenberg Citation2003), although the effect of this phenomenon on lipid oxidation seems inconsistent. Thanonkaew et al. (Citation2007) found that NaCl at 0.2–2.0% retarded iron-promoted oxidation of squid liposomes, possibly because of displacement of bound iron from the liposomal surface. However, Dacaranhe and Terao (Citation2001) found that dipalmitoyl-phosphatidylserine at 10%mol exhibited both iron-binding ability and an antioxidant activity against iron-promoted oxidation of egg yolk phosphatidylcholine-containing liposomes.
Related to lipid oxidation in muscle foods, free iron, free heme, and heme proteins (Hb and Mb) are often used as pro-oxidants in mechanistic studies of liposomal oxidation (Carvajal et al. Citation2009; Litvinko, Skorostetskaya, and Gerlovsky Citation2018; Lynch and Faustman Citation2000; Min, Nam, and Ahn Citation2010; Thanonkaew et al. Citation2007; Yamauchi et al. Citation2017). Furthermore, mechanisms of both lipid and Mb oxidation, related to rancidity and discoloration of meat, respectively, were studied simultaneously (Faustman et al. Citation2010). Abraham et al. (Citation2016) studied endogenous antioxidant capacity of beef by extracting and incorporating Mb-containing sarcoplasm into liposomes which were stored under fluorescent illumination. The investigators found positive correlations between Mb oxidation, lipid oxidation, and oxidation-reduction potential of sarcoplasm-containing liposomes. Using oxymyoglobin-containing liposomes, Yin and Faustman (Citation1993) found a dissimilar effect of phospholipid headgroups on redox stability of oxyMb. With a similar fatty acid profile, the phosphatidylethanolamine-based liposome promoted more lipid and Mb oxidation than the phosphatidylcholine-based liposome. Chan et al. (Citation1997) pointed out the activity of H2O2 in the oxidation of both oxymyoglobin and lipid oxidation by including superoxide dismutase (SOD) and catalase (CAT) in the liposome system. Liposomes are also used in combination with meat system. Chen et al. (Citation2017) prepared liposomes containing Porphyra haitanensis-derived phycobiliproteins which were added to a liposome-meat model; the authors found that the active compounds inhibited oxidation of the composite model.
Interactions between membranes with lipid-soluble antioxidants and pro-oxidants have been studied using liposomal models. For example, Szebeni et al. (Citation1988) investigated binding of Hb to the surface of liposomal bilayers, a phenomenon that was counteracted by inclusion of cholesterol to promote fluidity to the liposomal membranes and hinder the partition of Hb into the membranes. Small-angle X-ray diffraction is a useful technique for elucidating the relationship between membrane order and oxidizability. Using this technique, Mcnulty et al. (Citation2007) found contrasting effects of different carotenoids on membrane structure alteration and autooxidation of liposomal lipids. The authors reported that membrane disorder caused by lycopene and beta-carotene caused increased lipid oxidation, whereas membrane structure-preserving astaxanthin exhibited antioxidant activity.
Simple and customizable liposome models enable fundamental and mechanistic research on lipid oxidation and antioxidants because components can be selectively included or excluded. Despite the benefits, liposomal vesicles may not be a true representative of muscle membranes in terms of shape and composition. Nevertheless, this lipid model can be desirable for the meat industry to gain rapid recognition of potential antioxidants (Nieto, Huvaere, and Skibsted Citation2011).
3.4. Microsomes
Microsomes () are vesicle-like fragments of endoplasmic reticulum (between 20 and 200 nm diameter) or other membranes (Yin et al. Citation2013). Being compositionally similar to cellular membranes, microsomes thus provide versatile models for lipid oxidation studies related to muscle quality. The model allows studies of the contribution of enzymatic systems to the total degree of lipid oxidation as well as the deposition and metabolism of dietary supplements.
Figure 3. Microsome models. A simplified preparative procedure of microsomes from muscle tissues is illustrated. The presence of membrane-bound enzymes make microsomes versatile models for studying both enzymatic- and non-enzymatic lipid oxidation pathways as well as metabolism of dietary antioxidants can be studied using microsomal models. Muscle-derived microsomal models also connect between dietary supplementation studies and lipid oxidation studies.
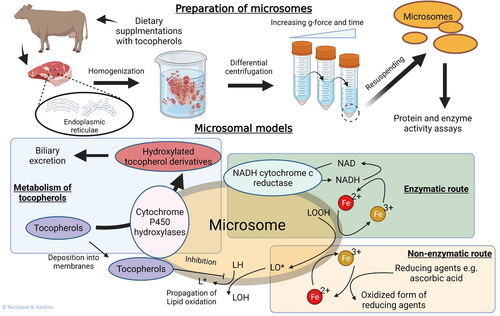
Preparation of microsomes involves tissue homogenization and differential centrifugation to obtain the subcellular fraction. Although hepatic microsomes are common, microsomes can also be prepared from skeletal muscle (Kathirvel and Richards Citation2012; Rey, Lopez-Bote, and Arias Citation1997). Homogenization of tissue samples with a Dounce homogenizer or a Potter-Elvehjem glass with Teflon pestle should be conducted with gentle strokes to prevent foaming (Sabatini Citation2014). During preparation, a constant temperature below 4 °C is important to delay oxidative degradation of lipids and thermal denaturation of proteins. Isolation buffers often contain cocktails of protease- and phosphatase-inhibitors that are required to prevent unintentional proteolysis and phospholysis. At the end of the preparation, protein and enzyme activity assays are performed to assess quality and standardize the model.
Being derived from muscle, microsomes have the composition of muscle membranes and carry various types of phospholipids, cholesterol, and membrane-bound proteins. Some membrane-bound proteins integrated into microsomes possess catalytic activity, e.g., glutathione-dependent transferases, peroxidases, and cytochrome P450 enzymes (Guengerich Citation1977; Hosoya, Matsukawa, and Nagai Citation1971; Jakobsson et al. Citation1997). Generally, determination of microsomal lipid oxidation can be performed in a manner similar to the methods used for liposomes. An exception is that the determination of conjugated dienes requires extraction of microsomal lipids prior to spectrophotometric measurements because of the light scattering effect of the heterogeneous microsomes (Perera et al. Citation1985; Srinivasan and Recknagel Citation1971). It should also be noted that other compounds with conjugated double bonds can interfere with the measurement; therefore, blanks prepared from non-oxidized samples are used for subtracting the background light absorption. For in situ detection of microsomal lipid oxidation, an EPR-spin trapping technique has been employed for detecting lipid radicals generated from iron-mediated decomposition of LOOHs (Boveris, Galatro, and Puntarulo Citation2000).
Microsomal models offer many applications in lipid oxidation-related research. Numerous mechanistic studies of antioxidants and pro-oxidants have been reported (Ikeda et al. Citation2011; Kathirvel and Richards Citation2012; Pazos et al. Citation2006; Pazos, Medina, and Hultin Citation2005; Richards, Cai, and Grunwald Citation2009; Shewfelt and Hultin Citation1983), and microsomes are unique lipid models for studying both enzymatic and non-enzymatic lipid oxidation (Lin and Hultin Citation1976; Rhee, Dutson, and Smith Citation1984; Thanonkaew et al. Citation2005). The enzymatic pathway involves NADH- or NADPH-mediated reduction of ferric iron (Fe3+) to ferrous iron (Fe2+), thereby promoting the Fenton reaction in converting peroxides to free radicals (Svingen et al. Citation1979). In this scheme, oxidoreductases bound to sarcoplasmic reticulum and mitochondrial membranes are responsible for recycling NAD/NADH and NADP/NADPH. In contrast, the non-enzymatic route relies on reducing agents, e.g., ascorbic acid, to promote the Fe3+/Fe2+ conversion. In a very pure microsomal model, purified sarcoplasmic reticulum from winter flounder (Pseudopleuronectes americanus), Decker et al. (Citation1989) studied whether iron and copper promoted lipid oxidation in vitro at the concentrations found in the low molecular weight (LMW) fraction of flounder muscle press juice. Several studies with this model then followed the effect of cytosol, histidine or soluble and bound iron (Erickson and Hultin Citation1992; Huang and Hultin Citation1992), providing in depth mechanistic data on fish membranal lipid oxidation. It was however revealed that the order of adding pro-oxidants, particularly iron and ascorbate, was very important for the resulting lipid oxidation in the sarcoplasmic reticulum system (Soyer and Hultin Citation2000).
Unlike liposomes, dietary supplementation can enrich microsomes with PUFA and lipid-soluble antioxidants. This advantage enables microsomes to be used for determining deposition of such dietary supplements and their effects on microsomal oxidation. Rey, Lopez-Bote, and Arias (Citation1997) modified the fatty acid compositions of pork longissimus dorsi via feeds formulated with α-tocopherol acetate and different combinations of olive oil, sunflower oil, and linseed oil. Partial replacement of olive oil with linseed oil as a rich source of n-3 fatty acids improved the n-6:n-3 ratio in the muscle lipids but resulted in muscle microsomes with low oxidative stability. Protection was therefore required from α-tocopherol. Lynch and Faustman (Citation2000) and Yin et al. (Citation2013) used microsomes prepared from tissues with different α-tocopherol content for testing the pro-oxidative activity of Mb alkylated with lipid oxidation-derived aldehydes. Their results revealed microsomes with elevated concentrations of α-tocopherol delayed lipid and Mb oxidations.
Being compositionally similar to cellular membranes, microsomes thus provide versatile models for lipid oxidation studies related to muscle quality. The model allows studies of the contribution of enzymatic systems to the total degree of lipid oxidation as well as the deposition and metabolism of dietary supplements. However, a tedious preparation process and potential lack of consistency are drawbacks of microsomal models. Care should be exercised during the handling of raw materials (liver or skeletal muscle tissues) and during the actual isolation to avoid loss of enzymatic activities. In addition, the compositional variation in the raw materials, particularly, enzyme activity, fatty acid profile, and tocopherol content, may affect oxidative stability of microsomes.
3.5. Erythrocytes
In vertebrates, erythrocytes (red blood cells) transfer oxygen from the lungs or gills to body tissues via the circulatory system (Karabulut et al. Citation2009). The structure of erythrocyte membrane is composed of a phospholipid bilayer and imbedded proteins, and the membrane is the direct target of lipid oxidation (Davidovic-Plavsic et al. Citation2021). The importance of erythrocyte as a model for lipid oxidation study is due to the fact that residual blood can promote lipid oxidation upon hemolysis and release of Hb (Richards and Hultin Citation2002). Therefore, the ability of erythrocytes to withstand oxidative stress and stay intact is crucial for oxidative stability of muscle.
For erythrocyte preparation, non-coagulated whole blood is centrifuged, and the plasma and buffy coat layer are removed. After further washing, the red cells are suspended in normal saline (Ghirmai et al. Citation2020). Experimental procedures for measuring lipid oxidation can be based on methods described by Li et al. (Citation2016). This system to investigate external parameters of lipid oxidation utilizes physiological saline containing 1% erythrocytes and dimethyl sulfoxide. Then, oxidants (e.g., FeSO4 and H2O2) are added to induce oxidation. After incubation, the erythrocytes are recovered to measure various analytes including O2 concentration, H2O2, malondialdehyde, protein carbonyls, and the activities of SOD, CAT, and glutathione peroxidase (GPx).
There are additional examples of erythrocyte models to study effects of antioxidants, feed ingredients on lipid oxidation in muscles. Hua-Tao et al. (Citation2019) investigated effects of extract of Angelica sinensis and ethoxyquin on lipid oxidation in erythrocytes and on growth, digestion, and absorptive and antioxidant capacity in carp muscle. The results indicated that Angelica sinensis extracts and ethoxyquin at concentrations of 0.5 and 0.25 mg/ml suppressed lipid oxidation by decreasing the generation of reactive oxygen species and restoring the activities of antioxidants in hydroxyl radical-treated erythrocytes. Li et al. (Citation2016) revealed that the fish erythrocyte system can be used as an experimental model to evaluate lipid oxidation in food and feed ingredients. An ethyl acetate extract of Ginkgo biloba leaves was used as an inhibitor in a carp erythrocyte model. They concluded that the inhibition effects of the extract on lipid oxidation occurred because of the presence of flavonoid compounds. Pig erythrocytes and their ghosts were used in studies of hemolytic and protection activities of quaternary ammonium bromides and chlorides in the presence of UV-irradiation. Hemolytic activities increased with the lipophilicity of the compounds, and each bromide salt was more hemolytic than the chloride forms (Kleszczyfiska et al. Citation2003). Glutamine is an important dietary supplement and a major free L-amino acid in fish (Yan and Qiu-Zhou Citation2006). Alanine, citrulline and proline are the important metabolites of glutamine in rats (Windmueller Citation1982) and pigs (Wu, Borbolla, and Knabe Citation1994). Li et al. (Citation2013) showed that a mixture of glutamine, alanine, citrlulline, and proline significantly reduced •OH-induced lipid oxidation and protein oxidation in carp erythrocytes.
3.6. Washed muscle mince
A model system of washed muscle mince is composed most of muscle components primarily myofibrillar proteins and membrane phospholipids (Wu et al. Citation2022c) and maintains partial muscle structure (Cai et al. Citation2013; Richards, Modra, and Li Citation2002a; Xiao et al. Citation2018). A visualization of washed muscle mince is shown in . The washing process mostly removes undesired contents such as blood, lipids, heme pigments (Hb and Mb) and iron, to concentrate muscle proteins and membranes (Karayannakidis et al. Citation2008; Kunyaboon et al. Citation2021). Washed muscles used for monitoring of muscle lipid oxidation can be prepared from muscles of different animal species (Kunyaboon et al. Citation2021; Tatiyaborworntham and Richards Citation2018; Wu et al. Citation2021b; 2021c; Wu et al. Citation2022d; Wu et al. Citation2017). The removal of aqueous antioxidants and pro-oxidants from the ground muscle provides rapid assessment of lipid oxidation with the capacity to examine environmental and compositional aspects (Cui et al. Citation2018). For example, the relative ability of different pro-oxidants that are added to incur lipid oxidation can be examined at varying pH, and the ability of added antioxidants to potentially counteract progress of lipid oxidation can also be evaluated.
Figure 4. Washed muscle models. The washed muscles consist primarily of myofibrillar protein, connective tissues, and lipid membranes as the result of exhaustive washing of minced muscle tissues to remove water-soluble components, e.g., sarcoplasmic proteins and low molecular weight compounds. External pro-oxidants and antioxidants, as well as muscle components of interest can be added back to the washed muscle for studying their individual and interaction effects on lipid oxidation.
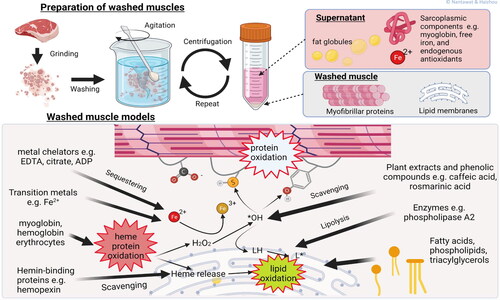
There are different washing procedures that provide different qualities of washed muscle from different species primarily due to variance in heme protein contents (Vallejo-Cordoba, Rodriguez-Ramirez, and Gonzalez-Cordova Citation2010). In general, the preparation of washed muscles requires grinding, washing with water, and centrifugation. Muscle mince is typically washed three or more times with potable water or buffers (< 5 °C) at a mince/water ratio of 1:3. Centrifugation is performed at each washing step to concentrate myofibrillar protein and lipid membranes. Any floating matters is removed manually (Halldorsdottir et al. Citation2013; Kunyaboon et al. Citation2021; Undeland, Hultin, and Richards Citation2002).
Washed muscles have been extensively used for studying various pro- and antioxidants. Richards, Ostdal, and Andersen (Citation2002b) studied deoxyhemoglobin-mediated lipid oxidation by comparing the pro-oxidative activity of anodic and cathodic Hb from trout in a washed cod muscle model system. The authors proposed that deoxyhemoglobin was more pro-oxidative than its oxygenated counterpart at pH values found in postmortem fish muscle. Tatiyaborworntham and Richards (Citation2018) and Tatiyaborworntham, Yin, and Richards (Citation2021) studied antioxidant effects of phospholipase A2 (PLA2), an lipolytic enzyme that hydrolyzes phospholipids at the sn-2 position, against the oxidation of trout hemoglobin and lipids in washed cod muscle. In addition, they found that ferrylhemoglobin may be involved in the pro-oxidative activity of trout Hb, which was previously reported (Lee et al. Citation2015). In contrast, Wu et al. (Citation2017) stated that the contribution of ferrylhemoglobin may be negligible in lipid oxidation of washed cod muscles promoted by either porcine or turkey hemolysates.
The effect of FFAs, products of phospholipid hydrolysis by PLA2, on lipid oxidation in muscles was investigated by electron paramagnetic resonance; the spectra results revealed FFA-induced modification of Hb into hemichrome which did not stimulate lipid oxidation in washed turkey muscle during the period of storage. This finding suggested that FFA was able to diminish the lipid oxidation capacity of Hb possibly through formation of hemichrome (Wu et al. Citation2021c). Lee et al. (Citation2015) assessed Mb- and Hb-mediated lipid oxidation in a washed muscle model; key findings suggested that ferri-protoporphyrin IX released from metHb generated free radicals that facilitated both lipid oxidation and degradation of the heme moiety while a crosslinked form of Mb was effective in promoting lipid oxidation. In addition, mechanisms of the inhibitory effect of L-carnosine on lipid oxidation in the presence of oxidized metmyoglobin-mediated washed cod muscle was studied and results showed that the high inhibitory effect of L-carnosine was because of free radical scavenging, metal chelating, and especially its influence on pro-oxidant metmyoglobin structure (Xiao et al. Citation2018).
The washed muscle model is versatile in that a multitude of external factors can be examined pH, pressure, ionic strength, pro-oxidant type, antioxidant type, and storage condition) and internal factors such as type of tissue. GÜner et al. (Citation2020) demonstrated that washed tilapia muscle samples prepared at pH 6.3 were more susceptible to lipid oxidation than samples prepared at pH 6.8. Also, they found that the ability of red onion skin polyphenols to induce a lag phase in lipid oxidation was pH dependent. Using washed muscle of pork, Papuc et al. (Citation2018) showed that the activity of hawthorn berry ethanolic extract (100 mg kg−1) was more greater than butylhydroxyanisole (100 mg kg−1) in diminishing lipid oxidation and protein degradation, for preservation of firmness and lipid stability for six days at 4 °C. Grunwald and Richards (Citation2012) described that pH and NaCl concentration are critical factors that affect the ability of hemopexin to bind ferri-protoporphyrin IX released from metHb and thereby inhibit Hb-mediated lipid oxidation in washed cod muscle. Yang et al. (Citation2018) showed that LOX activity and lipid oxidation were increased under high pressure conditions.
3.7. Muscle homogenates
The overall aim of preparing muscle homogenates is to break up the tissue to generate a uniform system that is compositionally similar to intact muscles. The preparation of muscle homogenates normally consists of homogenization, centrifugation, and clarification. When the system is to be used in lipid oxidation studies, the target muscle is usually homogenized with an extraction buffer (e.g., including KCl, EDTA, and butylated hydroxytoluene) at 4 °C where upon the homogenate is cooled in an ice bath and subsequently maintained at the decided study temperature.
Homogenizing the meat generates small particles and increases interactions between reactants normally contained in different compartments (Bekhit et al. Citation2013; Wang et al. Citation2019), which shortens the lag phase of lipid oxidation and duration of experiments. Min and Ahn (Citation2009) investigated how free ionic iron and Mb affected muscle lipid oxidation in chicken breast and beef homogenates. In addition, Terevinto et al. (Citation2010) investigated factors such as the initial oxidative status, metal content and activities of SOD, CAT, and GPx on lipid oxidation of rhea (Rhea americana) muscle homogenates. Also, ferrous ion-chelating ability, ferric reducing antioxidant power assay, ABTS radical cation-scavenging activity and DPPH radical-scavenging activity of essential oils from Thymus zygis was analyzed in homogenates of minced beef, cooked ham or dry-cured sausage (Ballester-Costa et al. Citation2017). Ahn and Kim (Citation1998) showed that ionic iron-, ferritin-, and Hb-catalyzed oxidation of turkey muscle homogenates were different from those in an oil-based emulsion and cooked-meat homogenate. The changes were ascribed to heat sensitive compounds such as reducing enzymes which contributed to the redox potentials in the muscle homogenates. The investigators found that ferrous iron was the main pro-oxidant among all the iron sources, and, during storage, heme pigments and ferritin did not have a significant catalytic impact on oxidation in the studied homogenates.
Using muscle homogenates, investigators have also assessed the role of storage conditions, pH, chelating agents, salts, temperature, and dietary factors on lipid oxidation. Chen et al. (Citation2021) used duck breast muscle homogenates and revealed that curing by vacuum tumbling decreased the lipid oxidation compared with static brining and pulsed pressure salting. Also, Tongnuanchan, Benjakul, and Prodpran (Citation2011) evaluated the effect of lipid oxidation and pH on the structure and yellow discoloration during storage of a red tilapia (Oreochromis niloticus) muscle homogenate. They found that lipid oxidation significantly stimulated yellow discoloration, mostly by modification of the carbonyl groups involved in the Maillard reaction, and that pH controlled the rate of this reaction. Furthermore, Jin et al. (Citation2012) showed that both temperature and NaCl significantly affected lipid oxidation in a pork belly muscle homogenate. Results indicated that < 5% NaCl (w/w) reduced the activation energy for lipid oxidation and that high temperature as well as moderate concentration of NaCl accelerated lipid oxidation. Moroney et al. (Citation2012) reported that iron-induced lipid oxidation was amplified in liver, heart, kidney, and lung tissue homogenates during 24 h storage 4 °C, while a dietary seaweed extract containing laminarin and fucoidan reduced the degree of lipid oxidation particularly in the liver tissue homogenates. The results confirmed the potential of incorporating marine-derived natural antioxidant in muscle foods via the animal’s diet (Moroney et al. Citation2012). In another similar study, Jeronimo et al. (Citation2020) investigated the influence of Cistus ladanifer L. plant and vegetable oils in the diet for lamb on intramuscular fat composition and muscle antioxidant status. The results indicated that Cistus ladanifer increased α-tocopherol content in the lamb muscle and also limited lipid oxidation in a lamb muscle homogenate during 7 days of storage at 2 °C.
Overall, muscle homogenates are closest to the actual muscle tissue based on composition and structure among all models. This may aid food scientists to obtain more reliable presumptions for muscle oxidation process compared other models. However, the moisture content of muscle homogenates is significantly higher than the actual muscle. In addition, the homogenizing process may result in more exposed cell membranes and destruction of protein structure. These factors could lead to different lipid oxidation features between muscle homogenates and actual muscle.
4. Comparison of model systems
summarizes the main characteristics of the seven muscle models reviewed here, together with their advantages and disadvantages. In general, models that do not include a lipid substrate from actual muscle tissue are easy to prepare and have fewer disturbing factors. For example, lipid monolayer models as emulsions and fatty acid micelles and lipid bilayer models as liposomes are all three simple models to simulate muscle cell membranes (Akbarzadeh et al. Citation2013; Echegaray et al. Citation2021; Laguerre et al. Citation2020). The fact that these models are rapid and easy to prepare as well as to standardize results in universal application, and the results from different researchers can be compared and discussed. Another advantage is that it is relatively easy to monitor the oxidation products and reaction processes in these models. For example, some more advanced analytical techniques, e.g., chemiluminescence, fluorescence emission, Raman spectroscopy, infrared spectroscopy and magnetic resonance are more compatible with these simple models compared with models that include proteins, e.g., microsomes, erythrocytes, washed muscle mince and muscle homogenates (Barriuso, Astiasaran, and Ansorena Citation2013; Laguerre et al. Citation2020). In addition, electron spin resonance and electron paramagnetic resonance can directly be used to monitor the generation of free radicals during oxidation of emulsions, fatty acid micelles, and liposomes (Ikeda et al. Citation2011), but not in the more complex models. Although the three simpler models have many advantages, they do not include any components from actual muscle tissue, which indeed can result in incorrect conclusions when extrapolated results to actual muscle. Yet, these simple models have a value in understanding, e.g., membrane interactions with anti- and pro-oxidants or interfacial phenomena between aqueous/lipid phases in processed muscle products.
Conversely, microsomes, erythrocytes, washed muscle mince, and muscle homogenates have the obvious advantage of containing muscle components and/or muscle structure. For example, microsomes are compositionally similar to muscle cell membranes, while erythrocytes can simulate intact cell membranes. Further, washed muscle mince and muscle homogenates have many structural and compositional elements of actual muscle. These models are therefore appropriate models for lipid oxidation investigations that depend on animal species, muscle type and anatomical location, while more simplistic models cannot be used for these aims.
5. Caution in extrapolating findings from model systems to postmortem muscle tissue
Muscle contains cytosolic, membrane-bound, myofibrillar and stromal proteins as well as trace amounts of carbohydrates, vitamins, and minerals (at less than 1% of muscle wet weight). All these molecules that are to varying degrees excluded or modified in the model systems described in this review. Besides compositional interactions between the different macro- and micro-molecules, structural organization is often overlooked in simple models such as lipid micelles, emulsions, liposomes, and microsomes. Although washed muscle minces are subjected to some physical alteration during their preparation, this model still provides a level of structural organization that cannot be achieved by simply mixing lipid models and myofibrillar components. Using washed muscle mince as study models, studies have related variations in the muscle microstructures to the oxidative stability of lipids (Sannaveerappa, Sandberg, and Undeland Citation2007b; Wu et al. Citation2021b).
Caution must be exercised when comparing lipid models (microsomes, washed muscles, and muscle homogenates) prepared from different sources due to variations in endogenous compounds, e.g., the tocopherol content which should be determined. Increasing concentrations of tocopherols in muscle tissue extends the lag phase prior to formation of lipid oxidation products (Russell et al. Citation2004). Varying the concentrations of endogenous carotenoids in muscle tissue will also influence the onset of lipid oxidation (Jensen et al. Citation1998), which can be an issue, e.g., in salmonoid fish (Salmo gairdnerii gairdnerii). Most of the tocopherols and carotenoids will not be removed by washing with aqueous solutions as they are bound to membranes and to some degree to myofibrillar proteins (carotenoids).
The pH is a very important factor to consider in lipid oxidation model systems and in postmortem muscle tissue. The pH for a model system should be near the pH of the muscle tissue or muscle food product of interest. In a turkey deli meat product, formation of hexanal during refrigerated storage was suppressed 2.4-fold as the pH was increased 0.36 units because of addition of sodium tripolyphosphate (STPP) (Bak, Rankin, and Richards Citation2020). The ability of STPP to inactivate metals and change muscle structure could contribute to the antioxidant effect of STPP in addition to the pH increasing the effect of STPP. Ammonium hydroxide (0.5%) increased pH in raw buffalo patties from 5.6 to 6.4 and decreased formation of lipid oxidation products 3 to 4-fold during 4 °C storage (Naveena et al. Citation2011).
Muscle tissue contains co-factors that facilitate iron-mediated lipid oxidation (e.g., phosphorylated nucleotides and free amino acids) that are often absent in model systems. Nevertheless, co-factors can be added to model systems. For example, Erickson and Hultin (Citation1992) reported the ability of adenosine diphosphate and histidine to activate added iron to instigate lipid oxidation in a sarcoplasmic reticulum preparation. The ability of copper to bind to Mb and thereby increase metmyoglobin formation should be noted in the context of LMW metals increasing the pro-oxidative capacity of heme proteins (Moiseeva and Postnikova Citation2001).
The activity of copper ions can be underestimated when added to a model system if critical endogenous muscle co-factors are excluded. For example, the ability of Cu2+ to decompose linoleic acid hydroperoxide increased greater than three orders of magnitude when a physiological concentration of ascorbate was added to the model system (O’brien Citation1969). This effect was likely because of the ability of ascorbate to facilitate redox cycling of copper between the 2+ and 1+ oxidation state (Khan and Martell Citation1967). The formation of Cu+ may facilitate decomposition of linoleic acid hydroperoxide, in that reduced metals decompose peroxides up to five orders of magnitude more effectively than the higher valence state of the metal (Mcclements and Decker Citation2017).
Soluble components of muscle (e.g., press juices and cytosolic fractions) can be pro-oxidative or antioxidative toward lipids depending on compositional attributes and possibly the type of oxidizable lipid. For example, addition of the low molecular weight (LMW) and high molecular weight (HMW) soluble fractions of ordinary mackerel muscle to liposomes generated maximal lipid oxidation that was partly attributed to the ability of metals in the LMW fraction and heme proteins in the HMW fraction to collectively oxidize the liposomes (Decker and Hultin Citation1990). However, the press juice from, e.g., chicken breast muscle, cod muscle and herring muscle were inhibitory to Hb-mediated lipid oxidation in washed muscle (Li, Richards, and Undeland Citation2005; Sannaveerappa et al. Citation2007a; Undeland, Hultin, and Richards Citation2003). This inhibitor effect can be attributed to the ability of antioxidants in the soluble fraction to inhibit Hb-mediated lipid oxidation that outcompeted the ability of pro-oxidants in the press juice to facilitate lipid oxidation. The greater ability of fish Hbs and Mbs in the soluble fraction to oxidize lipids compared with mammalian and avian heme proteins should be noted (Lee et al. Citation2015). Further, Hb from perch was more oxidative toward lipids than Hb from trout (Aranda et al. Citation2009). And the concentration of heme proteins in cytosol from ordinary muscle (also called light or white muscle) will be lower than that from dark muscle and muscle that is a mixture of light and dark fibers. Heat labile/non-dialyzable and heat resistant/dialyzable factors in the cytosol of white muscle from rainbow trout protected against lipid oxidation in microsomes (Han and Liston Citation1989). Thus, adding the soluble components from different muscles to a selected model system may provide improved understanding of factors that contribute to oxidative stability of the lipids in postmortem muscle.
It should be emphasized that many models are used to increase the speed of oxidation. This is often necessary to observe oxidation before tissues are spoiled by bacterial growth. Many intact meats will spoil from microbial growth before oxidation occurs. Frozen storage or addition of antimicrobials as steptomycin are routes to avoid microbial spoilage however for the former, prolonged time is required to incur oxidation products. Cooked meat will oxidize rapidly so that screening of antioxidants can be examined fairly efficiently after a thermal step with this in mind.
6. Prospects
Technology development always brings expectations to existing challenge. For example, with growing interest in cellular agriculture, the production of meat and fish from stem cell- and tissue-cultures is emerging as an approach to address current ecological challenges (Rubio et al. Citation2019; Tuomisto Citation2018). These technologies may be used also to assess the mechanisms of muscle lipid oxidation. Stem cells are cultivated in a bioreactor under strict control of environmental factors; thus, the endogenous components could be controlled by adjusting the nutrient medium. For example, many investigators reported that Mb is a strong endogenous pro-oxidant in red meat (Faustman et al. Citation2010; Yin, Zhang, and Richards Citation2017; Zhou et al. Citation2016). The addition of Mb into washed muscle is a common approach for this study. However, Mb is located within muscle cells (e.g., myocytes) (Richards Citation2010), which could prevent the interaction between Mb and lipid substrates in situ. Thus, cultured meat or fish could be a good model to identify the activities of important endogenous pro- and antioxidants at the cell scale. In addition, three-dimensional printing stands as a developing technology for food manufacturing (Dick, Bhandari, and Prakash Citation2019) and offers the opportunity to design a new tailored model for lipid oxidation study. This three-dimensional model could be schemed into expected components and muscle structures that meet special needs for lipid oxidation study. The pathways and relationships involved in lipid oxidation are complex in muscle. Existing studies normally just identify affecting factors one-by-one. Simultaneous analysis of multiple factors is a challenge. Mathematical modeling provides a chance to integrate the synthesis of data from many experiments into one system, which could calculate quantitative changes in many factors (Kaczmarek and Muzolf-Panek Citation2022). Thanks to advances in quantitative analysis and computation, chemometrics and machine learning algorithms can be useful for classification, optimization, and prediction in the analysis of complex dataset (Gu et al. Citation2017; Khoshnoudi‐Nia and Moosavi‐Nasab Citation2019; Rosario et al. Citation2020).
7. Conclusion
Muscle foods has complex composition and structures, which explain why the mechanisms and pathways of lipid oxidation are still not completely confirmed. The seven models discussed in this review have been used as tools to aid food scientists getting answers or indications on, e.g., oxidation pathways, oxidation product formation and roles of endogenous pro-/antioxidants or lipid substrates, in indirect ways. It is however vital to distinguish the main characteristics, advantages, and disadvantages of each model to make the right selection. Particularly, it is necessary to pay attention to the following points: 1) feasibility of preparation and analysis of lipid oxidation results; 2) the speed and accuracy of monitoring lipid oxidation products, e.g., tentative interference from model system components; 3) cost of preparing the desired model and related analyses; 4) stability and robustness of the model, i.e., the possibility to get reproducible results across different experiments. Based on this reviewing of oxidation models, tailoring correct model to different study aims could be facilitated, and readers are becoming acquainted with advantages and shortcomings. In addition, insight into recent technology developments, e.g., stem cell- and tissue-cultures as well as three-dimensional printing could provide new opportunities to overcome the current bottlenecks of lipid oxidation studies in muscle.
Acknowledgments
Cartoons in were created with BioRender.com.
Conflicts of interest
The authors declare no conflict of interest.
Additional information
Funding
References
- Abraham, A., K. B. Bjugstad, G. G. Mafi, D. L. Vanoverbeke, C. Gifford, L. T. Rael, R. Bar-Or, and R. Ramanathan. 2016. Correlating myoglobin and lipid oxidation with reduction potential in a sarcoplasm-liposome system. Meat Science 112:172. doi: 10.1016/j.meatsci.2015.08.161.
- Ahmmed, M. K., F. Ahmmed, I. Stewart, A. Carne, H. S. Tian, and A. E. A. Bekhit. 2021. Omega-3 phospholipids in pacific blue mackerel (Scomber australasicus) processing by-products. Food Chemistry 353:129451. doi: 10.1016/j.foodchem.2021.129451.
- Ahn, D. U., and S. M. Kim. 1998. Prooxidant effects of ferrous iron, hemoglobin, and ferritin in oil emulsion and cooked-meat homogenates are different from those in raw-meat homogenates. Poultry Science 77 (2):348–55. doi: 10.1093/ps/77.2.348.
- Akbarzadeh, A., R. Rezaei-Sadabady, S. Davaran, S. W. Joo, N. Zarghami, Y. Hanifehpour, M. Samiei, M. Kouhi, and K. Nejati-Koshki. 2013. Liposome: Classification, preparation, and applications. Nanoscale Research Letters 8 (1):102. doi: 10.1186/1556-276X-8-102.
- Alberts, B., A. Johnson, J. Lewis, M. Raff, K. Roberts, and P. Walter. 2002. Molecular biology of the cell. In Molecular biology of the cell. New York: Garland Science.
- Alvarado, C. Z., M. P. Richards, S. F. O’Keefe, and H. Wang. 2007. The effect of blood removal on oxidation and shelf life of broiler breast meat. Poultry Science 86 (1):156–61. doi: 10.1093/ps/86.1.156.
- Amaral, A. B., M. V. Da Silva, and S. C. D. Lannes. 2018. Lipid oxidation in meat: Mechanisms and protective factors – a review. Food Science and Technology 38 (suppl 1):1–15. doi: 10.1590/fst.32518.
- Aranda, R., H. Cai, C. E. Worley, E. J. Levin, R. Li, J. S. Olson, G. N. Phillips, and M. P. Richards. 2009. Structural analysis of fish versus mammalian hemoglobins: Effect of the heme pocket environment on autooxidation and hemin loss. Proteins: Structure, Function, and Bioinformatics 75 (1):217–30. doi: 10.1002/prot.22236.
- Bak, K. H., S. A. Rankin, and M. P. Richards. 2020. Hexanal as a marker of oxidation flavour in sliced and uncured deli turkey with and without phosphates using rosemary extracts. International Journal of Food Science & Technology 55 (9):3104–10. doi: 10.1111/ijfs.14574.
- Ballester-Costa, C., E. Sendra, J. Fernandez-Lopez, J. A. Perez-Alvarez, and M. Viuda-Martos. 2017. Assessment of antioxidant and antibacterial properties on meat homogenates of essential oils obtained from four thymus species achieved from organic growth. Foods 6 (8):59. doi: 10.3390/foods6080059.
- Baron, C. P., L. H. Skibsted, and H. J. Andersen. 2000. Peroxidation of linoleate at physiological ph: Hemichrome formation by substrate binding protects against metmyoglobin activation by hydrogen peroxide. Free Radical Biology & Medicine 28 (4):549–58. doi: Doi doi: 10.1016/S0891-5849(99)00240-3.
- Barriuso, B., I. Astiasaran, and D. Ansorena. 2013. A review of analytical methods measuring lipid oxidation status in foods: A challenging task. European Food Research and Technology 236 (1):1–15. doi: 10.1007/s00217-012-1866-9.
- Bekhit, A. E. A., D. L. Hopkins, F. T. Fahri, and E. N. Ponnampalam. 2013. Oxidative processes in muscle systems and fresh meat: Sources, markers, and remedies. Comprehensive Reviews in Food Science and Food Safety 12 (5):565–97. doi: 10.1111/1541-4337.12027.
- Bielski, B. H., D. E. Cabelli, R. L. Arudi, and A. B. Ross. 1985. Reactivity of ho2/o − 2 radicals in aqueous solution. Journal of Physical and Chemical Reference Data 14 (4):1041–100. doi: 10.1063/1.555739.
- Bieri, J. G, and A. A. Anderson. 1960. Peroxidation of lipids in tissue homogenates as related to vitamin-e. Archives of Biochemistry and Biophysics 90 (1):105–10. doi: 10.1016/0003-9861(60)90619-6.
- Boveris, A. D., A. Galatro, and S. Puntarulo. 2000. Effect of nitric oxide and plant antioxidants on microsomal content of lipid radicals. Biological Research 33 (2):159–65. doi: 10.4067/s0716-97602000000200016.
- Burri, S. C. M., A. Ekholm, U. Bleive, T. Pussa, M. Jensen, J. Hellstrom, S. Makinen, R. Korpinen, P. H. Mattila, V. Radenkovs, et al. 2020. Lipid oxidation inhibition capacity of plant extracts and powders in a processed meat model system. Meat Science 162:108033. doi: 10.1016/j.meatsci.2019.108033.
- Cai, H., E. W. Grunwald, S. Y. Park, B. F. Lei, and M. P. Richards. 2013. Lipid oxidation in trout muscle is strongly inhibited by a protein that specifically binds hemin released from hemoglobin. Journal of Agricultural and Food Chemistry 61 (17):4180–7. doi: 10.1021/jf4006142.
- Carvajal, A. K., T. Rustad, R. Mozuraityte, and I. Storro. 2009. Kinetic studies of lipid oxidation induced by hemoglobin measured by consumption of dissolved oxygen in a liposome model system. Journal of Agricultural and Food Chemistry 57 (17):7826–33. doi: 10.1021/jf9013394.
- Chaijan, M, and W. Panpipat. 2017. Mechanism of oxidation in foods of animal origin. In Natural antioxidants, 21–58: Apple Academic Press.
- Chan, W. K. M., C. Faustman, M. Yin, and E. A. Decker. 1997. Lipid oxidation induced by oxymyoglobin and metmyoglobin with involvement of H2O2 and superoxide anion. Meat Science 46 (2):181–90. doi: 10.1016/S0309-1740(97)00014-4.
- Chen, X., J. Luo, A. Lou, Y. Wang, D. Yang, and Q. W. Shen. 2021. Duck breast muscle proteins, free fatty acids and volatile compounds as affected by curing methods. Food Chemistry 338:128138. doi: 10.1016/j.foodchem.2020.128138.
- Chen, X., M. H. Wu, Q. Yang, and S. Y. Wang. 2017. Preparation, characterization of food grade phycobiliproteins from Porphyra haitanensis and the application in liposome-meat system. Lwt 77:468–74. doi: 10.1016/j.lwt.2016.12.005.
- Chéret, R., N. Chapleau, C. Delbarre‐Ladrat, V. Verrez‐Bagnis, and M. D. Lamballerie. 2005. Effects of high pressure on texture and microstructure of sea bass (Dicentrarchus labrax L.) fillets. Journal of Food Science 70 (8):e477–e83. 8doi: 10.1111/j.1365-2621.2005.tb11518.x.
- Chinnagounder Periyasamy, P., J. C. H. Leijten, P. J. Dijkstra, M. Karperien, and J. N. Post. 2012. Nanomaterials for the local and targeted delivery of osteoarthritis drugs. Journal of Nanomaterials 2012:1–13. doi: 10.1155/2012/673968.
- Cimen, M. Y. 2008. Free radical metabolism in human erythrocytes. Clinica Chimica Acta; International Journal of Clinical Chemistry 390 (1–2):1–11. doi: 10.1016/j.cca.2007.12.025.
- Cui, L, and E. A. Decker. 2016. Phospholipids in foods: Prooxidants or antioxidants? Journal of the Science of Food and Agriculture 96 (1):18–31. doi: 10.1002/jsfa.7320.
- Cui, L., J. Fan, Y. Sun, Z. Zhu, and J. Yi. 2018. The prooxidant activity of salts on the lipid oxidation of lecithin-stabilized oil-in-water emulsions. Food Chemistry 252:28–32. doi: 10.1016/j.foodchem.2018.01.094.
- Culbertson, S. M, and N. A. Porter. 2000. Unsymmetrical azo initiators increase efficiency of radical generation in aqueous dispersions, liposomal membranes, and lipoproteins. Journal of the American Chemical Society 122 (17):4032–8. doi: 10.1021/ja9934605.
- Dacaranhe, C. D, and J. Terao. 2001. A unique antioxidant activity of phosphatidylserine on iron-induced lipid peroxidation of phospholipid bilayers. Lipids 36 (10):1105–10. doi: 10.1007/s11745-001-0820-7.
- Davidovic-Plavsic, B., B. Kukavica, S. Skondric, C. Jimenez-Gallardo, and M. Zabic. 2021. Wild garlic extract reduces lipid peroxidation in terbuthylazine-treated human erythrocytes. Biomarkers 26 (7):617–24. doi: 10.1080/1354750X.2021.1953598.
- Decker, E. A., C. H. Huang, J. E. Osinchak, and H. O. Hultin. 1989. Iron and copper: Role in enzymic lipid oxidation of fish sarcoplasmic reticulum at in situ concentrations. Journal of Food Biochemistry 13 (3):179–86. doi: 10.1111/j.1745-4514.1989.tb00392.x.
- Decker, E. A, and H. O. Hultin. 1990. Factors influencing catalysis of lipid oxidation by the soluble fraction of mackerel muscle. Journal of Food Science 55 (4):947–50. doi: 10.1111/j.1365-2621.1990.tb01571.x.
- Dick, A., B. Bhandari, and S. Prakash. 2019. 3d printing of meat. Meat Science 153:35–44. doi: 10.1016/j.meatsci.2019.03.005.
- Dominguez, R., M. Pateiro, M. Gagaoua, F. J. Barba, W. Zhang, and J. M. Lorenzo. 2019. A comprehensive review on lipid oxidation in meat and meat products. Antioxidants 8 (10):429. doi: 10.3390/antiox8100429.
- Echegaray, N., M. Pateiro, P. E. S. Munekata, J. M. Lorenzo, Z. Chabani, M. A. Farag, and R. Dominguez. 2021. Measurement of antioxidant capacity of meat and meat products: Methods and applications. Molecules 26 (13):3880. doi: 10.3390/molecules26133880.
- Erickson, M. C. 2008. Lipid oxidation of muscle foods. In Food lipids: Chemistry, nutrition, and biotechnology, 321–64. Boca Raton, FL: CRC Press. doi: 10.1201/9781420046649.
- Erickson, M. C, and H. O. Hultin. 1992. Influence of histidine on lipid-peroxidation in sarcoplasmic-reticulum. Archives of Biochemistry and Biophysics 292 (2):427–32. doi: 10.1016/0003-9861(92)90012-L.
- Faustman, C., Q. Sun, R. Mancini, and S. P. Suman. 2010. Myoglobin and lipid oxidation interactions: Mechanistic bases and control. Meat Science 86 (1):86–94. doi: 10.1016/j.meatsci.2010.04.025.
- Folkes, L. K., L. P. Candeias, and P. Wardman. 1995. Kinetics and mechanisms of hypochlorous acid reactions. Archives of Biochemistry and Biophysics 323 (1):120–6. doi: 10.1006/abbi.1995.0017.
- Frenzel, M, and A. Steffen-Heins. 2015. Impact of quercetin and fish oil encapsulation on bilayer membrane and oxidation stability of liposomes. Food Chemistry 185:48–57. doi: 10.1016/j.foodchem.2015.03.121.
- Gal, S., I. Pinchuk, and D. Lichtenberg. 2003. Peroxidation of liposomal palmitoyllinoleoylphosphatidylcholine (PLPC), effects of surface charge on the oxidizability and on the potency of antioxidants. Chemistry and Physics of Lipids 126 (1):95–110. doi: 10.1016/S0009-3084(03)00096-3.
- Genot, C., M. Metro, A. Viau, G. Meynier, and G. Gandemer. 1992. How to prepared liposomes to study oxidation of muscle phospholipids? In 38th International Congress of Meat Science and Technology. Clermont Ferrand, France.
- Ghirmai, S., L. Eriksson, H. Wu, M. Axelsson, and I. Undeland. 2020. Improving the stability of red blood cells in rainbow trout (Oncorhynchus mykiss) and herring (Clupea harengus): Potential solutions for post-mortem fish handling to minimize lipid oxidation. Food and Bioprocess Technology 13 (8):1344–55. doi: 10.1007/s11947-020-02472-3.
- Greenberg, M. E., X. M. Li, B. G. Gugiu, X. Gu, J. Qin, R. G. Salomon, and S. L. Hazen. 2008. The lipid whisker model of the structure of oxidized cell membranes. The Journal of Biological Chemistry 283 (4):2385–96. doi: 10.1074/jbc.M707348200.
- Grunwald, E. W, and M. P. Richards. 2012. Effects of hemopexin on hemin and hemoglobin-mediated lipid oxidation in washed fish muscle. LWT – Food Science and Technology 46 (2):412–8. doi: 10.1016/j.lwt.2011.12.007.
- Gu, X., Y. Sun, K. Tu, and L. Pan. 2017. Evaluation of lipid oxidation of chinese-style sausage during processing and storage based on electronic nose. Meat Science 133:1–9. doi: 10.1016/j.meatsci.2017.05.017.
- Guengerich, F. P. 1977. Separation and purification of multiple forms of microsomal cytochrome-p-450 – activities of different forms of cytochrome-p-450 towards several compounds of environmental interest. The Journal of Biological Chemistry 252 (11):3970–9. doi: 10.1016/S0021-9258(17)40345-0.
- Guldiken, B., M. Gibis, D. Boyacioglu, E. Capanoglu, and J. Weiss. 2018. Physical and chemical stability of anthocyanin-rich black carrot extract-loaded liposomes during storage. Food Research International 108:491–7. doi: 10.1016/j.foodres.2018.03.071.
- GÜner, S., Y. YaĞiz, Z. Topalcengİz, H. G. Kristinsson, G. Baker, P. Sarnoski, B. A. Welt, A. Simonne, and M. R. Marshall. 2020. Effect of ph on lipid oxidation of red onion skin extracts treated with washed tilapia (Oreochromis niloticus) muscle model systems. Turkish Journal of Chemistry 44 (6):1528–38. doi: 10.3906/kim-2004-47.
- Hajimohammadi, M. 2020. Investigation of antioxidant activity of cumin (Cuminum cyminum L.) by means of UV-vis spectroscopy, proton nuclear magnetic resonance and iodometric method. Biomedical Journal of Scientific & Technical Research 27 (3):20713–9. doi: 10.26717/BJSTR.2020.27.004491.
- Hajimohammadi, M, and M. Bagheri. 2017. Comparison of the antioxidant activity of Echium Amoenum Fisch and ca Mey, Chamaemelum nobile (L.) all and Camellia sinensis (L.) o. Kuntze on oleic acid photooxidation using water soluble porphyrin complexes as catalyst. Innovative Food Technologies 4, no 4:57–66. doi: 10.22104/JIFT.2017.483.
- Hajimohammadi, M, and P. Nosrati. 2018. Scavenging effect of pasipay (Passiflora incarnate l.) on singlet oxygen generation and fatty acid photooxygenation. Food Science & Nutrition 6 (6):1670–5. doi: 10.1002/fsn3.731.
- Halldorsdottir, S. M., H. G. Kristinsson, H. Sveinsdottir, G. Thorkelsson, and P. Y. Hamaguchi. 2013. The effect of natural antioxidants on haemoglobin-mediated lipid oxidation during enzymatic hydrolysis of cod protein. Food Chemistry 141 (2):914–9. doi: 10.1016/j.foodchem.2013.03.101.
- Han, T. J, and J. Liston. 1989. Lipid-peroxidation protection factors in rainbow-trout (salmo-gairdnerii) muscle cytosol. Journal of Food Science 54 (4):809–13. doi: 10.1111/j.1365-2621.1989.tb07888.x.
- Hauville, C., S. Remita, P. Therond, A. Rouscilles, M. Couturier, D. Jore, and M. Gardes-Albert. 1998. Determination of the yield of radiation-induced peroxidation of sodium linoleate in aqueous monomeric and micellar solutions. Radiation Research 150 (5):600–8. doi: 10.2307/3579878.
- Heden, T. D., P. D. Neufer, and K. Funai. 2016. Looking beyond structure: Membrane phospholipids of skeletal muscle mitochondria. Trends in Endocrinology and Metabolism: TEM 27 (8):553–62. doi: 10.1016/j.tem.2016.05.007.
- Hosoya, T., S. Matsukawa, and Y. Nagai. 1971. Localization of peroxidase and other microsomal enzymes in thyroid cells. Biochemistry 10 (16):3086–93. doi: 10.1021/bi00792a016.
- Hu, M., E. A. Decker, and D. J. Mcclements. 2019. Emulsion technologies to produce oxidative stable emulsions containing n-3 fatty acids. In Healthful lipids, 547–557. New York, USA: AOCS Publishing.
- Hua-Tao, L., L. Lei, Z. Rong-Mei, L. Lan, Y. Zhi, Z. Shan-Fu, J. Jun, L. Si-Miao, D. Ting-Ting, L. Qi, et al. 2019. The extracts of Angelica sinensis inhibit lipid oxidation in fish erythrocytes and improve growth, digestive, absorptive and antioxidant capacity in Juvenile jian carp (Cyprinus carpio var. Jian). Aquaculture Nutrition 25 (1):119–33. doi: 10.1111/anu.12836.
- Huang, C. H, and H. O. Hultin. 1992. Soluble and bound iron equally effect lipid oxidation of sarcoplasmic-reticulum. Journal of Food Biochemistry 16 (1):1–13. doi: 10.1111/j.1745-4514.1992.tb00429.x.
- Hui, Y. H. 2006. Handbook of food science, technology, and engineering. Vol. 149. New York, USA: CRC Press.
- Ikeda, H., Y. Kimura, M. Masaki, and H. Iwahashi. 2011. Caffeic acid inhibits the formation of 1-hydroxyethyl radical in the reaction mixture of rat liver microsomes with ethanol partly through its metal chelating activity. Journal of Clinical Biochemistry and Nutrition 48 (3):187–93. doi: 10.3164/jcbn.10-45.
- Inchingolo, R., I. Bayram, S. Uluata, S. S. Kiralan, M. T. Rodriguez-Estrada, D. J. Mcclements, and E. A. Decker. 2021. Ability of sodium dodecyl sulfate (sds) micelles to increase the antioxidant activity of alpha-tocopherol. Journal of Agricultural and Food Chemistry 69 (20):5702–8. doi: 10.1021/acs.jafc.1c01199.
- Jakobsson, P. J., J. A. Mancini, D. Riendeau, and A. W. Ford-Hutchinson. 1997. Identification and characterization of a novel microsomal enzyme with glutathione-dependent transferase and peroxidase activities. The Journal of Biological Chemistry 272 (36):22934–9. doi: 10.1074/jbc.272.36.22934.
- Jensen, C., E. Birk, A. Jokumsen, L. H. Skibsted, and G. Bertelsen. 1998. Effect of dietary levels of fat, alpha-tocopherol and astaxanthin on colour and lipid oxidation during storage of frozen rainbow trout (oncorhynchus mykiss) and during chill storage of smoked trout. Zeitschrift für Lebensmitteluntersuchung und -Forschung A 207 (3):189–96. doi: 10.1007/s002170050317.
- Jeronimo, E., D. Soldado, S. Sengo, A. Francisco, F. Fernandes, A. P. V. Portugal, S. P. Alves, J. Santos-Silva, and R. J. B. Bessa. 2020. Increasing the alpha-tocopherol content and lipid oxidative stability of meat through dietary Cistus ladanifer L. In lamb fed increasing levels of polyunsaturated fatty acid rich vegetable oils. Meat Science 164:108092. doi: 10.1016/j.meatsci.2020.108092.
- Jha, R, and S. I. Rizvi. 2009. Age-dependent decline in erythrocyte acetylcholinesterase activity: Correlation with oxidative stress. Biomedical Papers of the Medical Faculty of the University Palacky, Olomouc, Czechoslovakia 153 (3):195–8. doi: 10.5507/bp.2009.032.
- Jiang, J, and Y. L. Xiong. 2015. Role of interfacial protein membrane in oxidative stability of vegetable oil substitution emulsions applicable to nutritionally modified sausage. Meat Science 109:56–65. doi: 10.1016/j.meatsci.2015.05.011.
- Jin, G. F., L. C. He, J. H. Zhang, X. Yu, J. M. Wang, and F. Huang. 2012. Effects of temperature and nacl percentage on lipid oxidation in pork muscle and exploration of the controlling method using response surface methodology (RSM). Food Chemistry 131 (3):817–25. doi: 10.1016/j.foodchem.2011.09.050.
- Johnson, D. R., R. Inchingolo, and E. A. Decker. 2018. The ability of oxygen scavenging packaging to inhibit vitamin degradation and lipid oxidation in fish oil-in-water emulsions. Innovative Food Science & Emerging Technologies 47:467–75. doi: 10.1016/j.ifset.2018.04.021.
- Junqueira, H., A. P. Schroder, F. Thalmann, A. Klymchenko, Y. Mely, M. S. Baptista, and C. M. Marques. 2021. Molecular organization in hydroperoxidized popc bilayers. Biochimica et Biophysica Acta. Biomembranes 1863 (10):183659. doi: 10.1016/j.bbamem.2021.183659.
- Kaczmarek, A. M, and M. Muzolf-Panek. 2022. Predictive modelling of tbars changes in the intramuscular lipid fraction of raw ground pork enriched with plant extracts. Journal of Food Science and Technology 59 (5):1756–68. doi: 10.1007/s13197-021-05187-1.
- Karabulut, I., Z. D. Balkanci, B. Pehlivanoglu, A. Erdem, and E. Fadillioglu. 2009. Effect of toluene on erythrocyte membrane stability under in vivo and in vitro conditions with assessment of oxidant/antioxidant status. Toxicology and Industrial Health 25 (8):545–50. doi: 10.1177/0748233709346758.
- Karayannakidis, P. D., A. Zotos, D. Petridis, and K. D. A. Taylor. 2008. The effect of washing, microbial transglutaminase, salts and starch addition on the functional properties of sardine (Sardina pilchardus) kamaboko gels. Food Science and Technology International 14 (2):167–77. doi: 10.1177/1082013208092816.
- Kathirvel, P, and M. P. Richards. 2012. Effect of a membrane permeable metal chelator on iron and hemoglobin-mediated lipid oxidation in washed fish muscle. Food Research International 48 (2):346–52. doi: 10.1016/j.foodres.2012.04.016.
- Kaurinovic, B, and M. Popovic. 2012. Liposomes as a tool to study lipid peroxidation. In Lipid peroxidation, ed. A. Catala. London: IntechOpen. doi: 10.5772/46020.
- Khan, M. M, and A. E. Martell. 1967. Metal ion and metal chelate catalyzed oxidation of ascorbic acid by molecular oxygen. Ii. Cupric and ferric chelate catalyzed oxidation. Journal of the American Chemical Society 89 (26):7104–11. doi: 10.1021/ja01002a046.
- Khoshnoudi‐Nia, S, and M. Moosavi‐Nasab. 2019. Comparison of various chemometric analysis for rapid prediction of thiobarbituric acid reactive substances in rainbow trout fillets by hyperspectral imaging technique. Food Science & Nutrition 7 (5):1875–83. doi: 10.1002/fsn3.1043.
- Kleszczyfiska, H., D. Bonarska, M. Oswiecimska, and J. Sarapuk. 2003. Hemolysis and antioxidative protection of erythrocytes by functionalized quaternary ammonium salts. Polish Journal of Environmental Studies 12 (1):63–66.
- Kunyaboon, S., K. Thumanu, J. W. Park, C. Khongla, and J. Yongsawatdigul. 2021. Evaluation of lipid oxidation, volatile compounds and vibrational spectroscopy of silver carp (Hypophthalmichthys molitrix) during ice storage as related to the quality of its washed mince. Foods 10 (3):495. doi: 10.3390/foods10030495.
- Labuza, T. P, and L. Dugan. Jr. 1971. Kinetics of lipid oxidation in foods. Critical Reviews in Food Science & Nutrition 2:355–405.
- Laguerre, M., M. Tenon, A. Bily, and S. Birtić. 2020. Toward a spatiotemporal model of oxidation in lipid dispersions: A hypothesis‐driven review. European Journal of Lipid Science and Technology 122 (3):1900209. doi: 10.1002/ejlt.201900209.
- Lee, S. K., N. Tatiyaborworntham, E. W. Grunwald, and M. P. Richards. 2015. Myoglobin and haemoglobin-mediated lipid oxidation in washed muscle: Observations on crosslinking, ferryl formation, porphyrin degradation, and haemin loss rate. Food Chemistry 167:258–63. doi: 10.1016/j.foodchem.2014.06.098.
- Lee, S. Y., D. Y. Lee, O. Y. Kim, H. J. Kang, H. S. Kim, and S. J. Hur. 2020. Overview of studies on the use of natural antioxidative materials in meat products. Food Science of Animal Resources 40 (6):863–80. doi: 10.5851/kosfa.2020.e84.
- Lee, Y. B., G. L. Hargus, J. A. Kirkpatrick, D. L. Berner, and R. H. Forsythe. 1975. Mechanism of lipid oxidation in mechanically deboned chicken meat. Journal of Food Science 40 (5):964–7. doi: 10.1111/j.1365-2621.1975.tb02244.x.
- Li, H, and G. Lykotrafitis. 2014. Erythrocyte membrane model with explicit description of the lipid bilayer and the spectrin network. Biophysical Journal 107 (3):642–53. doi: 10.1016/j.bpj.2014.06.031.
- Li, H., X. Zhou, P. Gao, Q. Li, H. Li, R. Huang, and M. Wu. 2016. Inhibition of lipid oxidation in foods and feeds and hydroxyl radical-treated fish erythrocytes: A comparative study of Ginkgo biloba leaves extracts and synthetic antioxidants. Animal Nutrition (Zhongguo xu mu Shou yi Xue Hui) 2 (3):234–41. doi: 10.1016/j.aninu.2016.04.007.
- Li, H. T., L. Feng, W. D. Jiang, Y. Liu, J. Jiang, S. H. Li, and X. Q. Zhou. 2013. Oxidative stress parameters and anti-apoptotic response to hydroxyl radicals in fish erythrocytes: Protective effects of glutamine, alanine, citrulline and proline. Aquatic Toxicology (Amsterdam, Netherlands) 126:169–79. doi: 10.1016/j.aquatox.2012.11.005.
- Li, Q. T., M. H. Yeo, and B. K. Tan. 2000. Lipid peroxidation in small and large phospholipid unilamellar vesicles induced by water-soluble free radical sources. Biochemical and Biophysical Research Communications 273 (1):72–6. doi: 10.1006/bbrc.2000.2908.
- Li, R., M. P. Richards, and I. Undeland. 2005. Characterization of aqueous components in chicken breast muscle as inhibitors of hemoglobin-mediated lipid oxidation. Journal of Agricultural and Food Chemistry 53 (3):767–75. doi: 10.1021/jf049515q.
- Lin, T. S, and H. O. Hultin. 1976. Enzymic lipid peroxidation in microsomes of chicken skeletal-muscle. Journal of Food Science 41 (6):1488–9. doi: 10.1111/j.1365-2621.1976.tb01203.x.
- Litvinko, N. M., L. A. Skorostetskaya, and D. O. Gerlovsky. 2018. The interaction of phospholipase a2 with oxidized phospholipids at the lipid-water surface with different structural organization. Chemistry and Physics of Lipids 211:44–51. doi: 10.1016/j.chemphyslip.2017.10.010.
- Liu, H.-P. 1970. Catalysts of lipid peroxidation in meats. 1. Linoleate peroxidation catalyzed by metmb or fe (ii)‐edta. Journal of Food Science 35:591–92.
- Lynch, M. P, and C. Faustman. 2000. Effect of aldehyde lipid oxidation products on myoglobin. Journal of Agricultural and Food Chemistry 48 (3):600–4. doi: 10.1021/jf990732e.
- Mcclements, D., S. Dungan, J. German, and J. Kinsella. 1992. Oil exchange between oil-in-water emulsion droplets stabilised with a non-ionic surfactant. Food Hydrocolloids. 6 (5):415–22. doi: 10.1016/S0268-005X(09)80027-1.
- Mcclements, D. J, and E. A. Decker. 2000. Lipid oxidation in oil-in-water emulsions: Impact of molecular environment on chemical reactions in heterogeneous food systems. Journal of Food Science 65 (8):1270–82. doi: 10.1111/j.1365-2621.2000.tb10596.x.
- Mcclements, D. J, and E. A. Decker. 2017. Lipids. In Fennema’s food chemistry eds. S. Damodaran, and K. L. Parkin, 171–233. New York, USA: CRC Press.
- Mcmurray, W, and T. L. Dormandy. 1974. Lipid autoxidation in human skeletal muscle. Clinica Chimica Acta 52 (1):105–14. doi: 10.1016/0009-8981(74)90393-3.
- Mcnulty, H. P., J. Byun, S. F. Lockwood, R. F. Jacob, and R. P. Mason. 2007. Differential effects of carotenoids on lipid peroxidation due to membrane interactions: X-ray diffraction analysis. Biochimica et Biophysica Acta 1768 (1):167–74. doi: 10.1016/j.bbamem.2006.09.010.
- Medina, I., I. Undeland, K. Larsson, I. Storro, T. Rustad, C. Jacobsen, V. Kristinova, and J. M. Gallardo. 2012. Activity of caffeic acid in different fish lipid matrices: A review. Food Chemistry 131 (3):730–40. doi: 10.1016/j.foodchem.2011.09.032.
- Mekhloufi, J., D. Bonnefont-Rousselot, S. Yous, D. Lesieur, M. Couturier, P. Therond, A. Legrand, D. Jore, and M. Gardes-Albert. 2005. Antioxidant activity of melatonin and a pinoline derivative on linoleate model system. Journal of Pineal Research 39 (1):27–33. doi: 10.1111/j.1600-079X.2005.00208.x.
- Mercier, Y., P. Gatellier, M. Viau, H. Remignon, and M. Renerre. 1998. Effect of dietary fat and vitamin e on colour stability and on lipid and protein oxidation in turkey meat during storage. Meat Science 48 (3–4):301–18. doi: 10.1016/S0309-1740(97)00113-7.
- Min, B, and D. U. Ahn. 2005. Mechanism of lipid peroxidation in meat and meat products – a review. Food Science and Biotechnology 14 (1):152–63.
- Min, B, and D. U. Ahn. 2009. Factors in various fractions of meat homogenates that affect the oxidative stability of raw chicken breast and beef loin. Journal of Food Science 74 (1):C41–C48. doi: 10.1111/j.1750-3841.2008.01003.x.
- Min, B., K. C. Nam, and D. U. Ahn. 2010. Catalytic mechanisms of metmyoglobin on the oxidation of lipids in phospholipid liposome model system. Food Chemistry 123 (2):231–6. doi: 10.1016/j.foodchem.2010.04.013.
- Min, B., K. C. Nam, J. Cordray, and D. U. Ahn. 2008. Endogenous factors affecting oxidative stability of beef loin, pork loin, and chicken breast and thigh meats. Journal of Food Science 73 (6):C439–46. doi: 10.1111/j.1750-3841.2008.00805.x.
- Mitra, A. K., K. Cholkar, and A. Mandal. 2017. Emerging nanotechnologies for diagnostics, drug delivery and medical devices. Amsterdam, Netherlands: William Andrew.
- Mohajeri, E, and G. D. Noudeh. 2012. Effect of temperature on the critical micelle concentration and micellization thermodynamic of nonionic surfactants: Polyoxyethylene sorbitan fatty acid esters. E-Journal of Chemistry 9 (4):2268–74. doi: 10.1155/2012/961739.
- Moiseeva, S. A, and G. B. Postnikova. 2001. Mechanism of oxidation of oxymyoglobin by copper ions: Comparison of sperm whale, horse, and pig myoglobins. Biochemistry. Biokhimiia 66 (7):780–7. doi: 10.1023/a:1010268813926.
- Moroney, N. C., M. N. O’grady, J. V. O’doherty, and J. P. Kerry. 2012. Addition of seaweed (Laminaria digitata) extracts containing laminarin and fucoidan to porcine diets: Influence on the quality and shelf-life of fresh pork. Meat Science 92 (4):423–9. doi: 10.1016/j.meatsci.2012.05.005.
- Mosca, M., A. Ceglie, and L. Ambrosone. 2011. Effect of membrane composition on lipid oxidation in liposomes. Chemistry and Physics of Lipids 164 (2):158–65. doi: 10.1016/j.chemphyslip.2010.12.006.
- Mozuraityte, R., T. Rustad, and I. Storr⊘. 2006. Oxidation of cod phospholipids in liposomes: Effects of salts, ph and zeta potential. European Journal of Lipid Science and Technology 108 (11):944–50. doi: 10.1002/ejlt.200600139.
- Naveena, B. M., A. R. Sen, M. Muthukumar, Y. Babji, and N. Kondaiah. 2011. Effects of salt and ammonium hydroxide on the quality of ground buffalo meat. Meat Science 87 (4):315–20. doi: 10.1016/j.meatsci.2010.11.004.
- Nieto, G., K. Huvaere, and L. H. Skibsted. 2011. Antioxidant activity of rosemary and thyme by-products and synergism with added antioxidant in a liposome system. European Food Research and Technology 233 (1):11–8. doi: 10.1007/s00217-011-1486-9.
- Niki, E. 2009. Lipid peroxidation: Physiological levels and dual biological effects. Free Radical Biology & Medicine 47 (5):469–84. doi: 10.1016/j.freeradbiomed.2009.05.032.
- Nuchi, C. D., P. Hernandez, D. J. Mcclements, and E. A. Decker. 2002. Ability of lipid hydroperoxides to partition into surfactant micelles and alter lipid oxidation rates in emulsions. Journal of Agricultural and Food Chemistry 50 (19):5445–9. doi: 10.1021/jf020095j.
- O’brien, P. J. 1969. Intracellular mechanisms for the decomposition of a lipid peroxide. I. Decomposition of a lipid peroxide by metal ions, heme compounds, and nucleophiles. Canadian Journal of Biochemistry 47 (5):485–92. doi: 10.1139/o69-076.
- Pandey, K. B., N. Mishra, and S. I. Rizvi. 2009. Myricetin may provide protection against oxidative stress in type 2 diabetic erythrocytes. Zeitschrift Für Naturforschung C 64 (9–10):626–30. doi: 10.1515/znc-2009-9-1004.
- Papuc, C., C. N. Predescu, L. Tudoreanu, V. Nicorescu, and I. Gâjâilă. 2018. Comparative study of the influence of hawthorn (Crataegus monogyna) berry ethanolic extract and butylated hydroxylanisole (bha) on lipid peroxidation, myoglobin oxidation, consistency and firmness of minced pork during refrigeration. Journal of the Science of Food and Agriculture 98 (4):1346–61. doi: 10.1002/jsfa.8599.
- Pazos, M., S. Lois, J. L. Torres, and I. Medina. 2006. Inhibition of hemoglobin- and iron-promoted oxidation in fish microsomes by natural phenolics. Journal of Agricultural and Food Chemistry 54 (12):4417–23. doi: 10.1021/jf0530300.
- Pazos, M., I. Medina, and H. O. Hultin. 2005. Effect of ph on hemoglobin-catalyzed lipid oxidation in cod muscle membranes in vitro and in situ. Journal of Agricultural and Food Chemistry 53 (9):3605–12. doi: 10.1021/jf0403890.
- Pereira, A. L. F, and V. K. G. Abreu. 2018. Lipid peroxidation in meat and meat products. In Lipid peroxidation research, 29. London: IntechOpen.
- Perera, M. I. R., A. J. Demetris, S. L. Katyal, and H. Shinozuka. 1985. Lipid peroxidation of liver microsome membranes induced by cholinedeficient diets and its relationship to the diet-induced promotion of the induction of γ-glutamyltranspeptidase-positive foci. Cancer Research 45 (6):2533–8.
- Perez, D. M., M. P. Richards, R. S. Parker, M. E. Berres, A. T. Wright, M. Sifri, N. C. Sadler, N. Tatiyaborworntham, and N. Li. 2016. Role of cytochrome p450 hydroxylase in the decreased accumulation of vitamin e in muscle from turkeys compared to that from chickens. Journal of Agricultural and Food Chemistry 64 (3):671–80. doi: 10.1021/acs.jafc.5b05433.
- Philpot, J. S. 1963. The estimation and identification of organic peroxides. Radiation Research 3 (Suppl 3):55–70. doi: 10.2307/3583676.
- Pliss, E. M., M. E. Soloviev, D. V. Loshadkin, S. V. Molodochkina, and O. T. Kasaikina. 2021. Kinetic model of polyunsaturated fatty acids oxidation in micelles. Chemistry and Physics of Lipids 237:105089. doi: 10.1016/j.chemphyslip.2021.105089.
- Puppo, A, and B. Halliwell. 1988. Formation of hydroxyl radicals from hydrogen peroxide in the presence of iron. Is haemoglobin a biological fenton reagent? The Biochemical Journal 249 (1):185–90. doi: 10.1042/bj2490185.
- Raleigh, J. A, and W. Kremers. 1978. Promotion of radiation peroxidation in models of lipid membranes by caesium and rubidium counter-ions: Micellar linoleic and linolenic acids. International Journal of Radiation Biology and Related Studies in Physics, Chemistry and Medicine 34 (5):439–47. doi: 10.1080/09553007814551101.
- Rey, A. I., C. J. Lopez-Bote, and R. S. Arias. 1997. Effect of extensive feeding on α-tocopherol concentration and oxidative stability of muscle microsomes from iberian pigs. Animal Science 65 (3):515–20. doi: 10.1017/S1357729800008729.
- Rhee, K. S., T. R. Dutson, and G. C. Smith. 1984. Enzymic lipid-peroxidation in microsomal fractions from beef skeletal-muscle. Journal of Food Science 49 (3):675–9. doi: 10.1111/j.1365-2621.1984.tb13186.x.
- Richards, M. P. 2010. Heme proteins and oxidation in fresh and processed meats. In Oxidation in foods and beverages and antioxidant applications, 76–104. Amsterdam, Netherlands: Elsevier. doi: 10.1533/9780857090447.1.77.
- Richards, M. P., H. Cai, and E. W. Grunwald. 2009. Phenylalanine substitution at site b10 (l29f) inhibits metmyoglobin formation and myoglobin-mediated lipid oxidation in washed fish muscle: Mechanistic implications. Journal of Agricultural and Food Chemistry 57 (17):7997–8002. 17 doi: 10.1021/jf901147a.
- Richards, M. P, and H. O. Hultin. 2001. Rancidity development in a fish model system as affected by phospholipids. Journal of Food Lipids 8 (3):215–30. doi: 10.1111/j.1745-4522.2001.tb00197.x.
- Richards, M. P, and H. O. Hultin. 2002. Contributions of blood and blood components to lipid oxidation in fish muscle. Journal of Agricultural and Food Chemistry 50 (3):555–64. doi: 10.1021/jf010562h.
- Richards, M. P., A. M. Modra, and R. Li. 2002a. Role of deoxyhemoglobin in lipid oxidation of washed cod muscle mediated by trout, poultry and beef hemoglobins. Meat Science 62 (2):157–63. doi: 10.1016/s0309-1740(01)00242-x.
- Richards, M. P., N. M. Nelson, H. G. Kristinsson, S. S. Mony, H. T. Petty, and A. C. Oliveira. 2007. Effects of fish heme protein structure and lipid substrate composition on hemoglobin-mediated lipid oxidation. Journal of Agricultural and Food Chemistry 55 (9):3643–54. doi: 10.1021/jf0628633.
- Richards, M. P., H. Ostdal, and H. J. Andersen. 2002b. Deoxyhemoglobin-mediated lipid oxidation in washed fish muscle. Journal of Agricultural and Food Chemistry 50 (5):1278–83. doi: 10.1021/jf011093m.
- Rosario, D. K., M. R. Furtado, Y. S. Mutz, B. L. Rodrigues, Y. A. Bernardo, J. D. Baltar, P. C. Bernardes, M. Estevez, and C. A. Conte-Junior. 2020. A chemometric approach to establish underlying connections between lipid and protein oxidation and instrumental color and texture characteristics in brazilian dry-cured loin. Foods 9 (4):536. doi: 10.3390/foods9040536.
- Rubio, N., I. Datar, D. Stachura, D. Kaplan, and K. Krueger. 2019. Cell-based fish: A novel approach to seafood production and an opportunity for cellular agriculture. Frontiers in Sustainable Food Systems 3:43. doi: 10.3389/fsufs.2019.00043.
- Russell, E. A., P. B. Lynch, K. O’sullivan, and J. P. Kerry. 2004. Dietary supplementation of alpha-tocopheryl acetate on alpha-tocopherol levels in duck tissues and its influence on meat storage stability. International Journal of Food Science and Technology 39 (3):331–40. doi: 10.1111/j.1365-2621.2004.00790.x.
- Sabatini, D. D. 2014. 2014. Preparation of rough microsomes from rat liver. Cold Spring Harbor Protocols 2014 (8):845–51. doi: 10.1101/pdb.prot079970.
- Sabow, A. B., I. Zulkifli, Y. M. Goh, M. Z. Ab Kadir, U. Kaka, J. C. Imlan, A. A. Abubakar, K. D. Adeyemi, and A. Q. Sazili. 2016. Bleeding efficiency, microbiological quality and oxidative stability of meat from goats subjected to slaughter without stunning in comparison with different methods of pre-slaughter electrical stunning. PLoS One 11 (4):e0152661. doi: 10.1371/journal.pone.0152661.
- Salami, S. A., M. N. O’grady, G. Luciano, A. Priolo, M. Mcgee, A. P. Moloney, and J. P. Kerry. 2021. Concentrate supplementation with dried corn gluten feed improves the fatty acid profile of longissimus thoracis muscle from steers offered grass silage. Journal of the Science of Food and Agriculture 101 (11):4768–78. doi: 10.1002/jsfa.11123.
- Salgo, M. G., F. P. Corongiu, and A. Sevanian. 1992. Peroxidation and phospholipase a2 hydrolytic susceptibility of liposomes consisting of mixed species of phosphatidylcholine and phosphatidylethanolamine. Biochimica et Biophysica Acta 1127 (2):131–40. doi: 10.1016/0005-2760(92)90268-z.
- Salvia-Trujillo, L., R. Soliva-Fortuny, M. A. Rojas-Grau, D. J. Mcclements, and O. Martin-Belloso. 2017. Edible nanoemulsions as carriers of active ingredients: A review. Annual Review of Food Science and Technology 8:439–66. doi: 10.1146/annurev-food-030216-025908.
- Sannaveerappa, T., N. G. Carlsson, A. S. Sandberg, and I. Undeland. 2007a. Antioxidative properties of press juice from herring (Clupea harengus) against hemoglobin (hb) mediated oxidation of washed cod mince. Journal of Agricultural and Food Chemistry 55 (23):9581–91. doi: 10.1021/jf071237i.
- Sannaveerappa, T., A. S. Sandberg, and I. Undeland. 2007b. Evaluation of occasional nonresponse of a washed cod mince model to hemoglobin (hb)-mediated oxidation. Journal of Agricultural and Food Chemistry 55 (11):4429–35. doi: 10.1021/jf063065f.
- Schnitzer, E., I. Pinchuk, and D. Lichtenberg. 2007. Peroxidation of liposomal lipids. European Biophysics Journal: EBJ 36 (4–5):499–515. doi: 10.1007/s00249-007-0146-2.
- Scollo, F., C. Tempra, F. Lolicato, M. F. M. Sciacca, A. Raudino, D. Milardi, and C. L. Rosa. 2018. Phospholipids critical micellar concentrations trigger different mechanisms of intrinsically disordered proteins interaction with model membranes. The Journal of Physical Chemistry Letters 9 (17):5125–9. doi: 10.1021/acs.jpclett.8b02241.
- Shahidi, F, and Y. Zhong. 2015. Measurement of antioxidant activity. Journal of Functional Foods 18:757–81. doi: 10.1016/j.jff.2015.01.047.
- Sharifian, S., E. Alizadeh, M. S. Mortazavi, and M. Shahriari Moghadam. 2014. Effects of refrigerated storage on the microstructure and quality of grouper (Epinephelus coioides) fillets. Journal of Food Science and Technology 51 (5):929–35.
- Shewfelt, R. L, and H. O. Hultin. 1983. Inhibition of enzymic and non-enzymic lipid peroxidation of flounder muscle sarcoplasmic reticulum by pretreatment with phospholipase a2. Biochimica et Biophysica Acta 751 (3):432–8. doi: 10.1016/0005-2760(83)90303-x.
- Soyer, A, and H. O. Hultin. 2000. Kinetics of oxidation of the lipids and proteins of cod sarcoplasmic reticulum. Journal of Agricultural and Food Chemistry 48 (6):2127–34. doi: 10.1021/jf990780z.
- Srinivasan, S, and R. O. Recknagel. 1971. A note on the stability of conjugated diene absorption of rat liver microsomal lipids after carbon tetrachloride poisoning. Journal of Lipid Research 12 (6):766–7. doi: 10.1016/S0022-2275(20)39466-9.
- Svingen, B. A., J. A. Buege, F. O. O’neal, and S. D. Aust. 1979. The mechanism of nadph-dependent lipid peroxidation. The propagation of lipid peroxidation. The Journal of Biological Chemistry 254 (13):5892–9. doi: 10.1016/S0021-9258(18)50498-1.
- Szebeni, J., H. Hauser, C. D. Eskelson, R. R. Watson, and K. H. Winterhalter. 1988. Interaction of hemoglobin derivatives with liposomes. Membrane cholesterol protects against the changes of hemoglobin. Biochemistry 27 (17):6425–34. doi: 10.1021/bi00417a034.
- Tappel, A. L. 1955. Unsaturated lipide oxidation catalyzed by hematin compounds. The Journal of Biological Chemistry 217 (2):721–33.
- Tatiyaborworntham, N, and M. P. Richards. 2018. Mechanisms involved in hemoglobin-mediated oxidation of lipids in washed fish muscle and inhibitory effects of phospholipase a2. Journal of the Science of Food and Agriculture 98 (7):2816–23. doi: 10.1002/jsfa.8779.
- Tatiyaborworntham, N., J. Yin, and M. P. Richards. 2021. Factors influencing the antioxidant effect of phospholipase a2 against lipid oxidation promoted by trout hemoglobin and hemin in washed muscle. Food Chemistry 343:128428. doi: 10.1016/j.foodchem.2020.128428.
- Terevinto, A., A. Ramos, G. Castroman, M. C. Cabrera, and A. Saadoun. 2010. Oxidative status, in vitro iron-induced lipid oxidation and superoxide dismutase, catalase and glutathione peroxidase activities in rhea meat. Meat Science 84 (4):706–10. doi: 10.1016/j.meatsci.2009.11.007.
- Thanonkaew, A., S. Benjakul, W. Visessanguan, and E. A. Decker. 2005. Lipid oxidation in microsomal fraction of squid muscle (Loligo peali). Journal of Food Science 70 (8):C478–C82. doi: 10.1111/j.1365-2621.2005.tb11504.x.
- Thanonkaew, A., S. Benjakul, W. Visessanguan, and E. A. Decker. 2007. Yellow discoloration of the liposome system of cuttlefish (Sepia pharaonis) as influenced by lipid oxidation. Food Chemistry 102 (1):219–24. doi: 10.1016/j.foodchem.2006.05.008.
- Tolasa Yılmaz, Ş., Ş. Çaklı, E. B. Şen Yılmaz, F. Kırlangıç, and C. Lee. 2018. Effect of fillet temperature on lipoxygenase activity in sardine mince with and without milk protein concentrate. Lwt 90:38–44. doi: 10.1016/j.lwt.2017.12.006.
- Tongnuanchan, P., S. Benjakul, and T. Prodpran. 2011. Roles of lipid oxidation and ph on properties and yellow discolouration during storage of film from red tilapia (Oreochromis niloticus) muscle protein. Food Hydrocolloids. 25 (3):426–33. doi: 10.1016/j.foodhyd.2010.07.013.
- Tuomisto, H. L. 2018. The eco‐friendly burger. EMBO Reports 20 (1):e47395. doi: 10.15252/embr.201847395.
- Tzankova, V., C. Gorinova, M. Kondeva-Burdina, R. Simeonova, S. Philipov, S. Konstantinov, P. Petrov, D. Galabov, and K. Yoncheva. 2017. Antioxidant response and biocompatibility of curcumin-loaded triblock copolymeric micelles. Toxicology Mechanisms and Methods 27 (1):72–80. doi: 10.1080/15376516.2016.1253811.
- Undeland, I. 2016. Oxidative stability of seafood. In Oxidative stability and shelf life of foods containing oils and fats, 391–460. Amsterdam, Netherlands: Elsevier. doi: 10.1016/b978-1-63067-056-6.00011-2.
- Undeland, I., H. O. Hultin, and M. P. Richards. 2002. Added triacylglycerols do not hasten hemoglobin-mediated lipid oxidation in washed minced cod muscle. Journal of Agricultural and Food Chemistry 50 (23):6847–53. doi: 10.1021/jf0201982.
- Undeland, I., H. O. Hultin, and M. P. Richards. 2003. Aqueous extracts from some muscles inhibit hemoglobin-mediated oxidation of cod muscle membrane lipids. Journal of Agricultural and Food Chemistry 51 (10):3111–9. doi: 10.1021/jf020770p.
- Undeland, I., H. G. Kristinsson, and H. O. Hultin. 2004. Hemoglobin-mediated oxidation of washed minced cod muscle phospholipids: Effect of ph and hemoglobin source. Journal of Agricultural and Food Chemistry 52 (14):4444–51. doi: 10.1021/jf030560s.
- Vallejo-Cordoba, B., R. Rodriguez-Ramirez, and A. F. Gonzalez-Cordova. 2010. Capillary electrophoresis for bovine and ostrich meat characterisation. Food Chemistry 120 (1):304–7. doi: 10.1016/j.foodchem.2009.09.080.
- Vieira, S., G. D. Zhang, and E. A. Decker. 2017. Biological implications of lipid oxidation products. Journal of the American Oil Chemists’ Society 94 (3):339–51. doi: 10.1007/s11746-017-2958-2.
- Vitrac, H., C. Hauville, F. Collin, M. Couturier, P. Therond, M. Delaforge, S. Remita, D. Jore, and M. Gardes-Albert. 2005. Hydroperoxide characterisation as a signature of the micelle/monomer balance in radiation-induced peroxidation of arachidonate. Free Radical Research 39 (5):519–28. doi: 10.1080/10715760500092543.
- Vossen, R. C. R. M., M. C. E. van Dam-Mieras, G. Hornstra, and R. F. A. Zwaal. 1993. Continuous monitoring of lipid-peroxidation by measuring conjugated diene formation in an aqueous liposome suspension. Lipids 28 (9):857–61. doi: 10.1007/BF02536243.
- Wang, Z. M., Z. F. He, A. M. Emara, X. Gan, and H. J. Li. 2019. Effects of malondialdehyde as a byproduct of lipid oxidation on protein oxidation in rabbit meat. Food Chemistry 288:405–12. doi: 10.1016/j.foodchem.2019.02.126.
- Wills, E. 1964. The effect of inorganic iron on the thiobarbituric acid method for the determination of lipid peroxides. Biochimica et Biophysica Acta (BBA) – Specialized Section on Lipids and Related Subjects 84 (4):475–7. doi: 10.1016/0926-6542(64)90016-2.
- Wills, E. D. 1966. Mechanisms of lipid peroxide formation in animal tissues. The Biochemical Journal 99 (3):667–76. doi: 10.1042/bj0990667.
- Windmueller, H. G. 1982. Glutamine utilization by the small intestine. In Advances in enzymology – and related areas of molecular biology, ed. D. Purich, 201–37. Hoboken, New Jersey: John Wiley & Sons. doi: 10.1002/9780470122983.ch6.
- Wu, G., A. G. Borbolla, and D. A. Knabe. 1994. The uptake of glutamine and release of arginine, citrulline and proline by the small intestine of developing pigs. The Journal of Nutrition 124 (12):2437–44. doi: 10.1093/jn/124.12.437.
- Wu, H., M. Abdollahi, and I. Undeland. 2021a. Effect of recovery technique, antioxidant addition and compositional features on lipid oxidation in protein enriched products from cod- salmon and herring backbones. Food Chemistry 360:129973. doi: 10.1016/j.foodchem.2021.129973.
- Wu, H., B. Forghani, M. Abdollahi, and I. Undeland. 2022a. Lipid oxidation in sorted herring (Clupea harengus) filleting co-products from two seasons and its relationship to composition. Food Chemistry 373 (Pt B):131523.
- Wu, H., S. Ghirmai, and I. Undeland. 2020. Stabilization of herring (Clupea harengus) by-products against lipid oxidation by rinsing and incubation with antioxidant solutions. Food Chemistry 316 (126337):126337. doi: 10.1016/j.foodchem.2020.126337.
- Wu, H., M. P. Richards, and I. Undeland. 2022b. Lipid oxidation and antioxidant delivery systems in muscle food. Comprehensive Reviews in Food Science and Food Safety 21 (2):1275–99. doi: 10.1111/1541-4337.12890.
- Wu, H., C. Tullberg, S. Ghirmai, and I. Undeland. 2022c. Pro-oxidative activity of trout and bovine hemoglobin during digestion using a static in vitro gastrointestinal model. Food Chemistry 393:133356. doi: 10.1016/j.foodchem.2022.133356.
- Wu, H., S. Xiao, J. Yin, J. Zhang, and M. P. Richards. 2021b. Impact of lipid composition and muscle microstructure on myoglobin-mediated lipid oxidation in washed cod and pig muscle. Food Chemistry 336:127729. doi: 10.1016/j.foodchem.2020.127729.
- Wu, H., S. Xiao, J. Yin, J. Zhang, and M. P. Richards. 2021c. Mechanisms involved in the inhibitory effects of free fatty acids on lipid peroxidation in turkey muscle. Food Chemistry 342:128333. doi: 10.1016/j.foodchem.2020.128333.
- Wu, H., J. Yin, S. L. Xiao, J. H. Zhang, and M. P. Richards. 2022d. Quercetin as an inhibitor of hemoglobin-mediated lipid oxidation: Mechanisms of action and use of molecular docking. Food Chemistry 384:132473. doi: 10.1016/j.foodchem.2022.132473.
- Wu, H., J. Yin, J. H. Zhang, and M. P. Richards. 2017. Factors affecting lipid oxidation due to pig and turkey hemolysate. Journal of Agricultural and Food Chemistry 65 (36):8011–7. 36doi: 10.1021/acs.jafc.7b02764.
- Xiao, S. L., H. Zhuang, G. H. Zhou, and J. H. Zhang. 2018. Investigation of inhibition of lipid oxidation by l-carnosine using an oxidized-myoglobin-mediated washed fish muscle system. Lwt 97:703–10. doi: 10.1016/j.lwt.2018.08.003.
- Yamauchi, R., T. Kinoshita, Y. Hasegawa, and S. Iwamoto. 2017. Hemin- and myoglobin-catalyzed reaction of 1-palmitoyl-2-linoleoyl-3-sn-phosphatidylcholine 13-hydroperoxide with gamma-tocopherol in micelles and liposomes. Chemistry and Physics of Lipids 209:37–44. doi: 10.1016/j.chemphyslip.2017.11.005.
- Yamazaki, H., K. Inoue, C. G. Turvy, F. P. Guengerich, and T. Shimada. 1997. Effects of freezing, thawing, and storage of human liver samples on the microsomal contents and activities of cytochrome p450 enzymes. Drug Metabolism and Disposition: The Biological Fate of Chemicals 25 (2):168–74.
- Yan, L, and X. Qiu-Zhou. 2006. Dietary glutamine supplementation improves structure and function of intestine of juvenile jian carp (Cyprinus carpio var. Jian). Aquaculture 256 (1-4):389–94. doi: 10.1016/j.aquaculture.2006.02.011.
- Yang, Y., Y. Sun, D. Pan, Y. Wang, and J. Cao. 2018. Effects of high pressure treatment on lipolysis-oxidation and volatiles of marinated pork meat in soy sauce. Meat Science 145:186–94. doi: 10.1016/j.meatsci.2018.06.036.
- Yin, D., H. Lingnert, B. Ekstrand, and U. T. Brunk. 1992. Fenton reagents may not initiate lipid peroxidation in an emulsified linoleic acid model system. Free Radical Biology and Medicine 13 (5):543–56. doi: 10.1016/0891-5849(92)90149-B.
- Yin, J., E. M. Becker, M. L. Andersen, and L. H. Skibsted. 2012. Green tea extract as food antioxidant. Synergism and antagonism with alpha-tocopherol in vegetable oils and their colloidal systems. Food Chemistry 135 (4):2195–202. doi: 10.1016/j.foodchem.2012.07.025.
- Yin, J., W. Zhang, and M. P. Richards. 2017. Attributes of lipid oxidation due to bovine myoglobin, hemoglobin and hemolysate. Food Chemistry 234:230–5. doi: 10.1016/j.foodchem.2017.04.182.
- Yin, M. C, and C. Faustman. 1993. Influence of temperature, ph, and phospholipid-composition upon the stability of myoglobin and phospholipid – a liposome model. Journal of Agricultural and Food Chemistry 41 (6):853–7. doi: 10.1021/jf00030a002.
- Yin, S., C. Faustman, N. Tatiyaborworntham, R. Ramanathan, and Q. Sun. 2013. The effects of hne on ovine oxymyoglobin redox stability in a microsome model. Meat Science 95 (2):224–8. doi: 10.1016/j.meatsci.2013.04.055.
- Zalkin, H, and A. L. Tappel. 1960. Studies of the mechanism of vitamin E action. Iv. Lipide peroxidation in the vitamin e-deficient rabbit. Archives of Biochemistry and Biophysics 88 (1):113–7. doi: 10.1016/0003-9861(60)90205-8.
- Zhang, Y. F., X. J. Tian, Y. Z. Jiao, Y. Wang, J. Dong, N. Yang, Q. H. Yang, W. Qu, and W. H. Wang. 2022. Free iron rather than heme iron mainly induces oxidation of lipids and proteins in meat cooking. Food Chemistry 382:132345. doi: 10.1016/j.foodchem.2022.132345.
- Zhou, F., S. Jongberg, M. Zhao, W. Sun, and L. H. Skibsted. 2016. Iron(ii) initiation of lipid and protein oxidation in pork: The role of oxymyoglobin. Journal of Agricultural and Food Chemistry 64 (22):4618–26. doi: 10.1021/acs.jafc.6b01168.