Abstract
Maize gluten meal (MGM) is a by-product of maize starch and ethanol, produced by the wet milling process. Its high protein content makes it a preferred ingredient in feed. Given the high prevalence of mycotoxins in maize globally, they pose a significant challenge to use of MGM for feed: wet milling could concentrate certain mycotoxins in gluten components, and mycotoxin consumption affects animal health and can contaminate animal-source foods. To help confront this issue, this paper summarizes mycotoxin occurrence in maize, distribution during MGM production and mycotoxin risk management strategies for MGM through a comprehensive literature review. Available data emphasize the importance of mycotoxin control in MGM and the necessity of a systematic control approach, which includes: good agriculture practices (GAP) in the context of climate change, degradation of mycotoxin during MGM processing with SO2 and lactic acid bacteria (LAB) and the prospect of removing or detoxifying mycotoxins using emerging technologies. In the absence of mycotoxin contamination, MGM represents a safe and economically critical component of global animal feed. With a holistic risk assessment-based, seed-to-MGM-feed systematic approach to reducing and decontaminating mycotoxins in maize, costs and negative health impacts associated with MGM use in feed can be effectively reduced.
Introduction
Worldwide maize production and use
Maize, Zea mays L. (also called corn), is considered the third most important global crop after wheat and rice, based on the area harvested (Ramirez-Cabral, Kumar, and Shabani Citation2017); more recent publications rank it second, after wheat (Erenstein et al. Citation2022). It can be readily produced areas across a wide range of agroecologies, with a relatively limited number of rainfall events during the production season, and is grown in every continent except Antarctica. From 1961 to 2020, the global area under maize production has increased from 106 million to 201 million hectares, while production increased from 205 million to 1.16 billion tons (Food and Agriculture Organization). Average maize yield has increased from 1.94 ton/ha to 5.75 ton/ha. As of 2020, the U.S.A, China and Brazil are the top three maize-producing countries in the world, contributing 31.0%, 22.4%, and 8.9% respectively of total production (FAOSTAT Citation2021) ().
Figure 1. Maize production a) and yields b) of the world, U.S., China and Brazil from 1961 to 2020 (FAOSTAT Citation2021).
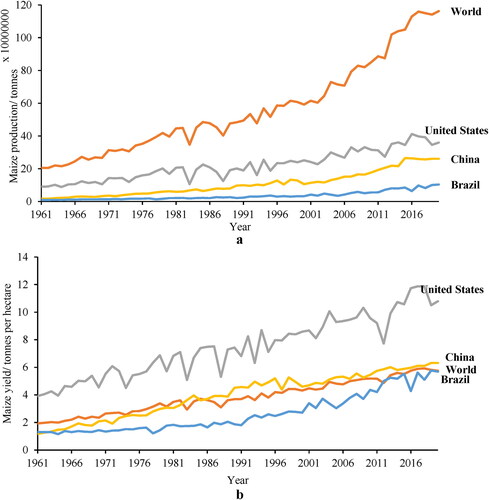
Global use of maize as both food and feed has increased accordingly, with maize having high worldwide value, nutritionally and commercially. With an energy density of 365 kcal/100 g, maize contains approximately 72% starch, 10% protein, and 4% fat, (Ranum, Peña‐Rosas, and Garcia‐Casal Citation2014; Dula Citation2019). Commonly used as a staple food, maize accounts for about 15-56% of daily caloric intake in 25 developing countries (Dubey et al. Citation2020). In addition to direct human consumption, maize is extensively processed in wet milling, dry milling, distilling and feed compounding for a broad range of industries. Food and industrial products produced from maize include staple foods, snacks, starch, MGM, sweeteners, cooking oils, alcoholic beverages, glue, and industrial alcohol (Ranum, Peña‐Rosas, and Garcia‐Casal Citation2014). Therefore, any food safety issue that threatens maize production poses a significant global challenge across a broad range of food and feed applications.
MGM production and use
Given that maize is such an important global crop, innovations for use in food, feed and industrial applications represent a major focus for research, industry and global food security. Maize gluten meal (MGM) is a major by-product of wet milling for starch and sometimes ethanol production, illustrated in . MGM is prepared by re-centrifugation, filtering and drying of the gluten slurry obtained from the maize starch gluten separation process. Starch and oil-rich germ are the main products from wet milling, comprising 75% on a dry weight basis. Following removal of these main products, fiber, and soluble components, MGM accounts for about 5% of the dry weight.
Figure 2. Maize wet milling process and MGM.
a) wet milling process, b) dry MGM, c) wet MGM. a) adapted from Blasi et al. (Citation2001); b) and c) from Ribeiro et al. (Citation2019) under CC BY 4.0.
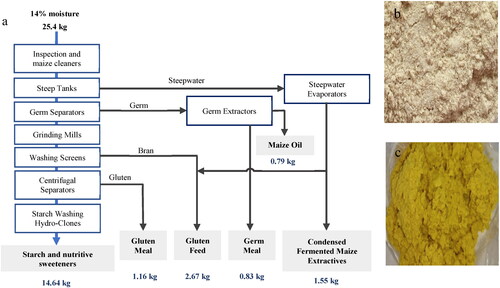
MGM is a protein-rich feed containing over 60% crude protein (dry basis) and low crude fat. It is used extensively as a source of protein, xanthophyll, energy and pigments for livestock, poultry, pets and fish feed, due to its high protein digestibility and gross energy content (RFA. Citation2008). Indeed, due to its commercial value and benefit to livestock, MGM has wide acceptance in the feed and pet industry, with an annual usage of about a million tons (Erickson, Klopfenstein, and Watson Citation2012).
MGM has a wide range of important applications beyond being used as a feed additive. Historically, MGM has been used as both fertilizer and a pre-emergent weed killer, making it useful in organic agriculture (Pasupuleti and Demain Citation2010). MGM is the major source for extracting zein, which has diverse uses, from edible food coating to industrial applications (Zheng et al. Citation2006; Anderson and Lamsal Citation2011). In the food industry, enzymatic hydrolysis or microbial fermentation of MGM to obtain bioactive peptides (Zhou et al. Citation2015) is increasing the interest in MGM for new functional dietary products (Li et al. Citation2019).
Demand and high-value applications for MGM are increasing. The global volume of MGM was estimated to be 10.22 million tons in 2019, based on the amount of wet milling carried out (Rausch et al. Citation2019). The U.S.A. and China account for more than 50% of global maize production and lead in the production of MGM. Enabling full utilization of MGM, through reducing mycotoxin contamination, will help to meet the global demand for these protein resources and minimize health risks in animals, and to humans who may be exposed through contaminated animal-source food.
Major mycotoxins and worldwide occurrence in maize
Major mycotoxins in maize
Given the susceptibility of maize and its kernels to fungal colonization, maize is vulnerable to fungal toxin (mycotoxin) contamination during both pre- and post-harvest phases. Mycotoxins pose major health risks for both humans and animals. Exposure can result in acute or chronic consequences including carcinogenic, teratogenic, immunosuppressive, or estrogenic effects (Carbas et al. Citation2021). Mycotoxin contamination can greatly increase losses and management costs, comprising both direct economic loss from reduced yield and crop value, and indirect loss due to reduced animal productivity, human health costs, and the costs of risk management and research (Munkvold et al. Citation2019). As an illustration of the economic importance of this issue, it has been estimated that in a year of conducive climatic conditions, aflatoxin – only one category of mycotoxins – could cause up to $1.68 billion of losses to US maize production alone (Mitchell et al. Citation2016).
Maize is susceptible to different fungal pathogens from growing in the field to product storage (Giorni, Bertuzzi, and Battilani Citation2019). Environmental conditions have a strong influence on mycotoxin development during each phase. The diversity of fungi that colonize maize and its kernels, the toxins they produce, and the stages of production and storage affected are summarized below.
Fusarium mycotoxins
Maize is susceptible to attack by Fusarium spp. in the field, with resultant production of Fusarium mycotoxins. Fumonisins (FBs), Trichothecenes (type A, T-2/HT-2 toxins and type B, deoxynivalenol (DON)) and zearalenone (ZEN) are common mycotoxins in maize and are mainly produced by several Fusarium species.
FBs, mainly produced by F. verticillioides, are the most common mycotoxins with the highest risk, because of the worldwide distribution of F. verticillioides (Munkvold et al. Citation2019), with DON being one of the most frequently detected. In regions with cooler climates, the occurrence of DON in maize is considered as a typical agricultural issue, as weather conditions are favorable for F. graminearum and F. culmorum growth and DON production (Kos et al. Citation2017).
T-2/HT-2 toxins are mainly produced by F. sporotrichioides, F. langsethiae and F. poae, and belong to type A trichothecene mycotoxins. These Fusarium species are widespread in both South and North America, Asia, Africa, and Europe (Richard et al. Citation2007) and produce T-2/HT-2 under moist cool conditions prior to cereals harvest (Porricelli et al. Citation2016).
ZEN is a nonsteroidal estrogenic mycotoxin primarily produced by is F. graminearum. Contamination of cereal grains by ZEN has been reported worldwide, primarily in temperate climates.
Some Fusarium species and their mycotoxins can also develop in stored maize when storage conditions are less than ideal. For example, FBs increased from 20 to 40% during a six-month storage due to growth of F. verticillioides (Carbas et al. Citation2021). Similarly, ZEN was also increased when the moisture content of the storage environment was greater than 30-40% (Gupta Citation2007).
Aflatoxin
The most recognized aflatoxigenic fungi are A. flavus and A. parasiticus. Maize is one of the major sources of human exposure to dietary aflatoxin (AF) globally, with an approximate contribution of more than 10% (JECFA. Citation2017; Schaarschmidt and Fauhl-Hassek Citation2021). Pre- and post- harvest AF contamination of maize production is highly dependent on biological (biotic) and environmental (abiotic) factors. Drought stress, excessive rainfall during planting and excessive water and temperature during storage will promote the growth of mold and the production of aflatoxin (Bhatnagar-Mathur et al. Citation2015).
Ochratoxins
Ochratoxins (OTA) may be significant mycotoxins in maize in some parts of the world. OTA is produced mainly by Penicillium. verrucosum in temperate climates and Aspergillus ochraceus and the rare Aspergillus carbonarius in warm and tropical climates. These fungi can contaminate agricultural products before harvest, but more commonly during storage (Ozbey and Kabak Citation2012).
Mycotoxin prevalence of maize
A global dataset of mycotoxin prevalence in maize sampled in different continents (including America, Europe, Asia, Middle East and Africa), conducted by the company BIOMIN, is summarized below (). While samples sizes for some regions (e.g., Middle East and Northern Africa) are too small to allow conclusions, this survey does provide a sense of mycotoxin contamination levels for many regions. Munkvold et al. (Citation2019) introduced the global compilation of several mycotoxins in maize, surveyed from 2014 to 2017 (including 11,237 maize samples collected in 75 countries) and Gruber-Dorninger, Jenkins, and Schatzmayr (Citation2019) performed a large scale survey of mycotoxin contamination in maize samples collected from 100 countries between 2008 and 2017. Both authors reached the similar conclusion that FBs-contaminated samples were the most abundant positive samples (>80%), followed by DON > ZEN > AFs > T-2 > OTA. BIOMIN’s survey also found that Fusarium mycotoxins, FBs, DON and ZEN, were much more prevalent compared with AFs, OTA and T-2 toxin between 2019 and 2020. This survey also noted that T-2 toxin occurred frequently in the European Union and high positive rates were also found in the Middle East and North Africa. Other literature supported the finding that T-2 toxin is widespread in the European Union (Vulic, Pleadin, and Persi Citation2011; Pleadin et al. Citation2012).
Table 1. Mycotoxin prevalence in maize in different regions.
Globally, at least one mycotoxin proved to be present in more than 90% of the samples tested. Contamination with multiple mycotoxins was also common, with 68% of samples contaminated with at least two mycotoxins (Leite et al. Citation2021). The co-occurrence percentage of aflatoxin B1(AFB1) and OTA in maize flours in the period between 2014 and 2016 was 7.1% − 34.4% (Torovic Citation2018). In 705 maize samples from 26 US states across 2015–2016, contamination with three mycotoxins was found in 21% of the samples (Hendel et al. Citation2017). During 2008–2017, FBs and DON, FBs and ZEN, and DON and ZEN co-occurred in 49%, 37% and 39% respectively of global maize samples tested (Gruber-Dorninger, Jenkins, and Schatzmayr Citation2019). In a study of mycotoxin occurrence in maize kernels in Spain from 2015 to 2019, co-occurrence of several mycotoxins (BIOMIN Citation2019; Munkvold Citation2003; Garcia-Diaz et al. Citation2020) reached quantifiable levels in 33.5% of samples (Tarazona et al. Citation2020). The synergistic effect of co-occurring mycotoxins could cause severe harm at relatively low levels. Therefore, multiple strategies are needed to mitigate risks, which increases the difficulty of mycotoxin risk control.
Mycotoxin incidence in maize varies between regions, due to differences in the geographic locations of maize growth including meteorological conditions (Perrone et al. Citation2020) and toxigenicity of fungal populations (Okoth et al. Citation2012). Each region has its own unique mycotoxin pattern. A key driver of this high correlation between mycotoxin prevalence and region is climatic characteristics (humidity, temperature and rain fall), which provide the conditions for toxigenic fungi infection and mycotoxin accumulation (Paterson, Russell, and Lima Citation2010). When climate conditions are favorable for fungal growth, contamination with mycotoxins is more severe (Mahdjoubi et al. Citation2020). For instance, Aspergillus fungi (AFs and OTA producers), especially A. flavus, are most common at latitudes 26-35 degrees North or South, mainly due to drought stress and high temperature (Munkvold et al. Citation2019).
The impacts of climate change on mycotoxin contamination of maize
It has been suggested that climate change is a worldwide factor driving emerging risks for food and feed safety, as well as for plant and animal health and nutritional characteristics. Reflecting this concern, the UN Environment Programme considered climate change-related increase of mycotoxin risk during their 2016 annual assembly (Harvey et al. Citation2016). Rainfall, humidity and temperature are major drivers of both maize stress (which increases susceptibility to fungal pathogens and mycotoxins) and fungal growth and mycotoxin production. The influences of climatic conditions on mycotoxigenic fungal infection and mycotoxin contamination of maize have been widely documented, and exploited for risk mapping and agricultural production simulation modeling (Moretti, Pascale, and Logrieco Citation2019; Chauhan et al. Citation2015). Multiple studies have shown that the fluctuation of weather conditions may cause interannual differences in observed mycotoxin incidences and concentrations and the presence of Fusarium spp. DNA (Gruber-Dorninger, Jenkins, and Schatzmayr Citation2018; Vandicke et al. Citation2019), translating to increased AFs risk in maize in Northern Italy and Eastern Europe over the last fifteen years (Perrone et al. Citation2020; Battilani et al. Citation2016). Vandicke et al. (Citation2019) also showed that the variation of weather conditions could influence fungal populations and significantly impact the corresponding mycotoxin contamination of maize. FBs were found in only 2% of the samples in 2016, which was wet and cold, but in 61% of samples in the hot and dry year 2018 in Flanders, Belgium.
The potential effects that are exerted by climate change on toxigenic fungi and mycotoxin risks warrant further study. Multidisciplinary cooperation is required to address these new fields and develop appropriate intervention strategies, such as creating a predictive model for farmers to diminish the impact of mycotoxins and thus secure food and feed safety.
Mycotoxins that contaminate maize carry over to MGM during processing. Furthermore, MGM production can actually concentrate certain mycotoxins, increasing contamination levels and making safe use of MGM potentially more challenging than the original raw material. With the widespread use and economic importance of MGM, mycotoxin contamination has become a critical challenge for its use in food and feed. Given the complexities of mycotoxigenic contamination of maize both pre- and post-harvest, that climate change is aggravating this challenge, and that MGM is used for food and feed, a systematic strategy to control mycotoxin risk in MGM is crucial to ensure its safe and economically viable use.
In order to identify critical points at which mycotoxins can be reduced or eliminated as they relate to MGM, the following sections discuss control strategies for mycotoxin risk reduction in maize production and storage, follow the fate of mycotoxins through the MGM production process, and review the potential application of sulfur dioxide (SO2) and lactic acid bacteria (LAB) for decontamination during processing. Finally, the potential application prospects, challenges, and opportunities for emerging technologies to control MGM mycotoxins are explored.
Mycotoxin distribution during MGM production
Mycotoxins are redistributed into different maize fractions during wet milling (). Different mycotoxins exhibit different migration characteristics, although the data suggest that most of the mycotoxin types found in maize are concentrated in the gluten fraction through the wet milling process–meaning that they are prone to enrichment during MGM production. Park et al. (Citation2018) reported that 31.3% of FB2 from maize was distributed into maize gluten, followed by OTA (30.6%), ZEN (27.7%), T-2 (24.2%), HT-2 (16.1%), AFB1 (15.1%), FB1 (12.7%) and DON (9%). Other studies reported that higher levels of ZEN (45-56%) were distributed into maize gluten (Romer Citation1984; Bennett and Anderson Citation1978). In addition, 11 − 25.3% of AFs has been observed reported that redistributed into gluten fraction (Yahl et al. Citation1971; Romer Citation1984; Schaarschmidt and Fauhl-Hassek Citation2021). Considering that the gluten content only accounts for 5% of the initial maize, the distribution rate of these mycotoxins demonstrates that the mycotoxins in MGM have not decreased, and some mycotoxins have accumulated significantly through wet milling (Schaarschmidt and Fauhl-Hassek Citation2021).
Table 2. Fate of mycotoxins during wet milling.
Redistribution of mycotoxins during the wet milling process may be affected by a number of factors. ZEN is mainly redistributed in soluble, fiber, germ and gluten, which are relatively small proportion (<30%) in maize (). However, there is little or even no ZEN detected in starch, which accounts for the highest proportion in maize (>65%) (Blasi et al. Citation2001). Therefore, the level of ZEN in the maize raw material is lower than all downstream products except starch, and ZEN is therefore enriched in the non-starch products. This result may be related to the poor water solubility of ZEN and its interaction with maize protein. By contrast, DON, T-2 and HT-2, exhibit high distribution rates in soluble components: Lauren and Ringrose (Citation1997) considered that the high concentration of DON in liquid fractions during wet milling was related to its high-water solubility. Collins and Rosen (Citation1981) and Park et al. (Citation2018) obtained similar results for T-2 redistribution through laboratory simulation and factory sampling.
There may be several reasons for the variation in distribution observed in different studies. Firstly, different process conditions were used for production of MGM. Scale was likely to have been an important factor as some of these studies were at factory scale whilst others were laboratory based. Secondly, the binding interactions between proteins and mycotoxins can make quantitative detection more variable because of the existence of hidden (or “masked”) mycotoxins. Recently, it was reported that ZEN in maize was underestimated (masked) because of the presence of zein-bound ZEN, and the amount of total detected ZEN significantly increased after hydrolysis (Tan et al. Citation2021). Thirdly, the effect of wet milling depends on characteristics of the maize batch, including how mycotoxins distribute between maize fractions during processing. Studies using different batches of “starting” maize with different levels of mycotoxin contamination have shown different degrees of mycotoxin distributed into maize gluten (Schaarschmidt and Fauhl-Hassek Citation2021). Finally, differences in sampling will also contribute to the different results.
Global mycotoxin occurrence data indicate that the prevalence of FBs, DON, ZEN and AFs are high in maize used for MGM production, especially FBs and ZEN (BIOMIN Citation2020, Citation2019). Due to mycotoxin enrichment during the wet milling process, MGM produced from a batch of compliant maize might be found to have excessive mycotoxins such as ZEN or FBs due to now elevated levels. Therefore, predicting the relationship between mycotoxin occurrence in “starting” maize and MGM, through specific and well-controlled milling processes, is critical for managing the risk of mycotoxin contamination of MGM. Furthermore, understanding the mechanism of mycotoxin re-distribution and accumulation will further provide directions to develop novel approaches for decontaminating mycotoxins in MGM.
Mycotoxin risk management strategies in maize and MGM
As goes the common adage, an ounce of prevention is worth a pound of cure. In other words, those who rely on clean raw materials for MGM production need to consider how that maize is produced in the first place and how that influences the risk of mycotoxin contamination. A systematic control strategy for maize pre-harvest, post-harvest and during processing is crucial to intercept, control and remove hazardous mycotoxins from the food chain. Multiple strategies have been established to manage either mycotoxin occurrence or fungal growth, or to eliminate and to degrade mycotoxins in foodstuffs (Xing et al. Citation2017). A sustainable and effective sourcing strategy may involve partnering with organizations and initiatives that specialize in promoting best maize production and postharvest practices.
Risk management strategies by GAP in maize
Good agricultural practices (GAP) refers to the best approaches, principles and standards applied to on-site farm production to assure the safety of food and non-food agricultural products. GAP has been demonstrated to be an effective strategy to control and manage contamination by mycotoxins from pre- to post-harvest. GAP is effective and practicable for mycotoxin control in maize in rural, commercial and sustainable applications. GAP has been recommended by the FAO with uniform guidance provided for all countries to consider (FAO Citation2003), with various agricultural development initiatives tailoring local, stakeholder-informed solutions for smallholder production systems. Given its documented effectiveness at reducing the risk of mycotoxin contamination in maize, as well as the win-win impacts of increasing yields and reducing post-harvest losses in general, GAP is an essential element that should be taken into consideration both within high risk regions for farmers and in industrial production (Thai Agriculture Standard Citation2010).
Pre-harvest strategies
Fungal infection starts at the pre-harvest stage in maize, so GAP needs to be implemented from the production stage to minimize mycotoxin contamination (Mahuku et al. Citation2019). Pre-harvest management includes mitigation of drought stress, insect infestation, and the primary inoculum. Humans have minimal control over environmental factors. However, good crop husbandry practices, such as crop rotation, proper tillage, irrigation, timely planting and harvesting, and pesticide usage where appropriate and safely practicable are preventative actions that reduce mycotoxin occurrence in crops (Erickson, Klopfenstein, and Watson Citation2012).
Pre-harvest can be divided into pre-planting, planting, and after-planting stages. During pre-planting, breaking the hardpan during tillage can reduce subsequent drought stress. During planting, healthy seeds of a variety adapted to the given agroecological conditions are recommended; these should be planted at an optimal planting time and plant density to avoid preferred conditions for mycotoxigenic fungi (Abbas et al. Citation2009).
Practices such as irrigation (where available), fertilizer use, insect control, weed control and fungicide use are critical for field management after planting, to reduce mycotoxigenic fungal contamination. It should be noted that many insects can spread or provide entry points for mycotoxigenic fungi in maize, for example, lepidopteran ear borer can spread A. flavus in harvested maize, and insect damage of maize can be a predictive indicator for Fusarium contamination. An appropriate pesticide or insect control can be beneficial in reducing mycotoxin contamination (Adegoke and Letum Citation2013). The capacity of actors in a given food system to appropriately and safely apply pesticides should be considered when assessing this intervention, given that 44% of farmers (385 million) are estimated to suffer from Unintentional Acute Pesticide Poisoning globally, many of whom are in developing countries (Boedeker et al. Citation2020).
Water stresses can be a driver of mycotoxin susceptibility. Excess moisture during flowering allows favorable conditions for the dissemination and infection by Fusarium; because of which, there should be appropriate restraint on irrigation during the flowering and crop maturation stages, where irrigation is used. For rainfed maize production, which represents much of production in developing countries, the use of adapted, drought-tolerant varieties can significantly reduce the risk of mycotoxin contamination before harvest.
The use of biocontrol agents have been developed to control mycotoxin production (Neaj, Habschied, and Mastanjevj Citation2021; Agriopoulou, Stamatelopoulou, and Varzakas Citation2020). Biocontrol of AF by competitive exclusion is a strategy in which non-toxigenic spores of A. flavus are introduced in large amounts into the soil of the fields where peanuts or maize are grown, thus outcompeting toxigenic spores and reducing AF accumulation. Some research reported that biocontrol could be fruitful in reducing aflatoxin-contamination of maize in Thailand, although results were variable (Pitt et al. Citation2015; Tran-Dinh, Pitt, and Markwell Citation2014, Citation2018). Pitt also discussed the advantages and related problems of the practical use of biocontrol to treat maize and reduce aflatoxin contamination in Africa (Pitt Citation2019). Other studies also reported high efficacy of biocontrol in reducing aflatoxin levels in maize, from field trials in Nigeria (Bandyopadhyay et al. Citation2019).
Post-harvest strategies
AF and OTA contamination can occur before and after harvest. Fusarium toxin contamination, such as with FB, ZEN and DON, is mainly a pre-harvest problem, but if the external environment used during storage, transportation, and processing of harvested crops meets the growth conditions for fungi, fungi will continue to grow and produce mycotoxins. Good post-harvest practices are, therefore, also important to avoid further mycotoxin contamination.
Timely harvest after crop maturity and adequate drying are recommended to reduce the risk of mycotoxin contamination in maize in high-risk regions, where fungal pathogens have a relatively shorter time to grow or potentially produce mycotoxins (Monda, Masanga, and Alakonya Citation2020). AFs will increase in maize when the plants proceed to maturity and harvest is delayed. One study found that AFs increased 4-7 fold after a 3-4 week delay in harvest (Hell et al. Citation2008). GAP also emphasizes proper drying after harvesting, i.e., avoid harvesting directly onto the soil, improved drying methods and good storage conditions can greatly reduce the risk of mycotoxin contamination, including in peanuts and maize (Turner et al. Citation2005). The common developing country practice of heaping maize in the field after harvest was found to significantly increase AFs contamination in farmers’ fields in Ghana (Manu et al. Citation2019). Contamination with AFs can increase 10 fold in three days when harvested maize is stored under high moisture content (Hell et al. Citation2008). When collecting and transporting the harvested crop from the field to the drying process, and/or to storage, the containers utilized should be clean and dry, meanwhile the vehicles should be checked to avoid insects, soil, or visible fungal growth (Demissie Citation2018).
De-husking and sorting, aiming to remove poor-quality ears, are also effective ways to reduce the spread of spores and subsequent infection. Xu et al. (Citation2021) suggested that the leafy outer covering of the maize could be removed manually before drying, because the husk could still protect the cobs during transportation. It has been suggested that maize ears should be removed if ear damage is greater than 10% (Setamou et al. Citation1998), however given that AF levels can be high even in visibly clean grains this practice may still fail to avert high levels of contamination.
Mycotoxin contamination during storage is mainly related to fungal load, moisture content, temperature, relative humidity, insect activities and packaging methods (Wang et al. Citation2021). Good field control will help to reduce fungal load. Good practice to avoid mycotoxin production in storage involves controlling moisture, which should be kept below 14% at 30 °C (corresponding to water activity below 0.70), temperature (<20 °C) and relative humidity (<80%) and maintaining proper ventilation. Under these conditions, the growth of fungi, the production of mycotoxins and the number of insects can be reduced (Channaiah and Maier Citation2014). In addition, pest and mycotoxin control can also be improved by closed storage, atmospheric control and the use of pesticides. Storage of maize and other crops in hermetic bags or silos is a highly effective method to arrest aflatoxin contamination in properly dried grains (Suleiman et al. Citation2018) and has been demonstrated to reduce the “lean season” where smallholder farming communities lack sufficient food between harvests (Brander, Bernauer, and Huss Citation2021). Hermetic storage technologies such as appropriate packaging (for example the Purdue Improved Crop Storage bag, and the Super Grain Bag) are also effective at eliminating insect pests (Singano, Mvumi, and Stathers Citation2019; Williams, Baributsa, and Woloshuk Citation2014). The use of phosphine fumigation is also beneficial in maize drying during storage, which helps to reduce mycotoxin contamination (FAO Citation1989). However, the safety of chemical residues should be fully guaranteed (Chulze Citation2010) and capacity of food system actors to safely apply them considered.
An appropriate training strategy needs to be developed for farmers and maize value chain workers to deploy these methods and GAP guidelines, so that mycotoxin contamination of maize is minimized before processing. A recent study in Kenya (Joutsjoki and Korhonen Citation2021) suggested that trained farmers deployed good practice, which resulted in a significant decrease of mycotoxin levels in maize. Xu et al. (Citation2021) provided practicable GAP for smallholder maize farmers to reduce aflatoxin contamination in high-risk regions. Massomo (Citation2020) also recommended an integrated action plan to address AF contamination in maize in Tanzania. GAP is an important tool to minimize mycotoxin occurrence and continuous monitoring of crops should be encouraged. Further research on the application of good agricultural storage practices and strategies to mitigate the mycotoxin risk in maize is critical (Carbas et al. Citation2021).
Risk management strategies for processing MGM
GAP can reduce mycotoxin contamination in maize, but not eliminate it. Mycotoxins may also be reduced through the physical separation, chemical inactivation and biological degradation that occurs during MGM processing.
Pre-processing
Regulations
To protect consumers from the harmful impact of mycotoxins, many countries have established regulations for mycotoxins in food and animal feed since the late 1960s. At least 99 countries specified mycotoxin limits for food and/or feed by late 2003 (FAO Citation2004). Maximum levels (MLs) in maize and its products have been agreed for FBs (sum of FB1 and FB2) and DON by the Codex Alimentarius Commission; application to commodities moving in international trade was recommended (FAO Citation1995). Corresponding MLs have been applied in EU regulations. AFB1, total AFs, AFM1, DON, total FBs, OTA, T-2 and HT-2 toxins and ZEN are regulated in unprocessed maize and different maize derivatives consumed by humans, as well as products used as animal feed (European Commission (EC)) Citation2006, Citation2007; Schaarschmidt and Fauhl-Hassek Citation2021). MLs ensure that food producers and processors consider measures to minimize the content of mycotoxins in maize and maize products.
Measurement
Measurement of mycotoxins is one of the key factors in mycotoxin management for surveillance, and to understand the occurrence, fate, and mitigation of mycotoxins in raw maize before processing and producing MGM. Several studies have reported that sampling is often the largest source of variability (among several sources of variability) in mycotoxin quantification, due to the highly heterogeneous distribution in contaminated raw and processed foods, for example, sampling attributed nearly 90% of the error when measuring AFs in some studies (Munkvold et al. Citation2019; Whitaker Citation2003; Romer Citation2006; Baker et al. Citation2014). Representative sampling becomes increasingly difficult as production scale increases (Schaarschmidt and Fauhl-Hassek Citation2021). A common sampling procedure has been established by the EU to reduce the variability caused by sampling. Thus, the establishment of an adequate sampling procedure is critical to guarantee reliable and accurate results (Pereira, Fernandes, and Cunha Citation2014).
Detection methods can also exert influence because different methods involve different extraction and clean-up procedures (Munkvold et al. Citation2019), and specific parameters (e.g. limit of detection) differ among studies (Leite et al. Citation2021). Simultaneous contamination with a variety of mycotoxins increases the difficulty of detection. Furthermore, the existence of hidden (masked) mycotoxins also makes quantitative measurement more variable. Thus, the accurate measurement of the types and contents of mycotoxins is required for effective monitoring and implementation of corresponding detoxification measures of mycotoxins during processing.
Cleaning
The cleaning process used in maize wet milling can remove large impurities, dust and sand. The ability of the ordinary cleaning process to remove grains contaminated with mycotoxin is limited. To reduce mycotoxin contamination from raw materials, the development of special mycotoxin sorters has attracted the attention of scientists and engineers. Improved gravity and optical sorters can effectively reduce the contents of FBs and AFs in maize (Morales et al. Citation2018; Lu et al. Citation2020; Lee, Herrman, and Yun Citation2014), but so far these sorters have not been widely used in production lines due to the sorting capacity.
Wet milling processing
SO2 steeping process
Mycotoxin distribution during wet milling was discussed above. This section focuses on the effect of chemicals on mycotoxins during wet milling. Steeping is a crucial and typical process performed in industrial wet milling using SO2 solutions at moderate temperatures (around 50 °C) for 24-48 h in a set of tanks with counter current flow. In such a series of tanks, as the steeping liquid (also called “steep water”) is pumped from one tank to the next, the concentration of SO2 increases (up to around 0.3%) (Schaarschmidt and Fauhl-Hassek Citation2021). Chemical/biological modifications or changes in binding will take place during SO2 steeping; these alterations will accelerate removal of the microorganisms and separation of the cellular components of the endosperm. At the same time steeping can significantly reduce the content of mycotoxins in maize, especially DON which migrates to water in large quantities because of its high solubility. SO2 promotes starch-protein disconnection, controls microbial multiplication, and can reduce the content of AFs in maize. The amount of degradation is related to SO2 concentration (17% of reduction rate under 0.15% SO2 and 56% of reduction rate under 0.3% SO2) (Wood Citation1982), temperature (CitationTabata et al. 1994) and time. A recent study found that 25-31 μg/kg of AFB1 in maize could be detoxified by steeping with 0.2-0.3% of H2SO3. The transformation product was identified as C17H14O9S, named AFB1-HSO3, and its toxicity was less than AFB1 (Yang et al. Citation2022). Wood (Citation1982) found that the OTA in kernels was reduced by 37% after steeping in a system that simulated commercial wet milling steeping with 0.15% SO2. However, it was also noted that 17% of the total OTA was undetected, due to degradation, binding or modification. Pujol et al. (Citation1999) showed that FB1 was not degraded/modified in pure SO2 solutions (0.2% and 0.4%, without maize kernels) over seven days at 60 °C. Nevertheless, he suggested that the combined effect of compounds in steep water released from maize kernels with SO2 might promote the decomposition or transformation of FB1. The mode of action and products from degradation of OTA and FB1 by SO2 are not well documented, therefore, further research in this area is necessary.
Other solutes are also used in steeping experiments to remove mycotoxins, such as sodium hypochlorite, sodium chlorite, hydrogen peroxide and sulfur-containing food additives (Schaarschmidt and Fauhl-Hassek Citation2021); degradation products resulting from use of these solutes are unknown or unstable and these are considered less advantageous than SO2. The optimal conditions, degradation mechanism and the safety of degradation products of different mycotoxins requires further research, to allow adaptation to complex environments containing a variety of mycotoxins.
Lactic acid bacteria fermentation
There is a natural Lactic acid bacteria (LAB) fermentation process in the initial stages of maize steeping. LAB hydrolyzes maize protein, which is conducive to protein separation and inhibition of pathogenic and spoilage microorganisms. Various strategies for mycotoxin detoxification using microorganisms such as bacteria, yeast and fungi have been widely reviewed (Wan, Chen, and Rao Citation2020). Bio-decontamination based on probiotic LAB provides an emerging and promising solution that can be applied directly in maize food products (Wang et al. Citation2019). Degradation of mycotoxins by LAB during fermentation may, therefore, have a useful application in detoxification of MGM. Diverse species of LAB have been studied for their decontamination capabilities by biodegradation and binding ().
Table 3. Mycotoxin decontamination potential of LAB in maize and maize products and related mechanisms.
The benefits of using LAB strains for decontamination of mycotoxins in maize and MGM include direct application of LAB and the low cost during maize processing; however, there are some concerns about the safety of degradation products and impact on the quality and safety of treated maize and MGM. Further research with specific LAB suitable for the processing conditions may be needed to improve the efficiency and safety of mycotoxin degradation characteristics of the chosen LAB, these may include high degradation efficiency (within maize steeping time of 24-48 h), and high-temperature resistance (steeping temperature around 50°C). It will also be necessary to analyze the safety of mycotoxin degradation products and their impact on the process. Finally, selecting LAB according to the type of mycotoxin and using compounded LAB with different decontamination functions may improve degradation efficiency.
Post-processing
Mycotoxin measurement in final products is the last line of defense to ensure food safety. The EU has set the MLs of mycotoxins in maize and maize-based products consumed by humans. The ML of AFB1 in maize and all maize products is 2 μg/kg. For processed maize-based foods that are consumed by infants and young children and dietary infant foods used for special medical purposes, it is 0.1 μg/kg. In addition, the limit of AFs (the sum of B1, B2, G1 and G2) in maize and all maize products is regulated as 4 μg/kg (European Commission (EC) Citation2006). MGM should be tested and follow the MLs to ensure safety before entering the market. Proper packaging, storage, and transportation of MGM are also necessary.
No single critical control point can achieve mycotoxin risk management in MGM. Comprehensive strategies to reduce the contamination caused by mycotoxins from the field through to the end-processed product are needed. GAP and improved processing can support the decontamination of mycotoxins in MGM by reducing the mycotoxin risk in “starting” maize. Future research will continue to address the challenges associated with the distribution of mycotoxins from maize to MGM during the wet milling process. We believe that research will enable the development of a risk assessment or predictive tool to help control mycotoxin contamination of MGM.
Emerging mycotoxin decontamination methods for maize and MGM
Mycotoxin removal and detoxification methods for cereals have been extensively researched (Mir et al. Citation2021) and mycotoxin control methods specialized for maize have been initiated and reviewed (Grenier et al. Citation2014; Munkvold et al. Citation2019). The approaches that are employed to mitigate mycotoxins in maize and maize products are commonly classified into chemical, physical, and biological methods, which act through removal or inactivation of mycotoxins. Finding safe and efficient methods to remove mycotoxins has always been the goal of researchers. Three emerging techniques for mycotoxin decontamination are discussed below which show great potential for mycotoxin control in MGM.
Emerging sorting techniques
Sorting is the most common and effective process for mycotoxin removal (Liu et al. Citation2020), although its effectiveness varies depending on context and application. Sorting enables differentiation between sub-standard or heterogeneous kernels and intact ones based on differences in density, size, shape and color. Various sorting techniques (such as hand-, color- and optical sorting) have been developed (Marshall et al. Citation2020). However, removal efficiency and accuracy of mycotoxin detection are challenges for traditional sorting machines. Advanced sorting methods embedded with emerging techniques are being studied and developed to exclude infected maize kernels.
Morales et al. (Citation2018) demonstrated that the correlation between kernel bulk density (KBD) and FBs levels in maize kernels was negative. This knowledge of the association between KBD and FBs has been used in the development of a low-priced grain sorter prototype (“DropSort”). This machine has been shown to be effective in reducing FBs concentrations to below 2 ppm, but the effectiveness in reducing AF levels in maize grain to under 20 ppb is still controversial, especially in heavily AF-contaminated grain (Aoun et al. Citation2020).
Methods that have been developed for optical sorting mainly include using ultraviolet (UV), visible or infrared wavelengths. A combination of infrared, visible, and UV light imaging is currently being used to sort contaminated maize kernels (Stasiewicz et al. Citation2017; Chavez et al. Citation2022; Cheng, Vella, and Stasiewicz Citation2019). However, these technologies have not been applied in production for maize wet milling due to failing to meet the processing capability.
Currently, research in sorting has progressed beyond detecting and removing indicators of fungal infection (such as the size, shape and color of contaminated grains), to targeting the mycotoxins directly. Fluorescence spectroscopy showed promising outcomes for sorting AF contaminated maize. In chromatographic sorting of AF contaminated maize, fluorescence emitted by aflatoxin and bright greenish yellow fluorescence emitted by kojic acid (another secondary metabolite of A. flavus and A. parasiticus) oxidation under UV light made nondestructive detection and sorting of AF contaminated grain possible (Lillehoj, Jacks, and Calvert Citation1986; Tao et al. Citation2018). The commercialization of fluorescence sorting has been promoted by the development of new technologies including hyperspectral imaging (HSI). HSI adds a spatial dimension to conventional spectroscopic techniques, enabling mapping of chemical components (also known as chemical imaging) in the tested sample, which is particularly useful for detection of unevenly distributed components (Saha and Manickavasagan Citation2021; Tao et al. Citation2018).
A method and detection system for AF in maize using fluorescence spectra has been patented (Yao et al. Citation2013). Smeesters et al. (Citation2015) used one- and two-photon induced fluorescence spectrometry to detect maize contaminated with AFs. Han et al. (Citation2019) suggested a fluorescence multispectral system with two cameras to screen AF contaminated maize. An accuracy of above 96.0% was obtained based on the fluorescence images at 436 nm and 532 nm. At present, a commercially available sorting technique uses LED light and fluorescence HSI to detect and sort contaminated maize at 15 tons per hour, with a reduction in AF contamination averaging at 85-90% in tests (Bühler Citation2018; Marshall et al. Citation2020). Another kind of laser induced fluorescence-based sorter has also started to detect AFs in incoming maize (Tomra Citation2022).
Fluorescence-based sorting devices have a sound scientific basis and research has provided a solid foundation for commercial promotion and inline application. The devices will play an important role in removing AF contaminated maize before MGM processing. The main limiting factors are cost, fluorescence intensity and image acquisition at processing speeds which affect the sorting accuracy; as a result industrial application is relatively slow (Lu et al. Citation2020). Further studies are needed to establish new modalities with faster image processing and analysis to fit with industrial scale treatment during maize wet milling for the reduction of mycotoxins risk.
Sorting is a promising technology. However, dealing with maize rejected by sorting must be considered by every responsible manufacturer and scientist and it is particularly important that rejected maize does not enter food chain. To reduce waste and environmental pollution, physical, chemical and biological decontamination strategies for rejected maize need further research (Temba et al. Citation2016; Bosch et al. Citation2017; Meijer et al. Citation2019).
Cold plasma
Cold Plasma (CP) is a potential decontamination technology that can be applied before crops enter the production process. The ability of cold plasma to inactivate the mycotoxigenic fungi (including Aspergillus spp., Fusarium spp., Penicillium spp. and Alternaria spp.) and detoxify mycotoxins (including FBs, ZEN, DON and AFs) in various food matrixes, including cereals, have been confirmed (Sharma and Singh Citation2020; Gavahian and Cullen Citation2019; Ten Bosch et al. Citation2017; Schluter et al. Citation2013).
CP has advantages over other mycotoxin reduction methods currently used in the agriculture and food industries, due to its lower cost and energy needs (Misra et al. Citation2019). One study was carried out to determine the efficacy of a self-designed Low-pressure CP device for the inactivation of Aspergillus spp. and Penicillium spp. in maize kernels; the results demonstrated a significant decrease of three log orders of magnitude for both species within 15 min (Selcuk, Oksuz, and Basaran Citation2008). Dasan, Boyaci, and Mutlu (Citation2016) performed research on the fungicidal effect of fluidized bed plasma treatment on A. flavus and A. parasiticus inoculated onto maize kernels. After a five-minute plasma treatment, a maximum reduction was observed with 5.48 and 5.20 lgCFU/g in A. flavus and A. parasiticus, respectively.
Ten Bosch et al. (Citation2017) showed that pure mycotoxins (FB1, DON, ZEN, and T2-toxin) exposed to Cold Atmospheric Pressure Plasma (CAPP) were degraded almost completely within 60 s. The degradation rate varied with mycotoxin structure and the presence of matrix slowed the process, but did not prevent degradation. Another study demonstrated high degradation of FBs (93%), ZEN (100%), AFs (93%) and T2/HT-2 (90%) after eight minutes of exposure to CAPP (Hojnik et al. Citation2019). AFs in maize could be reduced by 62% and 82% when treated with High Voltage Atmospheric Cold Plasma (HVACP) for one and 10 min, respectively. Humid air (40-80% RH) resulted in higher AFs degradation compared with dry air (5% RH), in maize treated by HVACP; there were no significant differences in AF degradation at RH between 40 and 80%. Lack of stirring of maize and difficulty in penetration of the reactive gas species into maize kernels also affected degradation (Shi et al. Citation2017). Results show that optimized CAPP (10 min of treatment) resulted in significant reduction of AFB1 (65%) and FB1 (64%) in maize. Whilst CAPP is a promising technology for mycotoxin detoxification, critical questions concerning potential changes to the nutritional and safety status of the food matrix require further investigation (Wielogorska et al. Citation2019).
CP is considered a potentially feasible technology to inactivate fungi and degrade mycotoxins in food and feed. CP can significantly reduce the toxigenic fungi and mycotoxins in maize and other food substrates and has the advantages that it has less impact on the nutrition, sensory attributes and texture of food than some other methods. It carries no risk of chemical pollution, and most of the intermediate and final degradation products are nontoxic (Adebo et al. Citation2020). However, this new technology is still in the early assessment/laboratory stage, costs are high and there is a risk of oxidation in lipid rich foods due to the existence of active gas (Guo et al. Citation2020). Due to the cost and poor permeability of dried solid material to ionized gas, CP is not readily used for detoxification of many dried maize raw materials. Wet milling provides better feasibility for using CP to degrade mycotoxins and inactivate fungi in MGM and other maize by-products, as the wet environment is more conducive to the degradation of mycotoxins. Treatment of MGM and other by-products with CP before drying may improve the degradation effect on mycotoxins. Further studies are necessary to optimize CP processing conditions for MGM and other maize by-products, including plasma type, energy, working gas, stirring, and time. Concurrent studies on the safety of mycotoxin degradation products, the interaction between CP and the food matrix (such as lipid peroxidation) will help to promote establishment of a standardized system and the commercial application of CP in MGM production.
Photocatalysis
Photocatalysis is a chemical reaction induced by the absorption of photons by a solid material (the photocatalyst). The process does not cause secondary pollutants (new pollutants by photocatalyst reacting with existing components), can be easy to operate and cost-effective, and some applications have been found in the energy and environmental fields. Decontamination of mycotoxins by photocatalysis is mature (Murugesan et al. Citation2021); for example, combining UV-visible irradiation with semiconducting photocatalysts can increase the efficiency of AF degradation in liquid matrix (Sun et al. Citation2019; Guo et al. Citation2020). During photocatalytic degradation, photo-generated valence band holes (h+), hydroxyl free radicals (OH−) and superoxide radical (O2−) can directly oxidize AFB1. The process also leads to the formation of different reactive oxygen species that help degrade AFs and convert them into less harmful derivatives (Sun et al. Citation2019; Mao, Zhang, Wang, et al. Citation2018). The most extensively researched photocatalyst is titanium dioxide (TiO2) because of its high activity under UV irradiation, high efficiency and long-term photostability (Sun et al. Citation2019; Magzoub et al. Citation2019).
The disadvantages of using TiO2 alone as a photocatalyst are that it needs to be removed from the substrate considering its potential risk to health, especially genotoxicity concerns (TiO2 banned as a food additive in the EU in 2022) (USDA Citation2022), which is a difficult process. In addition, this process requires UV irradiation to be effective (Guo et al. Citation2021). Therefore, researchers began to develop immobilization technology (Xu et al. Citation2018; Magzoub et al. Citation2019) and composite photocatalysts capable of degrading mycotoxins under visible light.
Composite photocatalysts can improve quantum efficiency, visible light response, and substrate separation. Carbon-based materials are mainly used in composite photocatalysts. Carbon-based materials increase the adsorption performance and specific surface area of AF, thus improving the efficiency of photosensitive catalysts. The degradation efficiency of an AC/TiO2 (AC stands for activated carbon) composite catalyst for AFB1 was higher than that of a single catalyst (TiO2) (Sun et al. Citation2019). Mao et al. (Citation2019), Mao, Zhang, Wang, et al. (Citation2018), Mao, Zhang, Li, et al. (Citation2018) synthesized z-scheme based ternary composites consisting of tungsten trioxide (WO3) nanowires and g-C3N4 nanosheets to enhance the photodegradation efficiency of AFB1 under visible light irradiation. Sun et al. (Citation2021) showed that a reduction of 96% of AFB1 could be achieved in maize oil using a magnetic graphene oxide/TiO2 nanocomposite after illumination for 120 min under UV-Vis light. Graphene/ZnO hybrid photocatalysts exhibited excellent activity for photodegradation of DON under UV irradiation (Bai et al. Citation2017). Other types of compound materials, such as Sc doping by SrTi0.7Fe0.3O3 (Jamil et al. Citation2017), iodine-TiO2 thin film (Xu et al. Citation2018), Clew-like WO3 decorated with nanoparticles (Mao et al. Citation2019), MGO doping by TiO2 (Popovic et al. Citation2018) had good effects on the degradation of AFB1. Upconversion nanoparticles@TiO2 composites (Wu et al. Citation2019) and NaYF4: Yb, Tm@TiO2 composites (Zhou et al. Citation2020) were effective for DON degradation.
Photocatalysis has not yet been applied to the manufacture of MGM. Nevertheless, the soaking and wet milling processes used for MGM production provide an appropriate liquid matrix for photocatalytic degradation of mycotoxins, suggesting that this may become a promising scientific and commercial field. There are still some problems to be solved for this application: i) development and utilization of new photosensitive composites. For example, the development of new nano carbon-based photosensitive materials with mycotoxin degradation under visible light has good prospects; ii) improving the efficiency of photocatalytic degradation; the most suitable type and dosage of photocatalyst should be selected according to the wet grinding soaking processing conditions, pH, toxin type and concentration, and the composition of the solution; iii) stability and safety of photosensitive catalysts and the influence of photosensitive catalysts on product quality and the safety of degradation products must be studied in detail.
It is worthwhile noting that the emerging techniques discussed above would require regulatory risk assessment and approval processes based on possible changes to the nutritional, quality and safety impacts of any by-products of the process itself.
Conclusions
Mycotoxin contamination of maize can occur pre-harvest, at harvest, during post-harvest operations and in storage. Moreover, the enrichment of mycotoxins in MGM processing increases the risk of contamination of MGM at unacceptable levels. A systematic and thorough mycotoxin risk management and decontamination strategy for MGM needs to be considered, extending from the maize field throughout processing and in research on mycotoxin decontamination in MGM ().
GAP is effective in reducing mycotoxin contamination during maize planting and storage and it is worth promoting in maize planting and production regions. Sorting before processing is also an effective approach to reduce mycotoxins by removing contaminated kernels, especially with the development of new sorting equipment; a safe alternative use or effective decontamination of the waste stream is needed to avoid unintended concentration of mycotoxin consumption by some people or animals. Wet milling can reduce the total amount of mycotoxins due to transfer to water during steeping and degrading by SO2, however, the enrichment of mycotoxins in MGM is still a disadvantageous factor. A full understanding of the migration path of mycotoxins during maize processing, and of the interaction between protein and mycotoxins, together with improvements to steeping (e.g., use of LAB), can be emphasized in future research to contribute to mycotoxin risk management in MGM.
It should be noted that the wet milling process is a mature manufacturing process that has been used for starch production for many decades. The quality and yield of starch cannot be ignored when considering any improvement for by-products such as MGM. Therefore, the method of removing mycotoxins from MGM should not increase the production burden from a commercial perspective and maintain the commercial specifications of MGM such as color, odor and nutritional composition. Technologies including the development of new sorters, the use of photocatalysts and cold plasma, show good potential within this context.
Acknowledgements
We extend our sincere thanks to Peter Markwell, Boris Bolschikov, Ahmad Tanvir, Stephen French and Cui Wang for comments and scoping that greatly improved the manuscript. We also would like to thank Cargill for supporting the technique integration, providing professional industry experience and building collaboration with the Mars Global Food Safety Center.
Disclosure statement
JH is a consultant for the Mars Global Food Safety Center.
Additional information
Funding
References
- Abbas, H. K., J. R. Wilkinson, R. M. Zablotowicz, C. Accinelli, C. A. Abel, H. A. Bruns, and M. A. Weaver. 2009. Ecology of Aspergillus flavus, regulation of aflatoxin production, and management strategies to reduce aflatoxin contamination of corn. Toxin Reviews 28 (2–3):142–53. doi: 10.1080/15569540903081590.
- Adebo, O. A., T. Molelekoa, R. Makhuvele, J. A. Adebiyi, A. B. Oyedeji, S. Gbashi, M. A. Adefisoye, O. M. Ogundele, and P. B. Njobeh. 2020. A review on novel non‐thermal food processing techniques for mycotoxin reduction. International Journal of Food Science & Technology 56 (1):13–27. doi: 10.1111/ijfs.14734.
- Adegoke, G. O., and P. Letum. 2013. Strategies for the prevention and reduction of mycotoxins in developing countries. In Mycotoxin and food safety in developing countries. eds. A. M. Hussaini. London: IntechOpen.
- Agriopoulou, S., E. Stamatelopoulou, and T. Varzakas. 2020. Advances in occurrence, importance, and mycotoxin control strategies: Prevention and detoxification in foods. Foods 9 (2):137. doi: 10.3390/foods9020137.
- Aly, S. E. 2002. Distribution of aflatoxins in product and by-products during glucose production from contaminated corn. Nahrung 46 (5):341–4. doi: 10.1002/1521-3803(20020901)46:5 < 341::AID-FOOD341 > 3.0.CO;2-N.
- Anderson, T. J., and B. P. Lamsal. 2011. Zein extraction from corn, corn products, and coproducts and modifications for various applications: A review. Cereal Chemistry 88 (2):159–73. doi: 10.1094/Cchem-06-10-0091.
- Aoun, M., W. Stafstrom, P. Priest, J. Fuchs, G. L. Windham, W. P. Williams, and R. J. Nelson. 2020. Low-cost grain sorting technologies to reduce mycotoxin contamination in maize and groundnut. Food Control 118:107363. doi: 10.1016/j.foodcont.2020.107363.
- Bai, X. J., C. P. Sun, D. Liu, X. H. Luo, D. Li, J. Wang, N. X. Wang, X. J. Chang, R. L. Zong, and Y. F. Zhu. 2017. Photocatalytic degradation of deoxynivalenol using graphene/ZnO hybrids in aqueous suspension. Applied Catalysis B-Environmental 204:11–20. doi: 10.1016/j.apcatb.2016.11.010.
- Baker, R. C., R. M. Ford, M. E. Helander, J. Marecki, R. Natarajan, and B. Ray. 2014. Framework for managing mycotoxin risks in the food industry. Journal of Food Protection 77 (12):2181–8. doi: 10.4315/0362-028x.Jfp-14-060.
- Bandyopadhyay, R., J. Atehnkeng, A. Ortega-Beltran, A. Akande, T. D. O. Falade, and P. J. Cotty. 2019. “Ground-truthing” efficacy of biological control for aflatoxin mitigation in farmers’ fields in Nigeria: From field trials to commercial usage, a 10-year study. Frontiers in Microbiology 10:2528. doi: 10.3389/fmicb.2019.02528.
- Battilani, P., P. Toscano, H. J. Van der Fels-Klerx, A. Moretti, M. Camardo Leggieri, C. Brera, A. Rortais, T. Goumperis, and T. Robinson. 2016. Aflatoxin B1 contamination in maize in Europe increases due to climate change. Scientific Reports 6:24328. doi: 10.1038/srep24328.
- Bennett, G. A., and R. A. Anderson. 1978. Distribution of aflatoxin and/or zearalenone in wet-milled corn products: A review. Journal of Agricultural and Food Chemistry 26 (5):1055–60. doi: 10.1021/jf60219a027.
- Bhatnagar-Mathur, P., S. Sunkara, M. Bhatnagar-Panwar, F. Waliyar, and K. K. Sharma. 2015. Biotechnological advances for combating Aspergillus flavus and aflatoxin contamination in crops. Plant Science: An International Journal of Experimental Plant Biology 234:119–32. doi: 10.1016/j.plantsci.2015.02.009.
- BIOMIN. 2019. BIOMIN world mycotoxin survey report. https://www.biomin.net/downloads/2019-biomin-world-mycotoxin-survey-report/.
- BIOMIN. 2020. BIOMIN world mycotoxin survey report. https://www.biomin.net/downloads/2020-biomin-world-mycotoxin-survey-report/.
- Blasi, D. A., F. Drouillard, M. J. Brouk, and S. P. Montgomery. 2001. Corn gluten feed, composition and feeding value for beef and dairy cattle. Manhattan: Kansas State University. https://bookstore.ksre.ksu.edu/pubs/MF2488.pdf.
- Boedeker, W., M. Watts, P. Clausing, and E. Marquez. 2020. The global distribution of acute unintentional pesticide poisoning: Estimations based on a systematic review. BMC Public Health 20 (1):1875. doi: 10.1186/s12889-020-09939-0.
- Bosch, G., H. J. V. Fels-Klerx, T. C. Rijk, and D. Oonincx. 2017. Aflatoxin B1 tolerance and accumulation in black soldier fly larvae (Hermetia illucens) and yellow mealworms (Tenebrio molitor). Toxins (Basel) 9 (6):185. doi: 10.3390/toxins9060185.
- Brander, M., T. Bernauer, and M. Huss. 2021. Improved on-farm storage reduces seasonal food insecurity of smallholder farmer households – Evidence from a randomized control trial in Tanzania. Food Policy. 98:101891. doi: 10.1016/j.foodpol.2020.101891.
- Bühler. 2018. Bühler LumoVision: Saving lives and improving livelihoods with revolutionary data-driven grain sorting technology. https://www.buhlergroup.com/content/buhlergroup/global/en/media/media-releases/buehler_lumovisionsavinglivesandimprovinglivelihoodswithrevoluti.html.
- Carbas, B., D. Simoes, A. Soares, A. Freitas, B. Ferreira, A. R. F. Carvalho, A. S. Silva, T. Pinto, E. Diogo, E. Andrade, et al. 2021. Occurrence of Fusarium spp. in maize grain harvested in Portugal and accumulation of related mycotoxins during storage. Foods 10 (2):375. doi: 10.3390/foods10020375.
- Channaiah, L. H., and D. E. Maier. 2014. Best stored maize management practices for the prevention of mycotoxin contamination. In Mycotoxin reduction in grain chains, eds. F. L. John, and F. L. Antonio, 78–88. New Jersey, NJ: John Wiley & Sons, Inc.
- Chauhan, Y., J. Tatnell, S. Krosch, J. Karanja, B. Gnonlonfin, I. Wanjiku, J. Wainaina, and J. Harvey. 2015. An improved simulation model to predict pre-harvest aflatoxin risk in maize. Field Crops Research 178:91–9. doi: 10.1016/j.fcr.2015.03.024.
- Chavez, R. A., X. Cheng, T. J. Herrman, and M. J. Stasiewicz. 2022. Single kernel aflatoxin and fumonisin contamination distribution and spectral classification in commercial corn. Food Control 131:108393. doi: 10.1016/j.foodcont.2021.108393.
- Chelule, P. K., H. P. Mbongwa, S. Carries, and N. Gqaleni. 2010. Lactic acid fermentation improves the quality of amahewu, a traditional South African maize-based porridge. Food Chemistry 122 (3):656–61. doi: 10.1016/j.foodchem.2010.03.026.
- Cheng, X. B., A. Vella, and M. J. Stasiewicz. 2019. Classification of aflatoxin contaminated single corn kernels by ultraviolet to near infrared spectroscopy. Food Control 98:253–61. doi: 10.1016/j.foodcont.2018.11.037.
- Chulze, S. N. 2010. Strategies to reduce mycotoxin levels in maize during storage: A review. Food Additives & Contaminants. Part A, Chemistry, Analysis, Control, Exposure & Risk Assessment 27 (5):651–7. doi: 10.1080/19440040903573032.
- Collins, G. J., and J. D. Rosen. 1981. Distribution of T-2 toxin in wet-milled corn products. Journal of Food Science 46 (3):877–9.
- Dasan, B. G., I. H. Boyaci, and M. Mutlu. 2016. Inactivation of aflatoxigenic fungi (Aspergillus spp.) on granular food model, maize, in an atmospheric pressure fluidized bed plasma system. Food Control 70:1–8. doi: 10.1016/j.foodcont.2016.05.015.
- Dawlal, P., C. Brabet, M. S. Thantsha, and E. M. Buys. 2017. Potential of lactic acid bacteria for the reduction of fumonisin exposure in African fermented maize based foods. World Mycotoxin Journal 10 (4):309–18. doi: 10.3920/Wmj2017.2184.
- Demissie, N. 2018. A review of aflatoxin: Occurrence, prevention, and gaps in both food and feed safety. Novel Techniques in Nutrition & Food Science 1 (3):190–7. doi: 10.31031/ntnf.2018.01.000511.
- Dubey, S., S. Zacharia, S. Simon, and N. Singh. 2020. Efficacy of plant extracts and Trichoderma viride against leaf spot of maize caused by Curvularia lunata. International Journal of Current Microbiology and Applied Sciences 9 (5):495–500. doi: 10.20546/ijcmas.2020.905.055.
- Dula, M. W. 2019. Review on development and performance evaluation of maize sheller. International Journal of Engineering Research & Technology 8 (05):471–481. doi: 10.17577/ijertv8is050329.
- Erenstein, O., M. Jaleta, K. Sonder, K. Mottaleb, and B. M. Prasanna. 2022. Global maize production, consumption and trade: Trends and R&D implications. Food Security 14 (5):1295–319. doi: 10.1007/s12571-022-01288-7.
- Erickson, G. E., T. J. Klopfenstein, and A. K. Watson. 2012. Utilization of feed co-products from wet or dry milling for beef cattle. In Biofuel co-products as livestock feed – Opportunities and challenges, eds. H. P. S. Makkar, 77-99. Rome: Food and Agriculture Organization of the United Nations (FAO).
- European Commission (EC). 2006. No.1881/2006: Setting maximum levels for certain contaminants in foodstuffs. https://www.legislation.gov.uk/eur/2006/1881.
- European Commission (EC). 2007. No.1126/2007: Setting maximum levels for certain contaminants in foodstuffs as regards Fusarium toxins in maize and maize products. https://www.legislation.gov.uk/eur/2007/1126/contents.
- FAO 1989. Drying and chemical treatment of grains to prevent mycotoxin contamination during storage. https://www.fao.org/3/x5036e/x5036E0w.htm.
- FAO. 1995. General standard for contaminants and toxins in food and feed. https://www.fao.org/input/download/standards/17/CXS_193e_2015.pdf.
- FAO. 2003. Development of a framework for good agricultural practices. Committee on Agriculture. https://www.fao.org/3/Y8704e/Y8704e.htm.
- FAO. 2004. Worldwide regulations for mycotoxins in food and feed in 2003. Rome, Italy: Food and Agriculture Organization of the United Nations. https://www.fao.org/3/y5499e/y5499e00.htm.
- FAOSTAT. 2021. Food and agriculture data. Accessed May 6, 2021. http://www.fao.org/faostat/en/#data/QC.
- Garcia-Diaz, M., J. Gil-Serna, C. Vazquez, M. N. Botia, and B. Patino. 2020. A Comprehensive Study on the occurrence of mycotoxins and their producing fungi during the maize production cycle in Spain. Microorganisms 8 (1):141. doi: 10.3390/microorganisms8010141.
- Gavahian, M., and P. J. Cullen. 2019. Cold plasma as an emerging technique for mycotoxin-free food: Efficacy, mechanisms, and trends. Food Reviews International 36 (2):193–214. doi: 10.1080/87559129.2019.1630638.
- Giorni, P., T. Bertuzzi, and P. Battilani. 2019. Impact of fungi co-occurrence on mycotoxin contamination in maize during the growing season. Frontiers in Microbiology 10:1265. doi: 10.3389/fmicb.2019.01265.
- Grenier, B., A.-P. Loureiro-Bracarense, J. F. Leslie, and I. P. Oswald. 2014. Physical and chemical methods for mycotoxin decontamination in maize. In Mycotoxin reduction in grain chains, eds. F. L. John and F. L. Antonio, 116–29. New Jersey, NJ: John Wiley & Sons, Inc.
- Gruber-Dorninger, C., T. Jenkins, and G. Schatzmayr. 2018. Multi-mycotoxin screening of feed and feed raw materials from Africa. World Mycotoxin Journal 11 (3):369–83. doi: 10.3920/Wmj2017.2292.
- Gruber-Dorninger, C., T. Jenkins, and G. Schatzmayr. 2019. Global mycotoxin occurrence in feed: A ten-year survey. Toxins (Basel) 11 (7):375. doi: 10.3390/toxins11070375.
- Guo, H., J. Ji, J. S. Wang, and X. Sun. 2020. Deoxynivalenol: Masked forms, fate during food processing, and potential biological remedies. Comprehensive Reviews in Food Science and Food Safety 19 (2):895–926. doi: 10.1111/1541-4337.12545.
- Guo, Y., L. Zhao, Q. Ma, and C. Ji. 2021. Novel strategies for degradation of aflatoxins in food and feed: A review. Food Research International (Ottawa, Ont.) 140:109878. doi: 10.1016/j.foodres.2020.109878.
- Gupta, R. C. 2007. Basic and clinical principles of veterinary toxicology. In Veterinary Toxicology, eds. C. G. Ramesh, 939–1018. New York: Elsevier.
- Han, D., H. Yao, Z. Hruska, R. Kincaid, K. Rajasekaran, and D. Bhatnagar. 2019. Development of high-speed dual-camera system for batch screening of aflatoxin contamination of corn using multispectral fluorescence imaging. Transactions of the ASABE 62 (2):381–91. doi: 10.13031/trans.13125.
- Harvey, J., M. Macdefette, S. Mutiga, J. M. Mutuku, T. Eldridge, P. Emmrich, D. Grace, S. Senay, A. Abate, R. Darnell, et al. 2016. Poisoned Chalice: Toxin accumulation in crops in the era of climate change. In Unfp Frontiers 2016 Report, 54–62. Nairobi: United Nations Environment Programme.
- Hell, K., P. Fandohan, R. Bandyopadhyay, K. Cardwell, S. Kiewnick, R. Sikora, and P. Cotty. 2008. Pre- and postharvest management of aflatoxin in maize: An African perspective. African Journal of Microbiology Research 19:219–229.
- Hendel, E. G., P. N. Gott, G. R. Murugesan, and T. Jenkins. 2017. 033 Survey of mycotoxins in 2016 United States corn. Journal of Animal Science 95 (suppl_4):16–7. doi: 10.2527/asasann.2017.033.
- Hojnik, N., M. Modic, G. Tavcar-Kalcher, J. Babic, J. L. Walsh, and U. Cvelbar. 2019. Mycotoxin decontamination efficacy of atmospheric pressure air plasma. Toxins (Basel) 11 (4):219. doi: 10.3390/toxins11040219.
- Jamil, T. S., H. A. Abbas, R. A. Nasr, A. A. El-Kady, and M. I. M. Ibrahim. 2017. Detoxification of aflatoxin B1 using nano-sized Sc-doped SrTi0.7 Fe0.3O3 under visible light. Journal of Photochemistry and Photobiology A: Chemistry 341:127–35. doi: 10.1016/j.jphotochem.2017.03.023.
- JECFA. 2017. Evaluation of certain contaminants in food: Eighty-third report of the Joint FAO/WHO Expert Committee on Food Additives. World Health Organization, Geneva.
- Joutsjoki, V. V., and H. J. Korhonen. 2021. Management strategies for aflatoxin risk mitigation in maize, dairy feeds and milk value chains—case study Kenya. Food Quality and Safety 5(1):1–11.
- Kos, J., E. J. Hajnal, B. Šarić, P. Jovanov, N. Nedeljković, I. Milovanović, and J. Krulj. 2017. The influence of climate conditions on the occurrence of deoxynivalenol in maize harvested in Serbia during 2013–2015. Food Control 73:734–40. doi: 10.1016/j.foodcont.2016.09.022.
- Lauren, D. R., and M. A. Ringrose. 1997. Determination of the fate of three Fusarium mycotoxins through wet-milling of maize using an improved HPLC analytical technique. Food Additives and Contaminants 14 (5):435–43. doi: 10.1080/02652039709374549.
- Lee, K. M., T. J. Herrman, and U. Yun. 2014. Application of Raman spectroscopy for qualitative and quantitative analysis of aflatoxins in ground maize samples. Journal of Cereal Science 59 (1):70–8. doi: 10.1016/j.jcs.2013.10.004.
- Leite, M., A. Freitas, A. S. Silva, J. Barbosa, and F. Ramos. 2021. Maize food chain and mycotoxins: A review on occurrence studies. Trends in Food Science & Technology 115:307–31. doi: 10.1016/j.tifs.2021.06.045.
- Li, G., W. Liu, Y. Wang, F. Jia, Y. Wang, Y. Ma, R. Gu, and J. Lu. 2019. Functions and applications of bioactive peptides from corn gluten meal. Advances in Food and Nutrition Research 87:1–41. doi: 10.1016/bs.afnr.2018.07.001.
- Lillehoj, E. B., T. J. Jacks, and O. H. Calvert. 1986. Aflatoxin estimation in corn by measurement of bright Greenish-Yellow fluorescence in aqueous extracts. Journal of Food Protection 49 (8):623–6. doi: 10.4315/0362-028X-49.8.623.
- Liu, Y., J. H. Galani Yamdeu, Y. Y. Gong, and C. Orfila. 2020. A review of postharvest approaches to reduce fungal and mycotoxin contamination of foods. Comprehensive Reviews in Food Science and Food Safety 19 (4):1521–60. doi: 10.1111/1541-4337.12562.
- Lu, Y. Z., W. Saeys, M. Kim, Y. K. Peng, and R. F. Lu. 2020. Hyperspectral imaging technology for quality and safety evaluation of horticultural products: A review and celebration of the past 20-year progress. Postharvest Biology and Technology 170:111318. doi: 10.1016/j.postharvbio.2020.111318.
- Magzoub, R. A. M., A. A. A. Yassin, A. M. Abdel-Rahim, E. A. Gubartallah, M. Miskam, B. Saad, and S. Sabar. 2019. Photocatalytic detoxification of aflatoxins in Sudanese peanut oil using immobilized titanium dioxide. Food Control 95:206–14. doi: 10.1016/j.foodcont.2018.08.009.
- Mahdjoubi, C. K., N. Arroyo-Manzanares, N. Hamini-Kadar, A. M. Garcia-Campana, K. Mebrouk, and L. Gamiz-Gracia. 2020. Multi-mycotoxin occurrence and exposure assessment approach in foodstuffs from Algeria. Toxins (Basel) 12 (3):194. doi: 10.3390/toxins12030194.
- Mahuku, G., H. S. Nzioki, C. Mutegi, F. Kanampiu, C. Narrod, and D. Makumbi. 2019. Pre-harvest management is a critical practice for minimizing aflatoxin contamination of maize. Food Control 96:219–26. doi: 10.1016/j.foodcont.2018.08.032.
- Manu, N., G. P. Opit, E. A. Osekre, F. H. Arthur, G. Mbata, P. Armstrong, J. K. Danso, S. G. McNeill, and J. F. Campbell. 2019. Moisture content, insect pest infestation and mycotoxin levels of maize in markets in the northern region of Ghana. Journal of Stored Products Research 80:10–20. doi: 10.1016/j.jspr.2018.10.007.
- Mao, J., L. X. Zhang, H. T. Wang, Q. Zhang, W. Zhang, and P. W. Li. 2018. Facile fabrication of nanosized graphitic carbon nitride sheets with efficient charge separation for mitigation of toxic pollutant. Chemical Engineering Journal 342:30–40. doi: 10.1016/j.cej.2018.02.076.
- Mao, J., P. W. Li, J. M. Wang, H. T. Wang, Q. Zhang, L. X. Zhang, H. Li, W. Zhang, and T. Y. Peng. 2019. Insights into photocatalytic inactivation mechanism of the hypertoxic site in aflatoxin B1 over clew-like WO3 decorated with CdS nanoparticles. Applied Catalysis B-Environmental 248:477–86. doi: 10.1016/j.apcatb.2019.01.057.
- Mao, J., Q. Zhang, P. W. Li, L. X. Zhang, and W. Zhang. 2018. Geometric architecture design of ternary composites based on dispersive WO3 nanowires for enhanced visible-light-driven activity of refractory pollutant degradation. Chemical Engineering Journal 334:2568–78. doi: 10.1016/j.cej.2017.10.165.
- Marshall, H., J. P. Meneely, B. Quinn, Y. J. Zhao, P. Bourke, B. F. Gilmore, G. T. Zhang, and C. T. Elliott. 2020. Novel decontamination approaches and their potential application for post-harvest aflatoxin control. Trends in Food Science & Technology 106:489–96. doi: 10.1016/j.tifs.2020.11.001.
- Massomo, S. M. S. 2020. Aspergillus flavus and aflatoxin contamination in the maize value chain and what needs to be done in Tanzania. Scientific African 10:e00606. doi: 10.1016/j.sciaf.2020.e00606.
- Meijer, N., G. M. Stoopen, H. J. van der Fels-Klerx, J. J. A. van Loon, J. Carney, and G. Bosch. 2019. Aflatoxin B1 conversion by black soldier fly (Hermetia illucens) larval enzyme extracts. Toxins 11 (9):532. doi: 10.3390/toxins11090532.
- Mir, S., A. B. N. Dar, M. A. Shah, S. A. Sofi, A. M. Hamdani, C. A. F. Oliveira, M. Hashemi Moosavi, A. Mousavi Khaneghah, and A. S. Sant’Ana. 2021. Application of new technologies in decontamination of mycotoxins in cereal grains: Challenges, and perspectives. Food and Chemical Toxicology : An International Journal Published for the British Industrial Biological Research Association 148:111976. doi: 10.1016/j.fct.2021.111976.
- Misra, N. N., B. Yadav, M. S. Roopesh, and C. Jo. 2019. Cold plasma for effective fungal and mycotoxin control in foods: Mechanisms, inactivation effects, and applications. Comprehensive Reviews in Food Science and Food Safety 18 (1):106–20. doi: 10.1111/1541-4337.12398.
- Mitchell, N. J., E. Bowers, C. Hurburgh, and F. Wu. 2016. Potential economic losses to the US corn industry from aflatoxin contamination. Food Additives & Contaminants. Part A, Chemistry, Analysis, Control, Exposure & Risk Assessment 33 (3):540–50. doi: 10.1080/19440049.2016.1138545.
- Mokoena, M. P., P. K. Chelule, and N. Gqaleni. 2005. Reduction of fumonisin B1 and zearalenone by lactic acid bacteria in fermented maize meal. Journal of Food Protection 68 (10):2095–9. doi: 10.4315/0362-028x-68.10.2095.
- Monda, E., J. Masanga, and A. Alakonya. 2020. Variation in occurrence and aflatoxigenicity of Aspergillus flavus from two climatically varied regions in Kenya. Toxins (Basel) 12 (1):34. doi: 10.3390/toxins12010034.
- Morales, L., T. P. Marino, A. J. Wenndt, J. Q. Fouts, J. B. Holland, and R. J. Nelson. 2018. Dissecting symptomatology and fumonisin contamination produced by Fusarium verticillioides in maize ears. Phytopathology 108 (12):1475–85. doi: 10.1094/PHYTO-05-18-0167-R.
- Moretti, A., M. Pascale, and A. F. Logrieco. 2019. Mycotoxin risks under a climate change scenario in Europe. Trends in Food Science & Technology 84:38–40. doi: 10.1016/j.tifs.2018.03.008.
- Munkvold, G. P. 2003. Cultural and genetic approaches to managing mycotoxins in maize. Annual Review of Phytopathology 41:99–116. doi: 10.1146/annurev.phyto.41.052002.095510.
- Munkvold, G. P., S. Arias, I. Taschl, and C. Gruber-Dorninger. 2019. Mycotoxins in corn: Occurrence, impacts, and management. In Corn, ed. S. O. Serna-Saldivar, 235–87. Oxford: AACC International Press.
- Murugesan, P., D. K. Brunda, J. A. Moses, and C. Anandharamakrishnan. 2021. Photolytic and photocatalytic detoxification of mycotoxins in foods. Food Control 123 (3):107748. doi: 10.1016/j.foodcont.2020.107748.
- Nazareth, T. d M., C. Luz, R. Torrijos, J. M. Quiles, F. B. Luciano, J. Mañes, and G. Meca. 2020. Potential application of lactic acid bacteria to reduce aflatoxin B1 and fumonisin B1 occurrence on corn kernels and corn ears. Toxins 12 (1):21. doi: 10.3390/toxins12010021.
- Neaj, K., K. Habschied, and K. Mastanjevj. 2021. Possibilities for the biological control of mycotoxins in food and feed. Toxins 13 (3):198. doi: 10.3390/toxins13030198.
- Niderkorn, V., D. P. Morgavi, E. Pujos, A. Tissandier, and H. Boudra. 2007. Screening of fermentative bacteria for their ability to bind and biotransform deoxynivalenol, zearalenone and fumonisins in an in vitro simulated corn silage model. Food Additives and Contaminants 24 (4):406–15. doi: 10.1080/02652030601101110.
- Nyamete, F. A., B. Mourice, and J. K. Mugula. 2016. Fumonisin B1 reduction in lactic acid bacteria fermentation of maize porridges. Tanzania Journal of Agricultural Sciences 15 (1):13–201.
- Nyamete, F. A., M. Bennink, and J. K. Mugula. 2016. Potential of lactic acid fermentation in reducing aflatoxin B1 in Tanzania maize-based gruel. African Journal of Food, Agriculture, Nutrition and Development 16 (3):11139–51. doi: 10.18697/ajfand.75.ILRI12.
- Okeke, C. A., C. N. Ezekiel, C. C. Nwangburuka, M. Sulyok, C. O. Ezeamagu, R. A. Adeleke, S. K. Dike, and R. Krska. 2015. Bacterial diversity and mycotoxin reduction during maize fermentation (steeping) for ogi production. Frontiers in Microbiology 6:1402. doi: 10.3389/fmicb.2015.01402.
- Okoth, S., B. Nyongesa, V. Ayugi, E. Kang’ethe, H. Korhonen, and V. Joutsjoki. 2012. Toxigenic potential of Aspergillus species occurring on maize kernels from two agro-ecological zones in Kenya. Toxins 4 (11):991–1007. doi: 10.3390/toxins4110991.
- Oluwafemi, F., and F. A. Da-Silva. 2009. Removal of aflatoxins by viable and heat-killed Lactobacillus species isolated from fermented maize. Journal of Applied Biosciences 16:871–6.
- Oluwafemi, F., M. Kumar, R. Bandyopadhyay, T. Ogunbanwo, and K. B. Ayanwande. 2010. Bio-detoxification of aflatoxin B1 in artificially contaminated maize grains using lactic acid bacteria. Toxin Reviews 29 (3–4):115–22. doi: 10.3109/15569543.2010.512556.
- Ozbey, F., and B. Kabak. 2012. Natural co-occurrence of aflatoxins and ochratoxin A in spices. Food Control 28 (2):354–61. doi: 10.1016/j.foodcont.2012.05.039.
- Park, J., D. H. Kim, J. Y. Moon, J. A. An, Y. W. Kim, S. H. Chung, and C. Lee. 2018. Distribution analysis of twelve mycotoxins in corn and corn-derived products by LC-MS/MS to evaluate the carry-over ratio during wet-milling. Toxins (Basel) 10 (8):319. doi: 10.3390/toxins10080319.
- Pasupuleti, V. K., and A. L. Demain. 2010. Protein hydrolysates in biotechnology. Springer, Dordrecht.
- Paterson, R., M. Russell, and N. Lima. 2010. How will climate change affect mycotoxins in food? Food Research International 43 (7):1902–14. doi: 10.1016/j.foodres.2009.07.010.
- Pereira, V. L., J. O. Fernandes, and S. C. Cunha. 2014. Mycotoxins in cereals and related foodstuffs: A review on occurrence and recent methods of analysis. Trends in Food Science & Technology 36 (2):96–136. doi: 10.1016/j.tifs.2014.01.005.
- Perrone, G., M. Ferrara, A. Medina, M. Pascale, and N. Magan. 2020. Toxigenic fungi and mycotoxins in a climate change scenario: Ecology, genomics, distribution, prediction and prevention of the risk. Microorganisms 8 (10):1496. doi: 10.3390/microorganisms8101496.
- Pitt, J. I. 2019. The pros and cons of using biocontrol by competitive exclusion as a means for reducing aflatoxin in maize in Africa. World Mycotoxin Journal 12 (2):103–12. doi: 10.3920/WMJ2018.2410.
- Pitt, J. I., C. Manthong, P. Siriacha, S. Chotechaunmanirat, and P. J. Markwell. 2015. Studies on the biocontrol of aflatoxin in maize in Thailand. Biocontrol Science and Technology 25 (9):1070–91. doi: 10.1080/09583157.2015.1028893.
- Pleadin, J., N. Persi, M. Mitak, M. Zadravec, M. Sokolovic, A. Vulic, V. Jaki, and M. Brstilo. 2012. The natural occurrence of T-2 toxin and fumonisins in maize samples in Croatia. Bulletin of Environmental Contamination and Toxicology 88 (6):863–6. doi: 10.1007/s00128-012-0559-1.
- Popovic, V., N. Fairbanks, J. Pierscianowski, M. Biancaniello, T. Zhou, and T. Koutchma. 2018. Feasibility of 3D UV-C treatment to reduce fungal growth and mycotoxin loads on maize and wheat kernels. Mycotoxin Research 34 (3):211–21. doi: 10.1007/s12550-018-0316-3.
- Porricelli, A. C. R., V. Lippolis, S. Valenzano, M. Cortese, M. Suman, S. Zanardi, and M. Pascale. 2016. Optimization and validation of a fluorescence polarization immunoassay for rapid detection of T-2 and HT-2 toxins in cereals and cereal-based products. Food Analytical Methods 9 (12):3310–8. doi: 10.1007/s12161-016-0527-1.
- Pujol, R., M. Torres, V. Sanchis, and R. Canela. 1999. Fate of fumonisin B1 in corn kernel steeping water containing SO2. Journal of Agricultural and Food Chemistry 47 (1):276–8. doi: 10.1021/jf9805045.
- Ramirez-Cabral, N. Y. Z., L. Kumar, and F. Shabani. 2017. Global alterations in areas of suitability for maize production from climate change and using a mechanistic species distribution model (CLIMEX). Scientific Reports 7 (1):5910. doi: 10.1038/s41598-017-05804-0.
- Ranum, P., J. P. Peña‐Rosas, and M. N. Garcia‐Casal. 2014. Global maize production, utilization, and consumption. Annals of the New York Academy of Sciences 1312 (1):105–12. Epub 2014 Mar 20. doi: 10.1111/nyas.12396.
- Rausch, K. D., D. Hummel, L. A. Johnson, and J. B. May. 2019. Wet milling: The basis for corn biorefineries. In Corn, ed. S. O. Serna-Saldivar, 501–35. Oxford: AACC International Press.
- RFA. 2008. Feeding the future: The role of the US ethanol industry in food and feed production. Renewable Fuels Association, Washington DC. https://feedipedia.org/node/13763.
- Ribeiro, K. D. O., M. C. Garcia, A. R. Oliveira, M. S. Soares Júnior, and M. Caliri. 2019. Characterization and proposal of potential use in foods of coproducts from waxy maize wet milling. Food Science and Technology 39 (2):315–320.
- Richard, E., N. Heutte, L. Sage, D. Pottier, V. Bouchart, P. Lebailly, and D. Garon. 2007. Toxigenic fungi and mycotoxins in mature corn silage. Food and Chemical Toxicology : An International Journal Published for the British Industrial Biological Research Association 45 (12):2420–5. doi: 10.1016/j.fct.2007.06.018.
- Rodrigues, I., and K. Naehrer. 2012. A three-year survey on the worldwide occurrence of mycotoxins in feedstuffs and feed. Toxins 4 (9):663–75. doi: 10.3390/toxins4090663.
- Romer. 1984. Detecting mycotoxins in corn and corn-milling products. Feedstuffs: The Weekly Newspaper for Agribusiness 56 (37):22–3.
- Romer. 2006. Vol. 2: Sampling and sample preparation for mycotoxin analysis. Romer Labs® Guide to Mycotoxins.
- Saha, D., and A. Manickavasagan. 2021. Machine learning techniques for analysis of hyperspectral images to determine quality of food products: A review. Current Research in Food Science 4:28–44. doi: 10.1016/j.crfs.2021.01.002.
- Schaarschmidt, S., and C. Fauhl-Hassek. 2021. The fate of mycotoxins during the primary food processing of maize. Food Control 121:107651. doi: 10.1016/j.foodcont.2020.107651.
- Schluter, O., J. Ehlbeck, C. Hertel, M. Habermeyer, A. Roth, K. H. Engel, T. Holzhauser, D. Knorr, and G. Eisenbrand. 2013. Opinion on the use of plasma processes for treatment of foods. Molecular Nutrition & Food Research 57 (5):920–7. doi: 10.1002/mnfr.201300039.
- Selcuk, M., L. Oksuz, and P. Basaran. 2008. Decontamination of grains and legumes infected with Aspergillus spp. and Penicillum spp. by cold plasma treatment. Bioresource Technology 99 (11):5104–9. doi: 10.1016/j.biortech.2007.09.076.
- Setamou, M., K. F. Cardwell, F. Schulthess, and K. Hell. 1998. Effect of insect damage to maize ears, with special reference to Mussidia nigrivenella (Lepidoptera: Pyralidae), on Aspergillus flavus (Deuteromycetes: Monoliales) infection and aflatoxin production in maize before harvest in the republic of Benin. Journal of Economic Entomology 91 (2):433–8. doi: 10.1093/jee/91.2.433.
- Sharma, S., and R. K. Singh. 2020. Cold plasma treatment of dairy proteins in relation to functionality enhancement. Trends in Food Science & Technology 102:30–6. doi: 10.1016/j.tifs.2020.05.013.
- Shi, H., K. Ileleji, R. L. Stroshine, K. Keener, and J. L. Jensen. 2017. Reduction of aflatoxin in corn by high voltage atmospheric cold plasma. Food and Bioprocess Technology 10 (6):1042–52. doi: 10.1007/s11947-017-1873-8.
- Singano, C. D., B. M. Mvumi, and T. E. Stathers. 2019. Effectiveness of grain storage facilities and protectants in controlling stored-maize insect pests in a climate-risk prone area of Shire Valley, Southern Malawi. Journal of Stored Products Research 83:130–47. doi: 10.1016/j.jspr.2019.06.007.
- Smeesters, L., W. Meulebroeck, S. Raeymaekers, and H. Thienpont. 2015. Optical detection of aflatoxins in maize using one- and two-photon induced fluorescence spectroscopy. Food Control 51:408–16. doi: 10.1016/j.foodcont.2014.12.003.
- Stasiewicz, M. J., T. D. O. Falade, M. Mutuma, S. K. Mutiga, J. J. W. Harvey, G. Fox, T. C. Pearson, J. W. Muthomi, and R. J. Nelson. 2017. Multi-spectral kernel sorting to reduce aflatoxins and fumonisins in Kenyan maize. Food Control 78:203–14. doi: 10.1016/j.foodcont.2017.02.038.
- Suleiman, R., C. J. Bern, T. J. Brumm, and K. A. Rosentrater. 2018. Impact of moisture content and maize weevils on maize quality during hermetic and non-hermetic storage. Journal of Stored Products Research 78:1–10. doi: 10.1016/j.jspr.2018.05.007.
- Sun, S., R. Zhao, Y. Xie, and Y. Liu. 2019. Photocatalytic degradation of aflatoxin B1 by activated carbon supported TiO2 catalyst. Food Control 100:183–8. doi: 10.1016/j.foodcont.2019.01.014.
- Sun, S., R. Zhao, Y. Xie, and Y. Liu. 2021. Reduction of aflatoxin B1 by magnetic graphene oxide/TiO2 nanocomposite and its effect on quality of corn oil. Food Chemistry 343:128521. doi: 10.1016/j.foodchem.2020.128521.
- Tabata, S., H. Kamimura, A. Ibe, H. Hashimoto, and Y. Tamura. 1994. Degradation of aflatoxins by food additives. Journal of Food Protection 57 (1):42–7. doi: 10.4315/0362-028X-57.1.42.
- Tan, H. X., H. Y. Zhou, T. Guo, Y. H. Zhang, and L. Ma. 2021. Zein-bound zearalenone: A hidden mycotoxin found in maize and maize-products. Food Control 124 (2021):107903. doi: 10.1016/j.foodcont.2021.107903.
- Tao, F. F., H. B. Yao, Z. Hruiska, L. W. Burger, K. Rajasekaran, and D. Bhatnagar. 2018. Recent development of optical methods in rapid and non-destructive detection of aflatoxin and fungal contamination in agricultural products. Trac-Trends in Analytical Chemistry 100:65–81. doi: 10.1016/j.trac.2017.12.017.
- Tarazona, A., J. V. Gomez, F. Mateo, M. Jimenez, D. Romera, and E. M. Mateo. 2020. Study on mycotoxin contamination of maize kernels in Spain. Food Control 118 (2020):107370. doi: 10.1016/j.foodcont.2020.107370.
- Temba, B. A., Y. Sultanbawa, D. J. Kriticos, G. P. Fox, J. J. Harvey, and M. T. Fletcher. 2016. Tools for defusing a major global food and feed safety risk: Nonbiological postharvest procedures to decontaminate mycotoxins in foods and feeds. Journal of Agricultural and Food Chemistry 64 (47):8959–72. doi: 10.1021/acs.jafc.6b03777.
- Ten Bosch, L., K. Pfohl, G. Avramidis, S. Wieneke, W. Viöl, and P. Karlovsky. 2017. Plasma-based degradation of mycotoxins produced by Fusarium, Aspergillus and Alternaria species. Toxins 9 (3) 97. doi: 10.3390/toxins9030097.
- Thai Agriculture Standard. 2010. Good agricultural practices for maize. Bangkok: National Bureau of Agricultural Commodity and Food Standards; Ministry of Agriculture and Cooperatives.
- Tomra. 2022. Sorting machines for pet food and rendering. TOMRA System ASA. https://www.tomra.com/en/solutions/food/proteins/petfood-and-rendering
- Torovic, L. 2018. Aflatoxins and ochratoxin A in flour: A survey of the Serbian retail market. Food Additives and Contaminants: Part B Surveillance 11 (1):26–32. doi: 10.1080/19393210.2017.1391335.
- Tran-Dinh, N., J. I. Pitt, and P. J. Markwell. 2014. Selection of non-toxigenic strains of Aspergillus flavus for biocontrol of aflatoxins in maize in Thailand. Biocontrol Science and Technology 24 (6):652–61. doi: 10.1080/09583157.2014.888398.
- Tran-Dinh, N., J. I. Pitt, and P. J. Markwell. 2018. Use of microsatellite markers to assess the competitive ability of nontoxigenic Aspergillus flavus strains in studies on biocontrol of aflatoxins in maize in Thailand. Biocontrol Science and Technology 28 (3):215–25. doi: 10.1080/09583157.2018.1436694.
- Turner, P. C., A. Sylla, Y. Y. Gong, M. S. Diallo, A. E. Sutcliffe, A. J. Hall, and C. P. Wild. 2005. Reduction in exposure to carcinogenic aflatoxins by postharvest intervention measures in West Africa: A community-based intervention study. Lancet (London, England) 365 (9475):1950–6. doi: 10.1016/S0140-6736(05)66661-5.
- United States Department of Agriculture (USDA). 2022. E42022-0011:Titanium dioxide banned as a food additive in the EU. Washington, DC: U.S. Mission to the European Union.
- Vandicke, J., K. De Visschere, S. Croubels, S. De Saeger, K. Audenaert, and G. Haesaert. 2019. Mycotoxins in Flanders’ fields: Occurrence and correlations with Fusarium species in whole-plant harvested maize. Microorganisms 7 (11):571. doi: 10.3390/microorganisms7110571.
- Vulic, A., J. Pleadin, and N. Persi. 2011. Determination of T-2 and HT-2 toxins in commodities and feed in Croatia. Bulletin of Environmental Contamination and Toxicology 86 (3):294–7. doi: 10.1007/s00128-011-0219-x.
- Wacoo, A. P., I. M. Mukisa, R. Meeme, S. Byakika, D. Wendiro, W. Sybesma, and R. Kort. 2019. Probiotic enrichment and reduction of aflatoxins in a traditional African maize-based fermented food. Nutrients 11 (2):265. doi: 10.3390/nu11020265.
- Wan, J., B. Chen, and J. Rao. 2020. Occurrence and preventive strategies to control mycotoxins in cereal-based food. Comprehensive Reviews in Food Science and Food Safety 19 (3):928–53. doi: 10.1111/1541-4337.12546.
- Wang, C., F. Xu, R. C. Baker, A. Pinjari, L. Bruckers, Y. Zhao, A. Stevenson, and G. Zhang. 2021. Fungi carried over in jute bags – a smoking gun for aflatoxin contamination in the food supply chain. World Mycotoxin Journal 14 (2):155–63. doi: 10.3920/wmj2020.2619.
- Wang, Y., G. Wang, Y. Dai, Y. Wang, Y. W. Lee, J. Shi, and J. Xu. 2019. Biodegradation of deoxynivalenol by a novel microbial consortium. Frontiers in Microbiology 10:2964. doi: 10.3389/fmicb.2019.02964.
- Whitaker, T. B. 2003. Standardisation of mycotoxin sampling procedures: An urgent necessity. Food Control 14 (4):233–7. doi: 10.1016/S0956-7135(03)00012-4.
- Wielogorska, E., Y. Ahmed, J. Meneely, W. G. Graham, C. T. Elliott, and B. F. Gilmore. 2019. A holistic study to understand the detoxification of mycotoxins in maize and impact on its molecular integrity using cold atmospheric plasma treatment. Food Chemistry 301:125281. doi: 10.1016/j.foodchem.2019.125281.
- Williams, S. B., D. Baributsa, and C. Woloshuk. 2014. Assessing Purdue Improved Crop Storage (PICS) bags to mitigate fungal growth and aflatoxin contamination. Journal of Stored Products Research 59:190–6. doi: 10.1016/j.jspr.2014.08.003.
- Wood, G. M. 1982. Effects of processing on mycotoxins in maize. Chemistry & Industry 18 (24):972–4.
- Wu, Y., J. Xu, E. T. Poh, L. Liang, H. Liu, J. K. W. Yang, C. W. Qiu, R. A. L. Vallee, and X. Liu. 2019. Upconversion superburst with sub-2 mus lifetime. Nature Nanotechnology 14 (12):1110–5. doi: 10.1038/s41565-019-0560-5.
- Xing, F. G., X. Liu, L. M. Wang, J. N. Selvaraj, N. Jin, Y. Wang, Y. J. Zhao, and Y. Liu. 2017. Distribution and variation of fungi and major mycotoxins in pre- and post-nature drying maize in North China Plain. Food Control 80:244–51. doi: 10.1016/j.foodcont.2017.03.055.
- Xu, C., S. Ye, X. Cui, Q. Zhang, and Y. Liang. 2018. Detoxification of aflatoxin B1 in peanut oil by iodine doped supported TiO2 Thin film under ultraviolet light irradiation. Current Nanoscience 15 (2):188–96. doi: 10.2174/1573413714666180621112032.
- Xu, F., R. C. Baker, T. B. Whitaker, H. Luo, Y. Zhao, A. Stevenson, C. J. Boesch, and G. Zhang. 2021. Review of good agricultural practices for smallholder maize farmers to minimise aflatoxin contamination. World Mycotoxin Journal 15:171–186. doi: 10.3920/wmj2021.2685.
- Yahl, K. R., S. A. Watson, R. J. Smith, and R. Barabolok. 1971. Laboratory wet-milling of corn containing high levels of aflatoxin and a survey of commercial wet-milling products. Cereal Chemistry 48 (4):385–91.
- Yang, B., L. Li, H. Geng, G. Wang, C. Zhang, S. Yang, Y. Zhao, F. Xing, and Y. Liu. 2022. Detoxification of aflatoxin B1 by H2SO3 during maize wet processing, and toxicity assessment of the transformation product of aflatoxin B1. Food Control 131 (2021):108444. doi: 10.1016/j.foodcont.2021.108444.
- Yao, H., Z. Hruska, R. D. Kincaid, T. E. Cleveland, and R. L. Brown. 2013. Method and detection system for detection of aflatoxin in corn with fluorescence spectra. Mississippi State: Mississippi State University.
- Zheng, X. Q., L. T. Li, X. L. Liu, X. J. Wang, J. Lin, and D. Li. 2006. Production of hydrolysate with antioxidative activity by enzymatic hydrolysis of extruded corn gluten. Applied Microbiology and Biotechnology 73 (4):763–70. doi: 10.1007/s00253-006-0537-9.
- Zhou, C., J. Hu, H. Ma, A. E. Yagoub, X. Yu, J. Owusu, H. Ma, and X. Qin. 2015. Antioxidant peptides from corn gluten meal: Orthogonal design evaluation. Food Chemistry 187:270–8. doi: 10.1016/j.foodchem.2015.04.092.
- Zhou, Y., S. Wu, F. Wang, Q. Li, C. He, N. Duan, and Z. Wang. 2020. Assessing the toxicity in vitro of degradation products from deoxynivalenol photocatalytic degradation by using upconversion nanoparticles@TiO2 composite. Chemosphere 238:124648. doi: 10.1016/j.chemosphere.2019.124648.
- Zielińska, K. J., and A. U. Fabiszewska. 2017. Improvement of the quality of maize grain silage by a synergistic action of selected lactobacilli strains. World Journal of Microbiology & Biotechnology 34 (1):9. doi: 10.1007/s11274-017-2400-9.