Abstract
Exposure to mycotoxins through the dietary route occurs on a daily basis while their deleterious effects are exhibited in the form of ailments, such as inflammation, cancer, and hormonal imbalance. The negative impact of mycotoxins can be attributed to their interaction with various biomolecules and their interference in metabolic pathways. The activity of biomolecules, such as enzymes/receptors, which engage the intricate mechanism of endogenous metabolism, is more susceptible to disruption by metabolites of high toxicity, which gives rise to adverse health effects. Metabolomics is a useful analytical approach that can assist in unraveling such information. It can simultaneously and comprehensively analyze a large number of endogenous and exogenous molecules present in biofluids and can, thus, reveal biologically relevant perturbations following mycotoxin exposure. Information provided by genome, transcriptome and proteome analyses, which have been utilized for the elucidation of biological mechanisms so far, are further complemented by the addition of metabolomics in the available bioanalytics toolbox. Metabolomics can offer insight into complex biological processes and their respective response to several (co-)exposures. This review focuses on the most extensively studied mycotoxins reported in literature and their respective impact on the metabolome upon exposure.
Keywords:
Introduction
Food safety is a topic of high concern, since contamination, that is, physical, biological, chemical may pose a hazard to human health. Therefore, the purity of food and absence of toxic xenobiotics is an essential requirement for food of high quality and nutritional value, contributing to good health. However, it has been noticed globally that different foods are serving as a vehicle for various xenobiotics, thus, raising food safety issues including microbial/chemical contamination, which may have adverse health effects. Such incidents are being reported with an increasing rate from economically less developed countries compared to more affluent world regions, indicating a higher degree of risk to mycotoxin-exposure related health effects in developing parts of the globe (Gizaw Citation2019). Chemical contaminants can enter food at any stage of its production, processing, transportation and storage. This contamination can occur either naturally (i.e., damage of crops by birds and insects, environmental conditions that promote the formation of spore colonies, local climate, chemicals naturally occurring in the environment) or it can be human-derived (i.e., food additives, pesticides, fungicides, and fertilizer residues, food packaging materials) (Cinar and Onbaşı Citation2019). Specifically, mycotoxins pose a significant food safety risk. Elimination of mycotoxins is a challenging task since they are highly resistant to mitigation strategies; therefore, implementation of a hazard analysis and critical control point (HACCP) system could be effective in the prevention, control and monitoring of mycotoxin contamination.
Mycotoxins are toxic secondary low-molecular weight metabolites produced by various fungi, which infect crops before or after harvest. The most extensively studied toxin-producing fungal species belong to the genera Aspergillus, Fusarium, Penicillium, Claviceps, and Alternaria (Agriopoulou, Stamatelopoulou, and Varzakas Citation2020). Mycotoxins mainly enter the food chain at the pre-harvest stage of agriculture or at the post-harvest stage during which the harvested crops are stockpiled in storehouses with conditions that favor fungal growth (Storm, Rasmussen, and Rasmussen Citation2014). As a consequence, several food products are often contaminated with mycotoxins which may represent an acute/chronic source of exposure to mycotoxins. Moreover, apart from grains and grain-based foods, mycotoxin exposure can also occur in animal-derived products as a consequence of carry-over phenomena when animals are exposed to contaminated feed.
Despite the considerable advancement in mycotoxin research, most of the studies, so far, have focused on risk assessment and determination of doses that do not cause adverse effects, while the understanding of the associated molecular pathways partially remains limited. Metabolomics approaches in combination with other “omics” disciplines and informatics tools can transcend beyond simple analysis/quantification of numerous analytes to elucidation of biological mechanisms and pathways indicative of deviations from physiological function (Johnson, Ivanisevic, and Siuzdak Citation2016). Thus, current metabolomics workflows represent promising avenues toward deciphering the mechanism of action of mycotoxins and their respective impact on living organisms (Richard-Forget, Atanasova, and Chéreau Citation2021). Understanding the molecular targets and mechanisms involved in mycotoxin-induced toxicity, with the aid of metabolomics-oriented approaches, would constitute a major paradigm shift in their toxicological research. The human biochemistry of chemical toxicity is quite complex and poorly understood. There are numerous metabolic enzymes that participate in the detoxification of parent compounds through phase I/II reactions that result in the production of metabolites that can be easily eliminated from our bodies. However, it is interesting to note that in some cases the newly formed metabolites may cause more significant adverse health effects than the parent compound. In the majority of cases, toxicity is generated by the actions of these reactive metabolites rather than by the parent compound administered.
Presently, there is no systematic review available in the literature on the topic of the contribution of metabolomics to the elucidation efforts of the mechanism of mycotoxin toxicity. However, two reviews have highlighted the need for the application of metabolomics for research into mycotoxin toxicology. The review by Dellafiora and Dall’asta (Citation2017) mainly focuses on multi-omics approaches for the toxicity evaluation of mycotoxins. Similarly, another review by Eshelli et al. (Citation2018) highlights the outcomes of studies conducted using multi-omics approaches (genomics, transcriptomics, and proteomics) but no biological outcomes generated by metabolomics are addressed, except for a short discussion on determining the LOD/LOQ of mycotoxins. Thus, no detailed systematic review regarding the role of metabolomics in deciphering the impact of mycotoxins on biological systems is currently available in the literature. This review is an attempt to summarize the studies that have, thus far, been conducted using metabolomics approaches to deepen our understanding of mycotoxin toxicity. Herein, we discuss all the available literature published from 2000 till the first quarter of 2022, which was selected by a screening process in the PubMed database using the keywords “mycotoxin” and “metabolomics.” We would like to note that only studies utilizing cell lines, animals, and humans as model systems have been included in this review.
Mycotoxins and their impact on health
Mycotoxin contamination of food and feed is one of the most challenging global constraints in ensuring food safety, security, and international trade (Agriopoulou, Stamatelopoulou, and Varzakas Citation2020). A recent review by Eskola et al. challenged the widely-cited 25% estimate of global crop contamination by mycotoxins and provided an updated value of 60–80% based on extensive literature research (Eskola et al. Citation2020). The maximum allowable mycotoxin contamination limits set by several countries lead to financial losses when these values are exceeded and, as a result, the food needs to be discarded or sold for other purposes at a reduced price. The lack of globally harmonized standards for mycotoxin contamination is a considerable constraint to international trade, with repercussions that go beyond the financial sphere, especially for low-income countries. Apart from the financial losses they cause, mycotoxins also pose a threat to human health on a global level (Janik et al. Citation2020). Consumption of mycotoxin-contaminated food or feed can induce teratogenic, carcinogenic, neurotoxic, and immunotoxic effects, among others, in humans and/or animals (Awuchi et al. Citation2021). An overview of adverse health effects caused by mycotoxins is presented in . The International Agency for Research on Cancer (IARC) has urged prompt action for the mitigation of widespread mycotoxin contamination in developing countries. According to their report, approximately 500 million people of limited means, located in the regions of sub-Saharan Africa, Asia and Latin America, are exposed to unacceptable levels of mycotoxins on a daily basis through consumption of their staple diet (IARC Citation2016).
Figure 1. Overview of potential mycotoxin-mediated adverse health effects that have been reported in animals and/or humans.
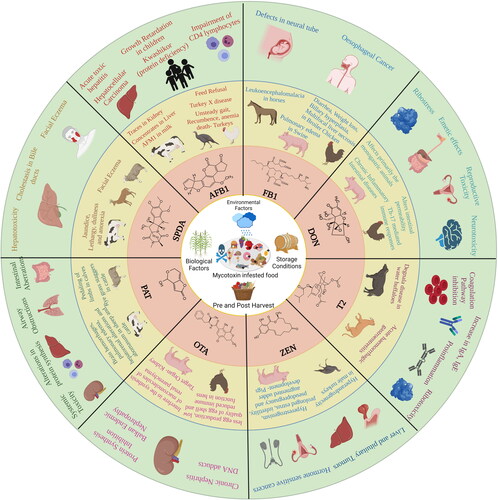
In this section, mycotoxin-mediated health effects are discussed briefly. One of the major hindrances to the research field is the quantification of the extent of exposure to individual mycotoxins. Among the known mycotoxins, aflatoxins (including naturally occurring AFB1, AFB2, AFG1, AFG2 along with the most abundant hydroxylation product AFM1) have been most widely studied for their adverse health implications. Analysis of human and animal metabolic systems has identified aflatoxin exposure biomarkers for pathologies, such as aflatoxicosis and liver cancer. Additional negative health effects include impaired growth, immune dysfunction and placental barrier penetration confirmed in animal studies (Wild and Gong Citation2010). By the effect of metabolism, intermediates that are formed tend to interact with biomolecules and form harmful adducts. AFB1-8,9-exo-epoxide interacts with DNA forming the 8,9-dihydro-8-(N7-guanyl)-9-hydroxy AFB1 (AFB1-N7-Gua) adduct which can be further split into two secondary lesions, that is, an apurinic site and a comparatively stable ring-opened AFB1-formamidopyrimidine (AFB1-FAPY) adduct, which is persistent in in vivo conditions (Benkerroum Citation2020). The latter DNA adduct has been reported to cause G to T transversions with approx. 6-fold higher frequency than its former counterpart, as well as base substitutions at the apurinic site (Benkerroum Citation2020). The G to T transversions in codon 249ser of p53 gene have been associated with aflatoxin-induced carcinogenicity (hepatocellular carcinoma) (Rivlin et al. Citation2011). The exo-/endo-epoxides undergo non-enzymatic hydrolysis to form AFB1-8,9-dihydrodiol that reacts with an ε-amino group of lysine in serum albumin to form aflatoxin-albumin adducts (Guengerich et al. Citation2002). These modifications are used as biomarkers for AFB1-induced health issues.
Exposure to fumonisins is associated with neural tube defects, liver, and esophagus cancer and growth impairment in humans (Kamle et al. Citation2019). The brain region is enriched with sphingolipids (a class of membrane lipids that participate in structural and signaling functions) which are reported to act as receptors for toxins. These biomolecules are a likely reason for the observed resistance to adverse effects, such as neural tube defects (Dasgupta and Ray Citation2017). It has been reported that due to the structural similarity of fumonisin B1 (FB1) with sphinganine, FB1 inhibits the function of ceramide synthase (CerS) via mutual competition for binding to the sphingoid base substrate, while the dicarboxylic side chains hinder the acylation of sphingosine at the amino position. Especially the N-acetylated form of FB1, exhibits a potent inhibitory effect and possesses higher cytotoxic capacity than its parent compound (Delgado et al. Citation2006). The in vivo inhibition of CerS by FB1 promotes an increase of the sphinganine-to-sphingosine ratio and disrupts the sphingolipid metabolism, thereby, bestowing toxicological and carcinogenic properties upon fumonisins (Voss and Riley Citation2013). Fumonisin-mediated CerS inhibition interferes in the signaling pathways of sphingoid base 1-phosphates, which regulate neural tube closure, thus leading to their defective function (Gelineau‐Van Waes et al. Citation2009). Synergistic effects upon sequential exposure to AFB1 and FB1 have been reported to promote liver cancer; the cancer-initiating ability of FB1 is further stimulated by the toxicity initially introduced by AFB1 (Gelderblom et al. Citation2002). The mechanism of FB1 interference with the ceramide/sphingosine signaling pathway is depicted in .
Another mycotoxin of concern is ochratoxin A (OTA) which is absorbed by the gastrointestinal tract and enters the bloodstream. OTA tends to bind with plasma proteins, especially albumin, which results in a markedly decreased uptake of OTA by organic anion transporters (regulate the balance of anions, drug/toxin flux and endogenous metabolites), thereby, causing delayed clearance rate of OTA by the kidneys (35 days half-life in human plasma) (Bow et al. Citation2006; Studer-Rohr, Schlatter, and Dietrich Citation2000). It competitively inhibits phenylalanine-tRNA ligase, while the metabolically activated form of OTA (OTA phenoxyl radical) forms DNA adducts with carcinogenic potential, and can also bind to macromolecules and proteins (Kőszegi and Poór Citation2016; Malir et al. Citation2021; Pfohl‐Leszkowicz and Manderville Citation2007; Žanić-Grubis˘ić et al. 2000). It causes nephrotoxicity and its occurrence has also been associated with Balkan endemic nephropathy. Experimental studies report carcinogenic potential by activating cyclin D1 and cyclooxygenase-2 (COX-2) via the nuclear factor-kappa B (NF-κB) and the activator protein-1 (AP-1) in mouse skin (Kumar et al. Citation2013; Kumar et al. Citation2012). IARC has maintained OTA within group 2B classification, that is, possible human carcinogen, since 1993; however, in light of recent collected and evaluated OTA data, an upgrade to group 2A, that is, probable human carcinogen, should be considered (Ostry et al. Citation2017). The detailed mechanism of action of OTA is illustrated in .
Figure 3. Mechanism of action of Ochratoxin A. OTA leads to the generation of reactive species that result in oxidative/nitrosative stress, which further may form adducts with DNA. The DNA-adduct formation may result in the generation of DNA-OTA metabolites after biotransformation (OTQ, OTHQ). Furthermore, OTA is likely to interfere with protein synthesis, energy metabolism and binds to plasma proteins for longer persistence in the blood.
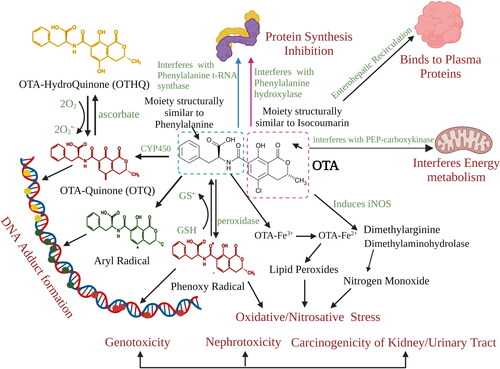
The toxicity of deoxynivalenol (DON) (another Fusarium toxin) can be attributed to the epoxide moiety present in its structure, thus, metabolic activation is not necessary for DON to induce toxic effects in biological systems (EFSA Citation2017). The underlying mechanism of DON toxicity is MAPK (mitogen-activated protein kinase) signaling activation and interaction with 60s ribosome (28s rRNA lesions; ribotoxic stress) which results in protein-synthesis inhibition, deregulation of the growth hormone, cell growth, differentiation, and apoptosis (Pestka Citation2010; Wang et al. Citation2018). DON negatively impacts health by affecting immunomodulation and the nervous system, inhibiting weight gain and causing gastroenteritis (Pestka Citation2010).
Zearalenone (ZEN) is a non-steroidal estrogenic mycotoxin. The main metabolic products of ZEN, acquired via reduction of its C-6′ keto group, are α- and β-zearalenol (α-ZEL and β-ZEL). Catalytic reduction of ZEN yields α- and β-zearalanol (α-ZAL and β-ZAL). All ZEN derivatives can be further conjugated with glucuronic acid and (partially) with a sulfate moiety during phase II metabolism. ZEN and its metabolites possess β-resorcylic acid lactone (RAL) rings which contain more than one hydroxyl group, thereby, facilitating conversion to monoglucuronide forms. The 14-O-glucuronide form is relatively abundant in vivo (tested with microsomes isolated from rat, steer, pig, human, and human intestinal microsomes). However, in the case of the metabolites of ZEN, the aliphatic 7-O-glucuronide forms are more. Specifically, the formation of the highly estrogenic α-ZEL (70-fold more potent than ZEN) is considered as a bioactivation step of ZEN (Frizzell et al. Citation2011; Pfeiffer et al. Citation2010; Ropejko and Twarużek Citation2021). ZEN interferes with steroid synthesis and metabolism by competing with substrates for enzymes involved in the pathway and, therefore, has been identified as potential endocrine disruptor. Maternofetal transfer of ZEN has been established by ex vivo placental perfusion method and it also presents great concern. It has been reported that human placenta is capable of phase I (α-ZEL) and II (ZEN-14-Sulf) transformations of the estrogenic mycotoxin. Early life exposure (especially during early pregnancy) to estrogen-like ZEN and its metabolites can result in consequential hormonal imbalance; however, this needs to be established in physiological conditions (Warth et al. Citation2019). Furthermore, it was also demonstrated that ZEN exposure results in frequent incidence of liver cell and pituitary benign tumors in mice, consistent with a hormonal mode of carcinogenic action (Awuchi et al. Citation2021; EFSA Citation2011).
T2 toxin is a type A trichothecene (no carbonyl group at C-8 position); it is the most toxic in the trichothecene family and the only member that can be directly absorbed through the skin (Adhikari et al. Citation2017). Its presence is associated with conditions such as alimentary toxic aleukia and inflammatory bowel disease (Makowska, Obremski, and Gonkowski Citation2018). The reported manifestations of T2 on health include diverse effects on the immune system, loss of body weight, vomiting, oral lesions, skin irritation and inflammation and stunted growth. It also exhibits cytotoxic and mutagenic effects (Adhikari et al. Citation2017). Modified T2 (T2-3-glucoside (T2-3-Glc), T2-3-sulfate, and T2-3-glucuronide (T2-3-GlcA)) mainly targets liver, affects reproductive health and also causes hematotoxicity, while it is rapidly excreted in the bile, urine, and feces. At low doses, T2 toxin acts as an immunostimulator causing increase in the concentrations of IgA and IgE levels in serum, inducing proinflammatory mediators, and upregulating the mRNA expression of inflammatory cytokines, such as TNF-α, IL-1β, and IL-6, augmenting the immune response with simultaneous overexpression of miR-155-5p (Kasimir et al. Citation2020). The T2-mediated toxicity is propagated by obstructing the activity of various key enzymes of the coagulation pathway, such as monoamine synthase, terminal deoxynucleotidyl transferase, DNA polymerases (Adhikari et al. Citation2017). Trichothecene toxicity can be attributed to their capability to disrupt the synthesis of proteins via binding to eukaryotic ribosomes. Binding to the ribosome can occur at different C positions of the trichothecene, with less sterically hindered binding configurations (type I substitution) leading to enhanced binding interactions, thus, increased toxicity. T2 toxins are representative of this type of interaction, which is responsible for their higher toxicity compared to their parent compounds (Wang et al. Citation2021). Another mycotoxin of concern, to which apples are particularly susceptible, is patulin (PAT), that can exert hepatotoxic, genotoxic, nephrotoxic, immunotoxic and mutagenic effects (Pal, Singh, and Ansari Citation2017). The mediation of toxicity by PAT proceeds through accumulation of ROS (reactive oxygen species), formation of adducts with biomolecules (GSH, proteins containing lysine/cysteine/histidine), inhibition of enzymes (aminoacyl-tRNA synthetases, RNA polymerases) and activity of alanine transaminase and aspartate transaminase (Janik et al. Citation2020).
Besides the most extensively studied mycotoxins, there are a few mycotoxins which raise concern but their effects have not been conclusively evaluated and/or are not regulated currently. Among such mycotoxins are sterigmatocystin (STE) and sporidesmin (SPDE). STE is a precursor of aflatoxin B1 (AFB1), sharing a common biosynthesis pathway and it typically contaminates cereals. It is mainly produced by Aspergillus and Podospora. Exposure to STE has also been associated with oxidative stress, apoptosis, cell cycle deregulation, and several cancers, such as hepatocellular carcinoma, hemangiosarcoma in liver, gastric cancer and pulmonary adenocarcinoma. It also produces double strand breaks in DNA and forms DNA-STE adducts which have been detected in specimens of cancerous and/or precancerous tissues indicating its genotoxic potential. Presence of STE has been documented in patients with hepatocellular carcinoma (urine and plasma). Upon exposure to STE, acute symptoms, such as bloody diarrhea and death in cattle, occur. On the other hand, SPDE (produced by Pithomyces chartarum) exhibits toxic effects mainly on cattle in the form of facial eczema (FE). Exposure to SPDE induces hepatobiliary injury, results in retention of chlorophyll metabolite phytoporphyrin, leads to phototoxic outcomes which are visible on face and ears when exposed to sun rays. The sulfur-bridged dioxopiperazine ring present in SPDE is chemically reactive and engages in covalent modifications of protein cysteine residues to protein-SPDE mixed disulfide forms. Furthermore, it also gives rise to ROS during oxidative recycling between the disulfide forms and dithiol forms of the toxins. The reactive nature of the mycotoxin has been identified; however, it remains to be validated in the in vivo/in vitro set up. For other non-regulated or “emerging” mycotoxins, such as the Alternaria toxins (Aichinger et al. Citation2021), no metabolomics studies to investigate biological effects/toxicity have been published to date to the best of our knowledge. Hence, they are not covered in this review.
Mycotoxins have a huge horizon of exposure which enables it to render a battery of toxic manifestations which was mentioned in section “Mycotoxins and their impact on health.” To date, several approaches have been used that have provided an understanding of mycotoxicity. Mycotoxins are known to generate multiple molecular alterations, such as oxidative free radicals, DNA damage, protein modifications, chromosomal aberrations, alterations in small molecules. ROS generation can be measured by different fluorescence-based methods, such as the DCF-DA (2′,7′-dichlorofluorescein-diacetate) assay (Juan-García et al. Citation2020). DNA damage can be evaluated by methods such as the comet assay, Hoechst staining, and analysis of molecular markers by PCR (polymerase chain reaction) and/or western blotting. Methods used for the detection of chromosome aberrations are the micronucleus test, single cell electrophoresis, FISH (fluorescence in situ hybridization) (Domijan et al. Citation2015). Similarly, differential alterations in gene expression can be attained by real time PCR and microarray (Romero et al. Citation2016). However, many molecular techniques have been developed that have helped researchers to gather accurate information. Omics-based technologies, such as genomics, transcriptomics, proteomics and metabolomics have emerged as a novel and robust approach to collect large amounts of information rapidly, which can be used for getting a deeper insight in the mechanism of toxicity of mycotoxins. Among these, metabolomics, which is the comprehensive analysis of metabolites, is an emerging approach in the field of toxicology. Metabolomics may provide detailed information about the changes caused by chemicals and about the biochemical/physiological status of a biological system, which will help in identification of exposure biomarkers for mycotoxicity or prognostic markers for mycotoxin-induced health effects.
Metabolomics: an emerging tool to assess mycotoxicity
Endogenous metabolites present in biological specimens belong to chemically diverse compound classes, for example, amino acids, steroids, lipids, characterized by different physico-chemical properties with distinct physiological role in health and disease. The interactions of xenobiotics with the biological system tend to perturb concentrations of endogenous metabolites involved in pivotal biochemical pathways. Metabolomics is an integrative branch of science that amalgamates the application of analytical chemistry, biochemistry, computational science, and statistical analysis to identify as well as to characterize the end products of biometabolism. It specifies the activity of small molecules (below 1.5 kDa) generated by living cells exposed to various conditions, which is not within the scope of genomics, proteomics, and transcriptomics studies (Muthubharathi, Gowripriya, and Balamurugan Citation2021). Furthermore, it can provide broad yet accurate information about the changes that occur as a response to internal and/or external environmental changes. This approach enables us to decipher pathways of toxicity (PoT) and identify fingerprints that are indicative of toxicity (extent of severity). PoT gives an insight into the cellular processes that are the connecting links between the chemico-biological interactions of mycotoxins with biological systems and their respective adverse outcomes. To understand the effects of mycotoxins on biological systems, in vivo and in vitro studies have been widely used. In vitro findings are further validated using animal models which serve as a case scenario to understand the different physiological effects on humans. In the case of mycotoxicology, rodent models such as kumming mice, CD-1 mice, wistar rats, F344/N rats, have been widely used to evaluate overall and specific effects of mycotoxins. In addition to rodent models, livestock, such as dairy cows, have been used in risk assessment studies of mycotoxins.
Such information can help bridge the knowledge gap in the mechanism of action of mycotoxins that contributes to different pathophysiologies and take a step further toward mitigation strategies. outlines the two approaches for performing metabolomics studies, that is, targeted (pre-defined set of metabolites are determined) and untargeted (detection of known and unknown metabolites) (Malachová et al. Citation2018). Furthermore, metabolomics approaches are continuously advancing. Recently, the use of stable isotope-assisted metabolomics was reported in the successful identification of newly identified biotransformation products in human cell models, including ZEN-pyridoxine, DON-3-sulfate, DON-10-sulfonate 2, and DON-10-glutathione as well as DON-cysteine, which introduced additional knowledge to the existing information concerning the biometabolism of ZEN and DON (Flasch et al. Citation2022; Flasch et al. Citation2020). In recent work, Bueschl et al. developed a machine learning approach in order to optimize peak picking in untargeted metabolomics. The developed PeakBot is highly effective in distinguishing between chromatographic peaks and background noise in LC-HRMS databases (Bueschl et al. Citation2022) and can aid metabolomic studies on mycotoxins.
Metabolomics offers unique insights into an organism’s phenotype since metabolites are the output of gene expression and protein synthesis. Additionally, small biomolecules in real-time and through the entire cell life cycle can be accessed. Extraction of such information is not within the reach of other “omics” disciplines, thereby, rendering metabolomics a powerful and, at the same time, complementary tool to the existing “omics” technologies. Quite importantly, the metabolic similarities across species imply that metabolomics can serve as a global interpretation system of biological activity (Peng, Li, and Peng Citation2015). The analysis of the metabolic signature first emerged in the 1960s and the first publication using the specific term “metabolome” appeared in literature in 1998 (Oliver et al. Citation1998). Many analytical platforms have been developed in order to account for the different types of samples, concentrations and target analytes; nuclear magnetic resonance (NMR) and separation techniques such as liquid chromatography (LC), gas chromatography (GC), capillary electrophoresis (CE), supercritical fluid chromatography (SFE) coupled with mass spectroscopy (MS), are some of the most commonly used strategies in metabolomics-based research (Segers et al. Citation2019).
Metabolomics in mycotoxin toxicity studies
The “omics” tools are now readily used to elucidate biological pathways that provide a better understanding of observed phenomena. The metabolic profile gives a snapshot of the ongoing biological processes within the cell, and, simultaneously, any deviation from the homeostatic state of the biological system. Here, we address the use of metabolomics in understanding mycotoxin-associated pathologies.
Metabolomics in single-mycotoxin toxicity studies
Aflatoxin B1 (AFB1)
As the liver is the main site for detoxification of xenobiotics, it automatically becomes a target site for xenobiotics. AFB1 is also known to cause liver fibrosis and carcinoma in humans which accounts for approximately 70% of cancer-related deaths in humans where aflatoxicosis is endemic and it has been evaluated that around 28% of hepatocellular carcinoma worldwide may be attributed to AFB1 (Yan Liu and Wu Citation2010; Magnussen and Parsi Citation2013; Ostry et al. Citation2017). AFB1 interferes with the main site that handles detoxification of harmful substances and alters metabolic pathways, such as glycogenolysis/glycolysis, therefore, it is likely that AFB1 may be affecting other metabolic signaling too. In order to verify this, a metabolomic study involving different biological matrices (due to metabolite compartmentalization) was warranted. In 2011, Zhang et al. analyzed multiple biological matrices of AFB1-exposed rats to understand the metabolic changes originating after mycotoxin exposure. Urine, plasma, and liver tissue extracts, from control and exposed rats, were analyzed using 1H-NMR spectroscopy. The investigation confirmed AFB1 leads to hepatic steatosis and extensive metabolic changes in glycolysis, lipid and cell membrane metabolism, TCA cycle, protein biosynthesis and gut microbiota functions in the liver, which indicates disruption of mitochondrial function brought on by oxidative stress () (Zhang et al. Citation2011). Furthermore, Lu et al. (Citation2013) looked deeper into the source of AFB1-induced hepatotoxicity in the search of novel biomarkers relevant to the pathophysiological state by integrating transcriptomics and metabolomics. The research group reported the involvement of the p53 signaling pathway in oxidative damage induced by AFB1. The study also confirmed the previous finding of Zhang et al. where disruption in gluconeogenesis and lipid metabolism were established as the major metabolic effects due to acute AFB1 exposure, and suggested to be used as a biomarker for detection of AFB1-induced hepatotoxicity () (Lu et al. Citation2013). Furthermore, the reported metabolic changes triggered similar studies with the aim to unveil the metabolic status after exposure to AFB1. A study on dairy goats exposed to low-dose AFB1 (50 µg/kg DM) revealed major changes in the metabolic profile, especially the lipid metabolism. Seventeen metabolites (lactate, glucose, citrate, acetate, acetone, citrulline, leucine/isoleucine, glycine, valine, creatine, betaine, acetoacetate, choline, β-hydroxybutyrate, (phosphatidylcholine)PC/glycerophosphocholine(GPC), lipoprotein, and N-acetyl glycoproteins) participating in carbohydrate, lipid, and amino acid metabolism were found to be altered in the AFB1-exposed dairy goats () (Cheng et al. Citation2017). Multiple biomatrices (rumen fluid, blood, and milk) from dairy cows were evaluated in order to get a comprehensive understanding of the metabolic changes resulting from AFB1 exposure by combining 1H-NMR spectroscopy and biochemical assays. Altogether, 39 metabolites were found to be affected of which 19 were rumen fluid metabolites, 11 were plasma metabolites, and 9 were milk metabolites. Among the affected metabolites, the most significant impact by AFB1 exposure was observed on phenylalanine in all three biofluids. The authors concluded that mycotoxicity should be assessed not only through micro-indicators (biofluid biomarkers) but also macro-indicators (milk composition and production) for complete assessment () (Wang et al. Citation2019). Taking the available information into consideration, research was performed utilizing intact zebrafish embryos as models for determining the overall metabolic changes arising from AFB1exposure. For the study, high-resolution magic angle spin (HRMAS) NMR was deployed and a range of AFB1 concentrations up to 2 µM (0, 0.5, 1, 1.5, and 2) for different intervals (24, 48, 72, and 96 h) were evaluated. The metabolomic profiling verified the hepatotoxicity of AFB1, which is consistent with deviations in the amino acid/lipid/carbohydrate metabolic pathways noted in previous research conducted in mammalian models. Additionally, this study presents data that suggest the neurotoxic potential that AFB1 possesses. A prominent alteration in the metabolites associated with the function as well as the development of the central nervous system, that is, GABA, Glu, and Gly, was observed () (Zuberi et al. Citation2019). The information on the AFB1-mediated metabolite profile can be used not only for the recognition of the mycotoxin toxicity but also for its mitigation. concisely depicts the AFB1 altered metabolites in different biomatrices.
Figure 5. Major metabolic alterations induced by AFB1-mediated hepatotoxicity. AFB1 leads to hepatic steatosis by altering amino acid, carbohydrate and lipid metabolism. Presence of metabolites from altered pathways can be detected in liver extracts, urine and blood.
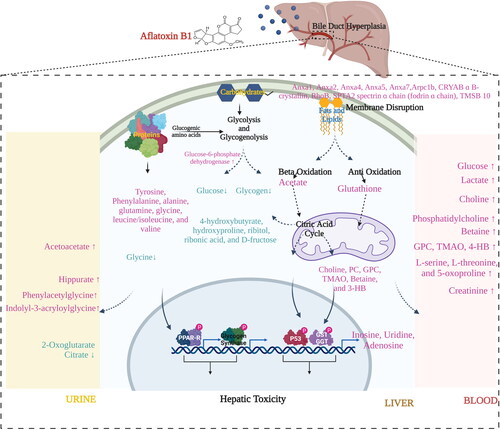
Table 1. List of 1H-NMR metabolomics to study the mycotoxin metabolism, toxicity mechanism, role in pathogenesis and disease.
Table 2. List of GC-MS-based metabolomics to study the mycotoxin metabolism, toxicity mechanism, role in pathogenesis and disease.
It is, thus, now more apparent that AFB1 mediated hepatotoxicity is reflected in the form of altered levels of different metabolites associated with some crucial metabolic pathways, such as lipid/amino acid/carbohydrate/glucose metabolism. Besides targeting the liver, the metabolic profile also indicated the effect of AFB1 on the central nervous system. This provides room for investigation of metabolic changes using human samples (different matrices), for validation and correlation of these metabolic changes as early biomarkers of AFB1 toxicity.
Fumonisin B1 (FB1)
The FB1 toxicity is canonically attributed to dysregulated lipidomic profiles and lipid metabolism leading to physiological consequences such as disturbed membrane integrity and lipid raft formation. In addition, it is also reported that FB1 can inhibit CerS, with a sequential buildup of sphingosine, sphinganine, and their 1-phosphate derivatives ( and ) (Voss and Riley Citation2013). These lipid metabolites are ubiquitous; however, the brain is especially rich with sphingolipids. Moreover, alterations in these metabolites can be correlated with neurotoxicity and neural tube defects in babies. Besides the central nervous system (CNS), sphingolipids are found in vital sites performing pivotal physiological roles such as growth regulation, cellular migration/adhesion, apoptosis, senescence and channel inflammatory responses. Therefore, further studies must be undertaken to establish that alterations in lipid metabolism (esp. sphingosine metabolism) are related to FB1-mediated cancer initiation, pulmonary edema and leukoencephalomalacia.
Figure 6. Major mycotoxin-mediated alterations in metabolic pathways. The exposure to mycotoxins results in several adverse health effects as mentioned in “Mycotoxins and their impact on health” and . The alterations in amino acid metabolism, nucleotide, glucose, lipid/fat, and energy metabolism are majorly found to be involved. The presence of metabolites found in the different biofluids and fractions can be correlated to the mycotoxicosis and can be helpful in formulating a therapeutic regimen.
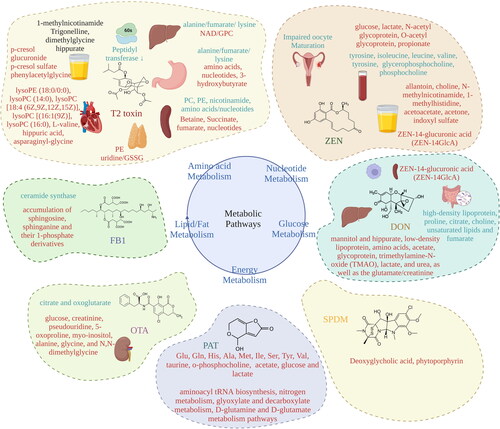
T2 toxin
Investigations in rats have correlated the toxicological endpoints with the altered metabolites. One study found that T2 toxin (0.5, 2.0, and 4.0 mg/kg body weight, orally administered) exposed rats have differentially altered metabolites in urine, liver, spleen, stomach and thymus. High levels of p-cresol glucuronide, p-cresol sulfate and phenylacetylglycine and low amount of 1-methylnicotinamide (MNA), trigonelline, dimethylglycine (DMG), and hippurate were reported during urinary metabolomics of T2 toxin exposed rats. Metabolome analysis of several organs identified enhancement of betaine, succinate, fumarate, and nucleotides with a concurrent decrease in phosphatidylcholine (PC), phosphatidylethanolamine (PE), nicotinamide, amino acids and nucleotides in the spleen, while higher uridine and glutathione disulfide (GSSG) and lower PE levels were detected in the thymus. T2 exposure resulted in augmentation of NAD and glycerophosphocholine (GPC) levels and decline in alanine, fumarate and lysine. In the stomach, a surge in amino acid levels, nucleotides and 3-hydroxybutyrate was noted. The research provided insight into the impact of acute exposure to T2 toxin, specifically, accumulation of oxidative load, alterations in cell functions (membrane integrity, protein synthesis, nucleotide synthesis, energy metabolism) and spleen function (immunomodulation), and imbalance in the gut-microbiota function () (Wan et al. Citation2015). In 2016, Wan et al. performed an 1H-NMR metabolomic study to determine the exposure of broiler chickens and piglets to T2 toxin. The toxin was administered intravenously which resulted in altered energy metabolism. Overall, the metabolic response was exhibited in the form of reduced glucose levels, accumulated lactate, decreased TCA intermediates, elevated creatine levels, and augmented GSSG levels, in the serum of broiler chickens, indicative of the effect of T2 toxin on energy metabolism and generation of oxidative stress. The analysis also revealed a significant effect on amino acid and nucleotide metabolisms on both broiler chickens and piglets () (Wan et al. Citation2016). These research outcomes indicate that T2 toxins shatters the cellular homeostasis by targeting mitochondrial (energy metabolism), ribosomal (amino acid metabolism) and nuclear health (nucleotide metabolism). Another study, conducted by Lei et al. (Citation2018), confirmed the cardiovascular alterations that T2 toxin induces on rats. The analysis of the metabolomic output supported the information on articular cartilage destruction. The study reported a significant change in 8 metabolites, that is, lysoPE, lysoPC (16:0, 16:1, 18:4, and 14:0), L-valine, hippuric acid, and asparaginyl-glycine in the T2 toxin treated rats when compared to the control group. The presence of these metabolites in serum is indicative of cartilage damage () (Lei et al. Citation2018).
Table 3. List of LC-MS/MS and UPLC-Q-TOF-MS-based metabolomics to study the mycotoxin metabolism, toxicity mechanism, role in pathogenesis and disease.
Ochratoxin A (OTA)
To enable early detection of OTA and a better understanding of its associated toxicity, several metabolomic studies have been performed. In order to correlate the phenotypic features of OTA toxicity with metabolic changes, a metabolomic analysis utilizing GC-MS, LC-MS, and 1H-NMR was carried out in urine samples of OTA-fed F344/N male rats (orally). Significant changes were reported for 10 of the metabolites (decrease in citrate and oxoglutarate; increase in glucose, creatinine, pseudouridine, 5-oxoproline, myo-inositol, alanine, glycine, and N,N-dimethylglycine) involved in the Krebs cycle, which can be correlated to the OTA-induced renal malfunction (). The author suggests the use of the tool combination, that is, GC-MS, LC-MS, and 1H-NMR, for urinary metabolome analysis since it correlates to the histopathological finding that is noted in the case of OTA toxicity (Sieber Citation2009). It has been reported that due to its stability OTA can accumulate in the system and, consequently, exhibit toxic manifestations. Mantle et al. attempted to confirm the magnitude of OTA levels by using one single dose of 40 mg in a rat model. It was noted that an OTA dose representative of cumulative exposure to OTA will be capable of inducing deleterious effects, as OTA is stable in serum and its presence can be used as a predictor of cancer. Therefore, Mantle, Nicholls, and Shockcor (Citation2011), tried to recreate high OTA exposure by means of frequent consumption of food commodities, such as cereals/beverages, with single exposure experiments. The research group conducted a uremic toxicity study where the acute toxic effects were noted. They reported that the initial signs of toxicity brought about by exposure of rats to OTA were reversible and the original state was gradually reached over a period of time (approx. 6 months), provided that no histopathological damage had occurred during the exposure (Mantle, Nicholls, and Shockcor Citation2011). Furthermore, with the help of GC-MS and 1H-NMR, the metabolome of OTA exposed rats was studied by Xia et al. to gain a better understanding of the systemic responses and identify a probable biomarker for OTA exposure. Biofluids, that is, plasma and urine, were used for the metabolomic analysis in accordance with the histopathological findings. The pathological study pointed out kidney damage but no liver damage. In line with these findings, various metabolic pathways were found to be altered () (Ellis et al. Citation2011; Xia et al. Citation2014). The half-life of OTA is comparatively long in serum, it interacts with serum proteins, forms adduct with nucleic acids, increases oxidative load and is also capable of causing cancer. The studies about OTA’s influence on overall metabolism and its correlation with disease state are still scarce. Therefore, from a risk assessment point of view and accurate determination of early exposure markers for OTA, further studies are a matter of high importance.
Deoxynivalenol (DON)
Several researchers have ascertained the presence of DON in urine samples collected from DON-exposed subjects (Brera et al. Citation2015; Hopton et al. Citation2010; Vidal et al. Citation2016). Therefore, using urine as biomatrix, Hopton et al. evaluated DON-exposed humans in order to identify potential correlations between the level of exposure and the presence of certain metabolites in urine. The analysis was performed with the aid of 1H-NMR spectroscopy. Based on the statistical analysis, two metabolites were pinpointed as potential exposure biomarkers, i.e., mannitol and hippurate. While the presence of mannitol is indicative of processed-food consumption, from a mechanistic perspective, it is most likely irrelevant to exposure; therefore, it was not evaluated further. Hippurate, on the other hand, is formed as a result of renal and hepatic activity via conjugation of glycine and benzoic acid and it can also be produced by the action of gut microbiota, and the authors concluded that it might serve as a biomarker of exposure (Hopton et al. Citation2010). However, the limited sample size should be considered and validation of the results in other cohorts and by alternative techniques would be needed. Wu et al. investigated whether dietary supplementation could minimize the toxic effects of DON. Following DON exposure of piglets, the metabolome was studied using 1H-NMR spectroscopy; elevation of the levels of low-density lipoprotein, glycoprotein, amino acids (Ala, Arg, and Gly), trimethylamine-N-oxide (TMAO), acetate, urea, lactate, as well as the glutamate/creatinine ratio was noted. On the contrary, a decrease in high-density lipoprotein, choline, proline, unsaturated lipids, citrate, and fumarate was observed. The authors stated that supplementation of diet with glutamate decreased the alterations in the energy and amino metabolism caused by DON exposure. The authors suggest that the use of glutamate may be beneficial in dealing with DON-mediated toxicity () (Wu et al. Citation2014).
Considering the ability of DON to affect rapidly proliferating cells, such as immune cells, a metabolomics study on murine macrophage ANA-1 cells was conducted for inspection of possible alterations. The analysis demonstrated a major effect on two metabolic pathways, that is, amino acid metabolism and glucose metabolism. The metabolomic profiles obtained, using GC-TOF-MS, reflected deviations in 27 endo- and exo-metabolites of the ANA cells involved in the two mentioned pathways, which indicated DON-mediated immunotoxicity and unnecessary activation of these immune cells that may direct them toward apoptosis () (Ji, Sun, et al. Citation2016). The research group performed another study on Kunming mice, using GC-MS, to probe the metabolic profile of their kidney and spleen following DON exposure. The energy metabolism and protein biosynthesis in these two organs were disturbed. Alteration of 58 metabolites in the kidney (24 upregulated and 34 downregulated) and 20 metabolites in the spleen (3 upregulated and 17 downregulated) was observed after DON exposure. The metabolic profile confirmed the immunotoxic capacity of DON since it could disrupt the immune functions by affecting the metabolism () (Ji, Zhu, Cui, Pi, Zhang, and Sun Citation2017). DON interacts with the gut microbiota for modulating its detoxification and the homeostasis of the vicinity. Toxic effects of DON are generated by its interaction with gut microbiota; however, it is unknown if gut microbiota participate in the detoxification process, especially when low-level exposures are considered. To elucidate this matter, the metabolome of fecal matter of Wistar rats, which had consumed DON, was monitored for DON and DOM-1 using UPLC-MS/MS. An increase in DOM-1 was observed with a concomitant increase of the genus Coprococcus (cocci of gut microbiota). Although this genus is suspected to play a role in DON detoxification, further data are needed to conclusively establish this correlation () (Miró-Abella et al. Citation2018). Recent research reported on the effect of ozone on DON degradation products. A GC-TOF-MS-based approach was used to evaluate the cytotoxicity of the degradation products on a Caco-2 cell line. The cells were dosed with aqueous ozone-treated DON for different time intervals, followed by cytotoxicity assessment and metabolic analysis. The metabolome evaluation showed minimal alterations in the metabolic pathways that are normally affected by DON exposure and, based on the experimental outcome, the authors suggest use of ozone treatment on food products as a safety measure against mycotoxin hazards () (Xu et al. Citation2019).
Studies focusing on DON’s effect on the metabolic status have been mostly pointing out disturbed energy and amino acid metabolism, which was prevented by glutamate and probiotics supplementation. However, more studies are warranted for understanding efficient alternatives for mitigation of DON-induced toxic effects. Additionally, correlation between affected nervous system and gastroenteritis (centering interaction with gut-microbiota and intestinal health) due to DON exposure needs to be established along with identifying metabolic biomarkers of the condition. DON is a mycotoxin of concern and strategies must be developed to combat its toxic effects. More metabolomics studies in this direction will enable better understanding and potential interventions in the future.
Zearalenone (ZEN)
Very little is known about how ZEN exposure modulates the metabolic balance in humans or animals. The first report discussing ZEN-induced metabolic alterations was published by Liu et al. (Citation2013). The study involved intragastric administration of ZEN to rats for 2 weeks and subsequent metabolic profiling of collected biofluids (blood and urine) with the aid of 1H-NMR. The metabolites detected in urine and plasma indicate that ZEN generates oxidative stress and alters some systemic metabolic pathways associated with the cell membrane, protein biogenesis, glucose metabolism, and gut microbiota. In urine, augmented levels of allantoin, choline, N-methylnicotinamide, 1-methylhistidine were detected 0–8 h after ZEN was administered and increased levels of acetoacetate, acetone, and indoxyl sulfate 8–24 h after ZEN administration. The increased levels of the aforementioned molecules indicate the affected purine synthesis induced by ZEN. In plasma, an increase in lactate, glucose, O-acetyl glycoprotein, N-acetyl glycoprotein, propionate was observed with concurrent decrease in tyrosine, branched-chain amino acids (isoleucine, leucine, valine, and tyrosine), and choline (glycerophosphocholine and phosphocholine) metabolites () (Liu et al. Citation2013). The metabolic profile suggested altered purine, amino acid and lipid metabolism with a significant oxidative stress response due to ZEN-induced toxicosis. As mentioned earlier, ZEN induced reproductive toxicity due to its estrogen-mimicking ability. A study was conducted where porcine follicular cell culture was exposed to ZEN, and granulosa cell culture media was assessed for metabolic changes using UPLC-Q-TOF-MS. Elevated levels of phosphatidylcholine or phosphatidylethanolamine adducts in the culture media were detected, while exposure to ZEN depleted the metabolite lysophosphatidylcholine (important for the follicular growth), ultimately obstructing proper follicular development and oocyte maturation () (Lai et al. Citation2018). Another metabolome analysis of breast cancer cell lines, using a 1H-NMR-based approach, revealed that α-ZEL is capable of increasing protein biosynthesis and lipid metabolism in the positive breast cancer cell line MCF7. The elevated levels of proline, hydroxyproline, arginine and ATP levels in breast cancer cells were predicted with the ability of α-ZEL to induce breast cancer progression upon exposure, which needs to be validated further in in vivo models () (Nittoli et al. Citation2018). However, there is a lack of targeted metabolic studies dealing with the effect on metabolic pathways involved in regulation of reproductive health in females (esp. using biomatrices from the reproductive system). However, an untargeted metabolomics study revealed that ZEN (besides the isoflavone genistein) is able to reverse the metabolic effect of a breast cancer combination therapy in the MCF7 cell model (Warth et al. Citation2018), a fact that should be further investigated in vivo within the framework of ‘drug-exposome interactions’ (Pristner and Warth Citation2020).
Often, modified versions of the mycotoxin, that is, ZEN-14-glucoside (ZEN-14G) or deoxynivalenol-3-β-D-glucoside (DON-3G) are found to contaminate the food/feed, falsely leading to underestimation of potential toxicity if only the parent compound is considered. A recent study attempted to identify the metabolic profile and toxicokinetic behavior of ZEN-14G, which can hydrolyze to its toxic parent ZEN, in rats, following its oral/intravenous administration. The toxicokinetics were investigated with the aid of LC-MS/MS, whereas the metabolomic profiling was performed with LC-HRMS. Low bioavailability levels of the modified mycotoxin were noted, while the metabolomic analysis revealed the presence of eight metabolites (α-ZEL, α-ZEL-14G, α-ZEL-14GlcA, ZEL-14GlcA, ZEN, β-ZEL, β-ZEL-14G, and ZEN-14G-16GlcA); specifically, ZEN-14-glucuronic acid (ZEN-14GlcA) was the most prominent of all in the plasma of ZEN-14G-exposed animals. Therefore, ZEN-14GlcA can serve as a potential biomarker of ZEN-14G () (Sun et al. Citation2019). A recent study investigated the effect of ZEN exposure in weaned piglets by biochemical analysis of collected serum. Serum collected on days 7 and 21 was analyzed, in which significant alterations on the adipokine levels were observed. Adiponectin, resistin, and fetuin B, which are pivotal in adipose metabolism and biosynthesis, were found to be significantly altered (Nagl et al. Citation2021).
Patulin (PAT)
PAT is produced mainly by Penicillium expansum and by a few Aspergillus as well as Byssochlamys species. The presence of PAT is mainly reported in apples and apple juices. As mentioned earlier, in the section “Mycotoxins and their impact/effect on health.” PAT shows hepatotoxicity, immunotoxicity, neurotoxicity, nephrotoxicity, genotoxicity, teratogenicity, and carcinogenicity (dermal route). PAT disrupts cellular permeability, interferes with the transport of ions, and leads to compromised integrity of intestinal tight junctions. Our lab has been an active contributor to understanding the dynamics of mycotoxin-mediated health effects. Recently, our team (Singh et al.) conducted a study to get an insight into the intricacies of PAT-induced carcinogenicity. Singh et al. conducted an elaborate study using 1H-HRMAS-NMR (non-targeted approach) revealing the presence of a total of 28 metabolites (12 significantly upregulated) such as Glu, Gln, His, Ala, Met, Ile, Ser, Tyr, Val, taurine, O-phosphocholine, acetate, glucose, and lactate. The study mentions that, as a result of exposure to PAT, interference with various processes, that is, aminoacyl tRNA biosynthesis, nitrogen metabolism, glyoxylate and decarboxylate metabolism, D-glutamine, and D-glutamate metabolism, was observed. The study was the first of its kind reporting the metabolic changes that occurred due to low-dose chronic exposure of intestinal cells to PAT, which may be contributors in PAT-mediated carcinogenicity () (Singh et al. Citation2021). The study pointed out deviations occurring in energy metabolism (TCA intermediates) may be involved in altered macromolecule synthesis and oxidative homeostasis. In addition, the altered amino acid metabolism can be correlated with altered macromolecule biosynthesis, contribution in cancer development and metastasis (Glu) to malignancy (taurine), while branched amino acids (Val) may serve as fuel for energy requirements in carcinogenesis. Furthermore, Pal et al. (Citation2022) contributed a metabolomics-aided insight into PAT-induced nephrotoxicity. Significant metabolic alterations were observed in both biomatrices, that is, blood and serum that were tested in this study; specifically, 167 altered metabolites were reported in serum and 219 in urine samples of the PAT-treated experimental animals. Based on data analysis, it was further concluded that 26 metabolites from serum and 11 from urine can be considered as potential biomarkers for PAT-induced nephrotoxicity. PAT exposure affected amino acid (Phe, Tyr, and Trp) biosynthesis, amino acid (Gly, Ser, Thre, Ala, Asp, and Tyr) metabolism and α-linolenic metabolism. The study highlights the fact that PAT acts as a metabolism disrupting mycotoxin which ultimately leads to nephrotoxicity () (Pal et al. Citation2022).
Sporidesmin (SPDE)
SPDE is produced by the saprophytic fungus Pithomyces chartarum. It has hepatotoxic potential and can cause an obstruction of the bile duct. A hepatogenous photosensitization disease in ruminants has also been reported, due to the particular mycotoxin, known as facial eczema (FE). Following exposure to SPDE, high levels of phytoporphyrin are reported in the blood (Scheie et al. Citation2003). Metabolomic analysis of blood serum using UPLC-MS revealed an increase in bile acids and deoxycholic acid following intra-ruminal SPDE administration to cows. On the basis of these results, an association between the levels of deoxycholic acid and FE is reported, while the authors suggest further research to establish bile acids as potential biomarkers of SPDE exposure () (Matthews et al. Citation2018). The effects of SPDE on metabolism remain not sufficiently explored.
Metabolomics in mycotoxin-mixture toxicity studies
A considerable issue with regard to mycotoxin contamination in food/feed is poly-contamination, that is, the food/feed is simultaneously contaminated with more than one mycotoxin (Agriopoulou, Stamatelopoulou, and Varzakas Citation2020). This occurrence can be attributed to the fact that not only can one fungus produce multiple mycotoxins but also co-occurrence of several fungal species is a distinct possibility, depending upon the environmental factors of the cultivation areas and the storage facilities (Daou et al. Citation2021). Therefore, the impact of poly-contamination can be much more severe than the impact of individual mycotoxins due to possible interaction effects (i.e., additive, synergistic, or antagonistic) (Fremy et al. Citation2019; Battilani et al. Citation2020). Studies dealing with mycotoxin mixtures and their respective impact on metabolic status are scarce in literature. Despite ongoing research contributing to an increased understanding of mycotoxin-mediated health hazards, information about the actual risk of combined mycotoxins is still poorly understood. The following section of the review deals with metabolic changes resulting from the simultaneous presence of multiple mycotoxins.
Studies of binary mixtures
DON + ZEN
ZEN, DON and their metabolites, α-ZEL, β-ZEL, zearalanone (ZAN), α-ZAL, β-ZAL, and DOM-1 as well as their respective glucuronides, have been detected not only in human but also in animal specimens (Dänicke and Brezina Citation2013; Olsen et al. Citation1985; Zöllner and Mayer-Helm Citation2006). A sensitive and selective LC-MS/MS method using negative electrospray ionization (ESI) was developed for the simultaneous determination of ZEN, DON, and their metabolites present in plasma, liquor and urine of female piglets (Brezina et al. Citation2014). Limited information is available in the literature concerning the combinatory effect of mycotoxins. As mentioned earlier, metabolomic studies are ideal for the collection of large datasets in a time-efficient manner. There are only few reports discussing the metabolic status in the presence of DON + ZEN. Using GC-TOF-MS, the metabolomics response of ANA cells exposed to DON + ZEN was evaluated. The pentose phosphate pathway (PPP) was found to be affected in addition to the amino acid- and glyco-metabolism. In this context, 4 specific metabolites were identified, that is, palmitic acid, 1-monopalmitin, ribose-5-phosphate, and 2-deoxy-D-galactose. This combination mitigated the estrogenic property of ZEN to some extent but PPP was specifically affected by their combination (Ji, Zhu, et al. Citation2016). The metabolic alterations on Kunming mice receiving DON + ZEN were investigated with the aid of serum and liver tissue samples. Analysis of liver samples showed 3 up-regulated and 17 down-regulated metabolites, while serum fractions had 6 down-regulated and 14 up-regulated metabolites. Although the biomarkers in the case of individual ZEN and DON treatments are compatible with liver damage, the combined ZEN + DON treatment indicates liver recovery. Additionally, the combined DON + ZEN treatment down-regulates the valine, leucine and isoleucine biosynthesis, and glycine, serine and threonine metabolism, and O-glycosyl compound-related glucose metabolism in liver tissue () (Ji, Zhu, Cui, Pi, Zhang, Li, et al. Citation2017). This report indicated reduced toxic manifestations of combined exposure to ZEN + DON on liver too, however, the reason remained unclear warranting further studies. Another metabolic profiling study on mice urine using GC-MS showed adverse effect majorly on galactose metabolism and alterations in TCA cycle. Decline in metabolites related to galactose, including mannitol, galactonic acid, myo-inositol, and tagatose indicated alterations in glucose metabolism. In addition to this, reduced levels of pyruvate, isoleucine, isocitric acid, serine, citric acid, and alanine, as well as their acetylated amino acids was also important to note () (Ji et al. Citation2018). It is interesting to note that the combination of these two mycotoxins does not increase the toxic load on liver, however, it affects energy, glucose and amino acid metabolism. Additionally, the studies conducted used a very low dose of ZEN which is lower than the actual exposure as well as unable to induce any toxic manifestations. Therefore, studies using real-life representative doses of ZEN in combination with DON need to be conducted for proper evaluation of the effects upon their co-occurrence. Furthermore, extensive metabolomics studies must be performed to establish possible correlation of toxic manifestations in the case where co-occurrence of these two mycotoxins is observed.
AFB1 + ZEN
A report states that low concentrations of ZEN (10 and 20 µM) amended the early apoptosis induced by 1 µM AFB1 on the cell line PK-15 (porcine kidney 15); on the other hand, ZEN at the dose of 40 µM combined with 1 µM AFB1 had an additive impact on late apoptosis. The study mentions the criticality of dose and response in this binary mixture. At a low dose of AFB1 antagonistic effect with ZEN was noted, whereas, at a high dose, AFB1 acts synergistically with ZEN. This study reports the cellular modulations that occurred as a consequence of exposure to the binary mixture (synergistic effect), however, the effect on metabolic status still remains to be understood. Furthermore, as the individual toxins alter various metabolic pathways, metabolomic studies for AFB1 + ZEN mixtures are warranted (Lei, Zhang, and Qi Citation2013).
AFB1 + DON
The study mentioned in the previous section also investigated the combined effect of AFB1 and DON on the survival capacity of BRL-3A rat liver cells. The binary mixture displayed a synergistic effect, as the combined dose increased early apoptosis in the studied cell line (Sun et al. Citation2015). Studies focusing on the evaluation of the metabolic status by use of different biofluids along with cellular extracts must be undertaken. The information obtained from such studies will be useful in identifying early exposure markers, which will help in improving health outcomes.
AFB1 + FB1
Rat liver cells BRL-3A were used in order to assess the possible cytotoxic effects of the AFB1 + FB1 mixture as well as its impact on the cell cycle function. The observed response to exposure is an increase in the production of ROS via stimulation of the metabolic pathways that are major ROS contributors, that is, arachidonic acid metabolism and cytochrome P450 activity. Further, the higher hepatocarcinogenic ability attributed to the mixture as opposed to the individual mycotoxins indicates the presence of a synergistic relationship/effect (Mary et al. Citation2017). The AFB1 + FB1 mixtures show additive effects, but more research integrating metabolomics is warranted for validation of the systemic effects.
ZEN + sterigmatocystin (STE)
Monitoring endogenous metabolites in cattle urine samples revealed ZEN and STE mixtures may affect amino acids, purine bases and metabolites involved in ATP generation. Toda et al. performed an extensive study on the effect of ZEN and STE on Japanese black cattle in order to identify the chronic combinatory effect exerted on the biological system. GC-MS was the employed analytical tool. The outcome of the study suggests that the altered metabolite profile due to exposure to ZEN + STE could be categorized broadly into five major classes: (a) energy metabolism, (b) amino acid metabolism, (c) glycine conjugation, (d) purine base metabolism and (e) metabolites derived from dietary components. However, noticeably fluctuating metabolites were the ones involved in amino acid metabolism, purine base metabolism and energy metabolism. Changes were also reported in essential amino acids, such as Thr, Lys, and taurine, which are mostly obtained from diet and are required for important physiological functions. Further alterations in tyrosine and oxoproline were quite interesting as fluctuations in both amino acid levels might affect thyroid hormone synthesis. In addition, levels of tryptophan, phosphocolamine, and serine, which are found to be involved in proper functioning of neurotransmitters (serotonin and melatonin), structural phospholipids and synthesis of purines/pyrimidines, were significantly augmented. These changes in amino acid metabolism can provide a connecting link to the appetite depression in cattle. Furthermore, the increased levels in urine of three metabolites associated with glycine conjugates, that is, hippurate, butyrylglycine, and methylbutyrylglycine, indicate that the toxin mixture may have affected detoxification during phase II, that is, conjugation phase. The observed changes on biomolecules such as ribofuranose, allantoin, β-pseudouridine, and uric acid suggested that the combined mixture of ZEN + STE can cause alterations in RNA, oxidative stress and nitrogen insufficiency. This metabolomic study indicates that co-exposure to ZEN + STE interferes with several metabolic pathways. Moreover, specific studies need to be conducted in order to identify exposure-specific markers () (Toda et al. Citation2017).
Mixtures of multiple mycotoxins
DON + ZEN + AFB1
The metabolic profile analysis of Caco-2 cells by GC-MS revealed considerable effects on amino acid metabolism, organic acid metabolism, and energy metabolism arising from the combinatory application of DON, ZEN, and AFB1. It was concluded that cellular apoptosis or necrosis could be attributed to the synergistic effect of the three mycotoxins on the metabolism () (Ji et al. Citation2019).
DON + AFB1 + FB1 + ZEN + OTA
1H-NMR spectroscopy and multivariate statistical analysis were used to study the metabolomic changes following exposure of piglets to a quintenary mycotoxin mixture. The urinary metabolome showed high concentrations of creatinine, p-cresol glucuronide, and phenyl acetyl glycine 24 h post-treatment and the same pattern was noted after a week following initial treatment, which indicates that a single administration of the mycotoxin mixture causes serious impairment to the overall health as well as gut microbiota. Decrease in the concentrations of betaine and trimethylamine-N-oxide was also observed in urine of treated piglets () (De Pascali et al. Citation2017). A recent study on cow milk was undertaken, where the cows were fed mycotoxin-contaminated corn silage, that is, Aspergillus, Penicillium, fumonisins, and Fusarium contaminations. The contamination profile was categorized into (a) high Aspergillus and Penicillium mycotoxins, (b) low fumonisins and Fusarium mycotoxins, (c) high Aspergillus mycotoxins, (d) high unregulated Fusarium mycotoxins and (e) high fumonisins and their metabolites. The untargeted metabolomics analysis of 45 cow milk samples revealed overall alterations in 628 metabolites/features. The authors suggest taking into consideration the chemical deviations in milk as an indication of mycotoxin contamination () (Rocchetti et al. Citation2021).
Data processing and pathway analysis in metabolomics studies
Small biomolecule analysis often provides a detailed picture of the organism’s current state of health/disease, which helps in establishing a plausible connection between an individual’s genotype and phenotype. Metabolomics allows insights into fundamental biological processes. To derive useful information from large amount of machine generated data, a stringent quality control with preprocessing of the data is essential in order to extract valuable knowledge about biological processes. The handling, processing, analysis, and integration of these data require specialized mathematical models, statistical and bioinformatics tools. Frequently used platforms include MS-Dial, MZmine 2, XCMS Online, Metaboanalyst, MarkerView, and Compound Discoverer. The information about metabolite patterns is used to obtain associations with molecular networks regulating important physiological functions. In case of the metabolomics studies conducted to evaluate the effects of mycotoxins, the data has been mostly examined by PCA coupled with OPLS-DA/PLS-DA followed by metabolite integrated pathway analysis. It has been noticed the metabolomics studies with mycotoxins have widely used the combination of Metaboanalyst and Cytospace for pathway analysis from the generated data (Gao et al. Citation2022; Ji Ji, Zhu, Cui, Pi, Zhang, Li, et al. Citation2017; Xu et al. Citation2019). However, there is an expanding set of tools that can be taken into consideration when planning and executing metabolomics based pathway studies. The implementation of the right combination of the aforementioned tools can provide a better picture of affected metabolic status as a consequence of mycotoxin exposure and requires careful consideration.
Use of metabolomics in regulatory mycotoxicology
Metabolomic studies have aided with remarkable efficacy, mechanistic insight, and real-time knowledge of the physiological status of biological systems, which reflects the state of exact health. The metabolomic branch of the “omics” disciplines has broadened the horizon of knowledge on biochemical routes, unveiled their mode of action, and provided new potential targets that can be exploited for the early identification of disorders or for therapeutic interventions. We are often exposed to several xenobiotics/toxicants in our daily life. The application of metabolomics has enabled a clearer view of the pathways involved in the pathophysiological conditions mediated by mycotoxins. Briefly, metabolomics can provide knowledge about (a) mycotoxin metabolism, (b) their interaction with biomolecules, (c) points of departure, (d) target organ system, (e) intraspecies variability, (f) potential exposure biomarkers, (g) biological/biochemical pathway analysis, (h) connections and crosstalks, and (i) determination of NOAELs (no-observed-adverse-effect level). The use of metabolomics for the assessment of clinical samples, that is, biofluids, is highly fruitful; however, human variability is a limiting factor that can be minimized by increasing sample size and defining appropriate categorization of the study sets.
The metabolomic approach allows the accumulation of information about pathways/mode of action of different mycotoxins but there are few common variables in this type of study, namely technical and biological. The technical variability is due to differences in the standard operating procedures (SOPs) on sample preparation, separation of metabolites, and their detection, and analysis. The biological variability may be due to the use of different species as study models, different environmental factors, and dietary factors. Therefore, before concrete SOPs are established to carry out metabolomic studies it is suggested to use the information derived from metabolomic experiments for academic assessments. Furthermore, in the near future, this information can be used by the regulatory bodies in order to limit the presence of mycotoxins in food.
Despite the numerous advantages of metabolomics, there are challenges that arise with regard to large experimental groups and data sets, namely (a) potential inter individual variation, (b) choice of instrumentation and combination of techniques to be used for the study, (c) lack of standard operating procedures, (d) identification and quantification of biomolecules, (e) identification of molecules as biomarkers and (e) statistical analysis (Di Minno et al. Citation2022; Johnson and Gonzalez Citation2012).
Conclusions
Metabolomics provides a real-time picture of the pathophysiological state mediated by mycotoxin exposure and leads to the path of discovering exposure biomarkers associated with mycotoxin-mediated adverse implications. The high throughput application of metabolomics provides insight into metabolic mechanisms and pathways potentially associated with health risks and disease outcomes. Furthermore, clinical applications for identifying mycotoxin-mediated pathology and therapeutic targets, as well as for developing mycotoxin-specific metabolic databases can also be achieved/realized with the help of metabolomics-derived information. Overall, the metabolic state of any biological system conveys the accurate picture of the pathophysiological condition; therefore, metabolomics is an important tool that will reveal suitable targets upon rigorous analysis. The use of this tool for mycotoxin-mediated pathologies is still in the early stages but possesses great potential which will help in establishing necessary regulations to ensure the protection of humans as well as livestock from adverse health effects.
Abbreviations | ||
AP1 | = | Activator protein 1 |
AF | = | Aflatoxin |
Ala | = | Alanine |
Arg | = | Arginine |
Asp | = | Aspartic acid |
ATA | = | Alimentary toxic aleukia |
BRL-3A | = | Buffalo rat liver cells |
Caco-2 | = | Caucasian colon adenocarcinoma |
CE | = | Capillary electrophoresis |
COX-2 | = | Cyclooxygenase 2 |
CerS | = | Ceramide synthase |
DON | = | Deoxynivalenol |
DOM-1 | = | Deepoxy-deoxynivalenol |
DM | = | Dry matter |
DNA | = | Deoxyribonucleic acid |
DMG | = | Dimethylglycine |
ESI | = | Electrospray ionization |
Fe2+ | = | Ferrous ion |
FE | = | Facial eczema |
GABA | = | γ-Aminobutyric acid |
GC/MS | = | Gas chromatography-mass spectrometry |
Gly | = | Glycine |
Glu | = | Glutamic acid |
Gln | = | Glutamine |
GPC | = | Glycerophosphocholine |
GSH | = | Glutathione |
GSSG | = | Glutathione disulfide |
HACCP | = | Hazard analysis and critical control point |
His | = | Histadine |
HRMAS | = | High-resolution magnetic angle spin |
HRMS | = | High-resolution mass spectrometry |
IgA | = | Immunoglobulin A |
IgE | = | Immunoglobulin E |
IL | = | Interleukin |
Ile | = | Isoleucine |
IARC | = | International agency for research and cancer |
KBD | = | Kashchin-Beck disease |
LC/MS | = | Liquid chromatography-mass spectrometry |
LC-MS/MS | = | Liquid chromatography-tandem mass spectrometry |
Leu | = | Leucine |
LOD | = | Limit of detection |
LOQ | = | Limit of quantification |
Lys | = | Lysine |
MALDI | = | Matrix-assisted laser desorption/ionization |
MAPK | = | Mitogen-activated protein kinase |
Met | = | Methionine |
miR | = | Micro RNA |
MNA | = | 1-methylnicotinamide |
NMR | = | Nuclear magnetic resonance |
NFκB | = | Nuclear factor-kappa B |
OTA | = | Ochratoxin A |
OTQ | = | Ochratoxin A-quinone |
OTHQ | = | Ochratoxin A-hydroquinone |
PAT | = | Patulin |
PC | = | Phosphocholine |
PC | = | Phosphatidylcholine |
PE | = | Phosphatidylethanolamine |
Phe | = | Phenylalanine |
PPP | = | Pentose phosphate pathway |
ROS | = | Reactive oxygen species |
rRNA | = | Ribosomal ribonucleic acid |
Ser | = | Serine |
SOP | = | Standard operating procedure |
STE | = | Sterigmatocystin |
T2-3-Glc | = | T2-3-glucoside |
T2-3-Glca | = | T-2-3-glucuronide |
TCA | = | Tricarboxylic acid |
Thr | = | Threonine |
TOF | = | Time of flight |
TMAO | = | Trimethylamine-N-oxide |
TNF | = | Tumour necrosis factor |
tRNA | = | Transfer RNA |
Trp | = | Tryptophan |
Tyr | = | Tyrosine |
UPLC | = | Ultra performance liquid chromatography |
Val | = | Valine |
ZEN | = | Zearalenone |
ZEL | = | Zearalenol |
ZAL | = | Zearelanol |
Acknowledgements
Authors are grateful to the Director of CSIR-Indian Institute of Toxicology Research, Lucknow. KMA is thankful to the Council of Scientific and Industrial Research, New Delhi for “CSIR Fellowship Abroad Raman Research Fellowship Grant P-80104.” The institutional manuscript communication number is IITR/SEC/MS/2023/13.
Disclosure statement
The authors declare that they have no known competing financial interests or personal relationships that could have appeared to influence the work reported in this paper.
The authors declare the following financial interests/personal relationships which may be considered as potential competing interests:
Kausar Mahmood Ansari:
Correction Statement
This article has been republished with minor changes. These changes do not impact the academic content of the article.
Additional information
Funding
References
- Adhikari, M., B. Negi, N. Kaushik, A. Adhikari, A. A. Al-Khedhairy, N. K. Kaushik, and E. H. Choi. 2017. T-2 mycotoxin: Toxicological effects and decontamination strategies. Oncotarget 8 (20):33933–52. doi: 10.18632/oncotarget.15422.
- Agriopoulou, S., E. Stamatelopoulou, and T. Varzakas. 2020. Advances in occurrence, importance, and mycotoxin control strategies: Prevention and detoxification in foods. Foods 9 (2):137. doi: 10.3390/foods9020137.
- Aichinger, G., G. Del Favero, B. Warth, and D. Marko. 2021. Alternaria toxins—Still emerging? Comprehensive Reviews in Food Science and Food Safety 20 (5):4390–406. doi: 10.1111/1541-4337.12803.
- Awuchi, C. G., E. N. Ondari, C. U. Ogbonna, A. K. Upadhyay, K. Baran, C. O. R. Okpala, M. Korzeniowska, and R. P. Guiné. 2021. Mycotoxins affecting animals, foods, humans, and plants: Types, occurrence, toxicities, action mechanisms, prevention, and detoxification strategies—A revisit. Foods 10 (6):1279. doi: 10.3390/foods10061279.
- Battilani, P.,R. Palumbo,P. Giorni,C. Dall’Asta,L. Dellafiora,A. Gkrillas,P. Toscano,A. Crisci,C. Brera,B. De Santis, et al. 2020. Mycotoxin mixtures in food and feed: holistic, innovative, flexible risk assessment modelling approach. EFSA Supporting Publications 17 (1). doi: 10.2903/sp.efsa.2020.EN-1757.
- Benkerroum, N. 2020. Chronic and acute toxicities of aflatoxins: Mechanisms of action. International Journal of Environmental Research and Public Health 17 (2):423. doi: 10.3390/ijerph17020423.
- Bow, D. A., J. L. Perry, J. D. Simon, and J. B. Pritchard. 2006. The impact of plasma protein binding on the renal transport of organic anions. The Journal of Pharmacology and Experimental Therapeutics 316 (1):349–55. doi: 10.1124/jpet.105.093070.
- Brera, C., B. de Santis, F. Debegnach, B. Miano, G. Moretti, A. Lanzone, G. Del Sordo, D. Buonsenso, A. Chiaretti, L. Hardie, et al. 2015. Experimental study of deoxynivalenol biomarkers in urine. EFSA Supporting Publications 12 (6):818E. doi: 10.2903/sp.efsa.2015.EN-818.
- Brezina, U., I. Rempe, S. Kersten, H. Valenta, H.-U. Humpf, and S. Dänicke. 2014. Diagnosis of intoxications of piglets fed with fusarium toxin-contaminated maize by the analysis of mycotoxin residues in serum, liquor and urine with lc-ms/ms. Archives of Animal Nutrition 68 (6):425–47. doi: 10.1080/1745039X.2014.973227.
- Bueschl, C., M. Doppler, E. Varga, B. Seidl, M. Flasch, B. Warth, and J. Zanghellini. 2022. Peakbot: Machine-learning-based chromatographic peak picking. Bioinformatics 38 (13):3422–8. doi: 10.1093/bioinformatics/btac344.
- Cheng, J., S. Huang, C. Fan, N. Zheng, Y. Zhang, S. Li, and J. Wang. 2017. Metabolomic analysis of alterations in lipid oxidation, carbohydrate and amino acid metabolism in dairy goats caused by exposure to aflotoxin b1. Journal of Dairy Research 84 (4):401–6. doi: 10.1017/S0022029917000590.
- Cinar, A., and E. Onbaşı. 2019. Mycotoxins: The hidden danger in foods. Mycotoxins and Food Safety 20:1–21. doi: 10.5772/intechopen.89001.
- Dänicke, S., and U. Brezina. 2013. Kinetics and metabolism of the fusarium toxin deoxynivalenol in farm animals: Consequences for diagnosis of exposure and intoxication and carry over. Food and Chemical Toxicology 60:58–75. doi: 10.1016/j.fct.2013.07.017.
- Daou, R., K. Joubrane, R. G. Maroun, L. R. Khabbaz, A. Ismail, and A. E. Khoury. 2021. Mycotoxins: Factors influencing production and control strategies. AIMS Agriculture and Food 6 (1):416–47. doi: 10.3934/agrfood.2021025.
- Dasgupta, S., and S. K. Ray. 2017. Diverse biological functions of sphingolipids in the CNS: Ceramide and sphingosine regulate myelination in developing brain but stimulate demyelination during pathogenesis of multiple sclerosis. Journal of Neurology and Psychology 5 (1): 1–17. doi: 10.13188/2332-3469.1000035.
- De Pascali, S. A., L. Gambacorta, I. P. Oswald, L. Del Coco, M. Solfrizzo, and F. P. Fanizzi. 2017. 1H NMR and MVA metabolomic profiles of urines from piglets fed with boluses contaminated with a mixture of five mycotoxins. Biochemistry and Biophysics Reports 11:9–18. doi: 10.1016/j.bbrep.2017.05.004.
- Delgado, A., J. Casas, A. Llebaria, J. L. Abad, and G. Fabrias. 2006. Inhibitors of sphingolipid metabolism enzymes. Biochimica et Biophysica Acta (BBA) –Biomembranes 1758 (12):1957–77. doi: 10.1016/j.bbamem.2006.08.017.
- Dellafiora, L., and C. Dall’asta. 2017. Forthcoming challenges in mycotoxins toxicology research for safer food—A need for multi-omics approach. Toxins 9 (1):18. doi: 10.3390/toxins9010018.
- Di Minno, A., M. Gelzo, M. Caterino, M. Costanzo, M. Ruoppolo, and G. Castaldo. 2022. Challenges in metabolomics-based tests, biomarkers revealed by metabolomic analysis, and the promise of the application of metabolomics in precision medicine. International Journal of Molecular Sciences 23 (9):5213. doi: 10.3390/ijms23095213.
- Domijan, A.-M., G. Gajski, IN. Jovanović, M. Gerić, and V. Garaj-Vrhovac. 2015. In vitro genotoxicity of mycotoxins ochratoxin a and fumonisin B1 could be prevented by sodium copper chlorophyllin–implication to their genotoxic mechanism. Food Chemistry 170:455–62. doi: 10.1016/j.foodchem.2014.08.036.
- EFSA. 2011. Scientific opinion on the risks for public health related to the presence of zearalenone in food. EFSA Journal 9 (6):2197.
- EFSA 2017. Risks to human and animal health related to the presence of deoxynivalenol and its acetylated and modified forms in food and feed. No 9. EFSA Journal 15: E 04718.
- Ellis, J. K., T. J. Athersuch, R. Cavill, R. Radford, C. Slattery, P. Jennings, T. Mcmorrow, M. P. Ryan, T. M. D. Ebbels, and H. C. Keun. 2011. Metabolic response to low-level toxicant exposure in a novel renal tubule epithelial cell system. Molecular BioSystems 7 (1):247–57. doi: 10.1039/C0MB00146E.
- Eshelli, M., M. M. Qader, E. J. Jambi, A. S. Hursthouse, and M. E. Rateb. 2018. Current status and future opportunities of omics tools in mycotoxin research. Toxins 10 (11):433. doi: 10.3390/toxins10110433.
- Eskola, M., G. Kos, C. T. Elliott, J. Hajšlová, S. Mayar, and R. Krska. 2020. Worldwide contamination of food-crops with mycotoxins: Validity of the widely cited ‘FAO estimate’ of 25%. Critical Reviews in Food Science and Nutrition 60 (16):2773–89. doi: 10.1080/10408398.2019.1658570.
- Flasch, M., C. Bueschl, G. Del Favero, G. Adam, R. Schuhmacher, D. Marko, and B. Warth. 2022. Elucidation of xenoestrogen metabolism by non-targeted, stable isotope-assisted mass spectrometry in breast cancer cells. Environment International 158:106940. doi: 10.1016/j.envint.2021.106940.
- Flasch, M., C. Bueschl, L. Woelflingseder, H. E. Schwartz-Zimmermann, G. Adam, R. Schuhmacher, D. Marko, and B. Warth. 2020. Stable isotope-assisted metabolomics for deciphering xenobiotic metabolism in mammalian cell culture. ACS Chemical Biology 15 (4):970–81. doi: 10.1021/acschembio.9b01016.
- Fremy, J.-M., I. Alassane-Kpembi, I. P. Oswald, B. Cottrill, and H. Van Egmond. 2019. A review on combined effects of moniliformin and co-occurring fusarium toxins in farm animals. World Mycotoxin Journal 12 (3):281–91. doi: 10.3920/WMJ2018.2405.
- Frizzell, C., D. Ndossi, S. Verhaegen, E. Dahl, G. Eriksen, M. Sørlie, E. Ropstad, M. Muller, C. Elliott, and L. Connolly. 2011. Endocrine disrupting effects of zearalenone, alpha-and beta-zearalenol at the level of nuclear receptor binding and steroidogenesis. Toxicology Letters 206 (2):210–7. doi: 10.1016/j.toxlet.2011.07.015.
- Gao, Y.-N., C.-Q. Wu, J.-Q. Wang, and N. Zheng. 2022. Metabolomic analysis reveals the mechanisms of hepatotoxicity induced by aflatoxin M1 and ochratoxin A. Toxins 14 (2):141. doi: 10.3390/toxins14020141.
- Gelderblom, W., W. Marasas, S. Lebepe-Mazur, S. Swanevelder, C. Vessey, P. D. La, and M. Hall. 2002. Interaction of fumonisin b1 and aflatoxin b1 in a short-term carcinogenesis model in rat liver. Toxicology 171 (2-3):161–73. doi: 10.1016/s0300-483x(01)00573-x.
- Gelineau‐Van Waes, J., K. Voss, V. Stevens, M. Speer, and R. Riley. 2009. Maternal fumonisin exposure as a risk factor for neural tube defects. Advances in Food and Nutrition Research 56:145–81.
- Gizaw, Z. 2019. Public health risks related to food safety issues in the food market: A systematic literature review. Environmental Health and Preventive Medicine 24 (1):21. doi: 10.1186/s12199-019-0825-5.
- Guengerich, F. P., K. O. Arneson, K. M. Williams, Z. Deng, and T. M. Harris. 2002. Reaction of aflatoxin b1 oxidation products with lysine. Chemical Research in Toxicology 15 (6):780–92. doi: 10.1021/tx010156s.
- Hopton, R. P., E. Turner, V. J. Burley, P. C. Turner, and J. Fisher. 2010. Urine metabolite analysis as a function of deoxynivalenol exposure: An NMR-based metabolomics investigation. Food Additives & Contaminants. Part A, Chemistry, Analysis, Control, Exposure & Risk Assessment 27 (2):255–61. doi: 10.1080/19440040903314015.
- IARC. 2016. New IARC report urges action against widespread mycotoxin contamination in developing countries. Lyon: IARC, Press release N: 242.
- Janik, E., M. Niemcewicz, M. Ceremuga, M. Stela, J. Saluk-Bijak, A. Siadkowski, and M. Bijak. 2020. Molecular aspects of mycotoxins—A serious problem for human health. International Journal of Molecular Sciences 21 (21):8187. doi: 10.3390/ijms21218187.
- Ji, J., J. Sun, F. Pi, S. Zhang, C. Sun, X. Wang, Y. Zhang, and X. Sun. 2016. GC-TOF/MS-based metabolomics approach to study the cellular immunotoxicity of deoxynivalenol on murine macrophage ANA-1 cells. Chemico-Biological Interactions 256:94–101. doi: 10.1016/j.cbi.2016.06.017.
- Ji, J., Q. Wang, H. Wu, S. Xia, H. Guo, I. Blaženović, Y. Zhang, and X. Sun. 2019. Insights into cellular metabolic pathways of the combined toxicity responses of caco-2 cells exposed to deoxynivalenol, zearalenone and aflatoxin B1. Food and Chemical Toxicology: An International Journal Published for the British Industrial Biological Research Association 126:106–12. doi: 10.1016/j.fct.2018.12.052.
- Ji, J., P. Zhu, I. Blaženović, F. Cui, M. Gholami, J. Sun, J. Habimana, Y. Zhang, and X. Sun. 2018. Explaining combinatorial effects of mycotoxins deoxynivalenol and zearalenone in mice with urinary metabolomic profiling. Scientific Reports 8 (1):3762. doi: 10.1038/s41598-018-21555-y.
- Ji, J., P. Zhu, F. Cui, F. Pi, Y. Zhang, Y. Li, J. Wang, and X. Sun. 2017. The antagonistic effect of mycotoxins deoxynivalenol and zearalenone on metabolic profiling in serum and liver of mice. Toxins 9 (1):28. doi: 10.3390/toxins9010028.
- Ji, J., P. Zhu, F. Cui, F. Pi, Y. Zhang, and X. Sun. 2017. The disorder metabolic profiling in kidney and spleen of mice induced by mycotoxins deoxynivalenol through gas chromatography mass spectrometry. Chemosphere 180:267–74. doi: 10.1016/j.chemosphere.2017.03.129.
- Ji, J., P. Zhu, F. Pi, C. Sun, H. Jiang, J. Sun, X. Wang, Y. Zhang, and X. Sun. 2016. GC-TOF/MS-based metabolomic strategy for combined toxicity effects of deoxynivalenol and zearalenone on murine macrophage ANA-1 cells. Toxicon: Official Journal of the International Society on Toxinology 120:175–84. doi: 10.1016/j.toxicon.2016.08.003.
- Johnson, C. H., and F. J. Gonzalez. 2012. Challenges and opportunities of metabolomics. Journal of Cellular Physiology 227 (8):2975–81. doi: 10.1002/jcp.24002.
- Johnson, C. H., J. Ivanisevic, and G. Siuzdak. 2016. Metabolomics: Beyond biomarkers and towards mechanisms. Nature Reviews. Molecular Cell Biology 17 (7):451–9. doi: 10.1038/nrm.2016.25.
- Juan-García, A., S. Carbone, M. Ben-Mahmoud, G. Sagratini, and J. Mañes. 2020. Beauvericin and ochratoxin a mycotoxins individually and combined in Hepg2 cells alter lipid peroxidation, levels of reactive oxygen species and glutathione. Food and Chemical Toxicology: An International Journal Published for the British Industrial Biological Research Association 139:111247. doi: 10.1016/j.fct.2020.111247.
- Kamle, M., D. K. Mahato, S. Devi, K. E. Lee, S. G. Kang, and P. Kumar. 2019. Fumonisins: Impact on agriculture, food, and human health and their management strategies. Toxins 11 (6):328. doi: 10.3390/toxins11060328.
- Kasimir, M., M. Behrens, M. Schulz, H. Kuchenbuch, C. Focke, and H.-U. Humpf. 2020. Intestinal metabolism of α-and β-glucosylated modified mycotoxins T-2 and HT-2 toxin in the pig cecum model. Journal of Agricultural and Food Chemistry 68 (19):5455–61. doi: 10.1021/acs.jafc.0c00576.
- Kőszegi, T., and M. Poór. 2016. Ochratoxin a: Molecular interactions, mechanisms of toxicity and prevention at the molecular level. Toxins 8 (4):111. doi: 10.3390/toxins8040111.
- Kumar, R., S. Alam, B. P. Chaudhari, P. D. Dwivedi, S. K. Jain, K. M. Ansari, and M. Das. 2013. Ochratoxin a-induced cell proliferation and tumor promotion in mouse skin by activating the expression of cyclin-D1 and cyclooxygenase-2 through nuclear factor-kappa B and activator protein-1. Carcinogenesis 34 (3):647–57. doi: 10.1093/carcin/bgs368.
- Kumar, R., K. M. Ansari, B. P. Chaudhari, A. Dhawan, P. D. Dwivedi, S. K. Jain, and M. Das. 2012. Topical application of ochratoxin a causes DNA damage and tumor initiation in mouse skin.
- Lai, F.-N., X.-L. Liu, N. Li, R.-Q. Zhang, Y. Zhao, Y.-Z. Feng, C. M. Nyachoti, W. Shen, and L. Li. 2018. Phosphatidylcholine could protect the defect of zearalenone exposure on follicular development and oocyte maturation. Aging 10 (11):3486–506. doi: 10.18632/aging.101660.
- Lei, M., N. Zhang, and D. Qi. 2013. In vitro investigation of individual and combined cytotoxic effects of aflatoxin b1 and other selected mycotoxins on the cell line porcine kidney 15. Experimental and Toxicologic Pathology: Official Journal of the Gesellschaft fur Toxikologische Pathologie 65 (7-8):1149–57. doi: 10.1016/j.etp.2013.05.007.
- Lei, Z. J., Zhao, X. B. Ren, L. Qiang, D. Hua, S. Zhou, Q. J. Kao, and L. H. Wang. 2018. Serum metabonomics of articular cartilage destruction induced by t-2 toxin in wistar rats. Biomedical and Environmental Sciences 31 (1):76–80.
- Li, Z., Y. Lu, Y. Guo, H. Cao, Q. Wang, and W. Shui. 2018. Comprehensive evaluation of untargeted metabolomics data processing software in feature detection, quantification and discriminating marker selection. Analytica Chimica Acta 1029:50–7. doi: 10.1016/j.aca.2018.05.001.
- Liu, G., T. Yan, J. Wang, Z. Huang, X. Chen, G. Jia, C. Wu, H. Zhao, B. Xue, L. Xiao, et al. 2013. Biological system responses to zearalenone mycotoxin exposure by integrated metabolomic studies. Journal of Agricultural and Food Chemistry 61 (46):11212–21. doi: 10.1021/jf403401v.
- Liu, Y., and F. Wu. 2010. Global burden of aflatoxin-induced hepatocellular carcinoma: A risk assessment. Environmental Health Perspectives 118 (6):818–24. doi: 10.1289/ehp.0901388.
- Lu, X., B. Hu, L. Shao, Y. Tian, T. Jin, Y. Jin, S. Ji, and X. Fan. 2013. Integrated analysis of transcriptomics and metabonomics profiles in aflatoxin B1-induced hepatotoxicity in rat. Food and Chemical Toxicology: An International Journal Published for the British Industrial Biological Research Association 55:444–55. doi: 10.1016/j.fct.2013.01.020.
- Magnussen, A., and M. A. Parsi. 2013. Aflatoxins, hepatocellular carcinoma and public health. World Journal of Gastroenterology 19 (10):1508–12. doi: 10.3748/wjg.v19.i10.1508.
- Makowska, K., K. Obremski, and S. Gonkowski. 2018. The impact of T-2 toxin on vasoactive intestinal polypeptide-like immunoreactive (Vip-li) nerve structures in the wall of the porcine stomach and duodenum. Toxins 10 (4):138. doi: 10.3390/toxins10040138.
- Malachová, A., M. Stránská, M. Václavíková, C. T. Elliott, C. Black, J. Meneely, J. Hajšlová, C. N. Ezekiel, R. Schuhmacher, and R. Krska. 2018. Advanced LC–MS-based methods to study the co-occurrence and metabolization of multiple mycotoxins in cereals and cereal-based food. Analytical and Bioanalytical Chemistry 410 (3):801–25. doi: 10.1007/s00216-017-0750-7.
- Malir, F., M. Louda, J. Toman, V. Ostry, D. Pickova, J. Pacovsky, M. Brodak, and A. Pfohl-Leszkowicz. 2021. Investigation of ochratoxin a biomarkers in biological materials obtained from patients suffering from renal cell carcinoma. Food and Chemical Toxicology: An International Journal Published for the British Industrial Biological Research Association 158:112669. doi: 10.1016/j.fct.2021.112669.
- Mantle, P. G., A. W. Nicholls, and J. P. Shockcor. 2011. 1H. NMR spectroscopy-based metabolomic assessment of uremic toxicity, with toxicological outcomes, in male rats following an acute, mid-life insult from ochratoxin A. Toxins 3 (6):504–19. doi: 10.3390/toxins3060504.
- Mary, V. S., S. L. Arias, S. N. Otaiza, P. A. Velez, H. R. Rubinstein, and M. G. Theumer. 2017. The aflatoxin B1‐fumonisin B1 toxicity in BRL‐3a hepatocytes is associated to induction of cytochrome p450 activity and arachidonic acid metabolism. Environmental Toxicology 32 (6):1711–24. doi: 10.1002/tox.22395.
- Matthews, Z. M., P. J. Edwards, A. Kahnt, M. G. Collett, J. C. Marshall, A. C. Partridge, S. J. Harrison, K. Fraser, M. Cao, and P. J. Derrick. 2018. Serum metabolomics using ultra performance liquid chromatography coupled to mass spectrometry in lactating dairy cows following a single dose of sporidesmin. Metabolomics 14 (5):1–14. doi: 10.1007/s11306-018-1358-4.
- Miró-Abella, E., H. Torrell, P. Herrero, N. Canela, L. Arola, F. Borrull, R. Ras, and N. Fontanals. 2018. Monitoring and evaluation of the interaction between deoxynivalenol and gut microbiota in wistar rats by mass spectrometry-based metabolomics and next-generation sequencing. Food and Chemical Toxicology: An International Journal Published for the British Industrial Biological Research Association 121:124–30. doi: 10.1016/j.fct.2018.08.006.
- Muthubharathi, B. C., T. Gowripriya, and K. Balamurugan. 2021. Metabolomics: Small molecules that matter more. Molecular Omics 17 (2):210–29. doi: 10.1039/d0mo00176g.
- Nagl, V., B. Grenier, P. Pinton, U. Ruczizka, M. Dippel, M. Bünger, I. P. Oswald, and L. Soler. 2021. Exposure to zearalenone leads to metabolic disruption and changes in circulating adipokines concentrations in pigs. Toxins 13 (11):790. doi: 10.3390/toxins13110790.
- Nittoli, A. C., S. Costantini, A. Sorice, F. Capone, R. Ciarcia, S. Marzocco, A. Budillon, and L. Severino. 2018. Effects of α-zearalenol on the metabolome of two breast cancer cell lines by 1H-NMR approach. Metabolomics 14 (3):1–11. doi: 10.1007/s11306-018-1330-3.
- Oliver, S. G., M. K. Winson, D. B. Kell, and F. Baganz. 1998. Systematic functional analysis of the yeast genome. Trends in Biotechnology 16 (9):373–8. doi: 10.1016/s0167-7799(98)01214-1.
- Olsen, M. E., H. I. Pettersson, K. A. Sandholm, and K.-H. C. Kiessling. 1985. Quantitative liquid chromatographic method using fluorescence detection for determining zearalenone and its metabolites in blood plasma and urine. Journal of AOAC INTERNATIONAL 68 (4):632–5. doi: 10.1093/jaoac/68.4.632.
- Ostry, V., F. Malir, J. Toman, and Y. Grosse. 2017. Mycotoxins as human carcinogens—the iarc monographs classification. Mycotoxin Research 33 (1):65–73. doi: 10.1007/s12550-016-0265-7.
- Pal, S., D. Rendedula, N. K. Nagendla, M. Kaliyaperumal, M. K. R. Mudiam, and K. M. Ansari. 2022. Serum and urine metabolomics analysis reveals the role of altered metabolites in patulin-induced nephrotoxicity. Food Research International (Ottawa, Ont.) 156:111177. doi: 10.1016/j.foodres.2022.111177.
- Pal, S., N. Singh, and K. M. Ansari. 2017. Toxicological effects of patulin mycotoxin on the mammalian system: An overview. Toxicology Research 6 (6):764–71. doi: 10.1039/c7tx00138j.
- Peng, B., H. Li, and X.-X. Peng. 2015. Functional metabolomics: From biomarker discovery to metabolome reprogramming. Protein & Cell 6 (9):628–37. doi: 10.1007/s13238-015-0185-x.
- Pestka, J. J. 2010. Deoxynivalenol-induced proinflammatory gene expression: Mechanisms and pathological sequelae. Toxins 2 (6):1300–17. doi: 10.3390/toxins2061300.
- Pfeiffer, E., A. Hildebrand, H. Mikula, and M. Metzler. 2010. Glucuronidation of zearalenone, zeranol and four metabolites in vitro: Formation of glucuronides by various microsomes and human UDP‐glucuronosyltransferase isoforms. Molecular Nutrition & Food Research 54 (10):1468–76. doi: 10.1002/mnfr.200900524.
- Pfohl‐Leszkowicz, A., and R. A. Manderville. 2007. Ochratoxin A: An overview on toxicity and carcinogenicity in animals and humans. Molecular Nutrition & Food Research 51 (1):61–99. doi: 10.1002/mnfr.200600137.
- Pristner, M., and B. Warth. 2020. Drug–exposome interactions: The next frontier in precision medicine. Trends in Pharmacological Sciences 41 (12):994–1005. doi: 10.1016/j.tips.2020.09.012.
- Richard-Forget, F., V. Atanasova, and S. Chéreau. 2021. Using metabolomics to guide strategies to tackle the issue of the contamination of food and feed with mycotoxins: A review of the literature with specific focus on Fusarium mycotoxins. Food Control 121:107610. doi: 10.1016/j.foodcont.2020.107610.
- Rivlin, N., R. Brosh, M. Oren, and V. Rotter. 2011. Mutations in the p53 tumor suppressor gene: Important milestones at the various steps of tumorigenesis. Genes & Cancer 2 (4):466–74. doi: 10.1177/1947601911408889.
- Rocchetti, G., F. Ghilardelli, P. Bonini, L. Lucini, F. Masoero, and A. Gallo. 2021. Changes of milk metabolomic profiles resulting from a mycotoxins-contaminated corn silage intake by dairy cows. Metabolites 11 (8):475. doi: 10.3390/metabo11080475.
- Romero, A., I. Ares, E. Ramos, V. Castellano, M. Martínez, M.-R. Martínez-Larrañaga, A. Anadón, and M.-A. Martínez. 2016. Mycotoxins modify the barrier function of Caco-2 cells through differential gene expression of specific claudin isoforms: Protective effect of illite mineral clay. Toxicology 353-354:21–33. doi: 10.1016/j.tox.2016.05.003.
- Ropejko, K., and M. Twarużek. 2021. Zearalenone and its metabolites—general overview, occurrence, and toxicity. Toxins 13 (1):35. doi: 10.3390/toxins13010035.
- Scheie, E., B. Smith, N. Cox, and A. Flåøyen. 2003. Spectrofluorometric analysis of phylloerythrin (phytoporphyrin) in plasma and tissues from sheep suffering from facial eczema. New Zealand Veterinary Journal 51 (3):104–10. doi: 10.1080/00480169.2003.36348.
- Segers, K., S. Declerck, D. Mangelings, Y. V. Heyden, and A. V. Eeckhaut. 2019. Analytical techniques for metabolomic studies: A review. Bioanalysis 11 (24):2297–318. doi: 10.4155/bio-2019-0014.
- Sieber, M. 2009. Evaluation of 1h-nmr and gc/ms-based metabonomics for the assessment of liver and kidney toxicity. Germany: Universität Würzburg.
- Singh, N., G. Sharma, I. Dev, S. K. Shukla, and K. M. Ansari. 2021. Study of the metabolic alterations in patulin-induced neoplastic transformation in normal intestinal cells. Toxicology Research 10 (3):592–600. doi: 10.1093/toxres/tfab023.
- Storm, I. M. D., R. R. Rasmussen, and P. H. Rasmussen. 2014. Occurrence of pre-and post-harvest mycotoxins and other secondary metabolites in Danish maize silage. Toxins 6 (8):2256–69. doi: 10.3390/toxins6082256.
- Studer-Rohr, I., J. Schlatter, and D. R. Dietrich. 2000. Kinetic parameters and intraindividual fluctuations of ochratoxin a plasma levels in humans. Archives of Toxicology 74 (9):499–510. doi: 10.1007/s002040000157.
- Sun, L.-H., M.-Y. Lei, N.-Y. Zhang, X. Gao, C. Li, C. S. Krumm, and D.-S. Qi. 2015. Individual and combined cytotoxic effects of aflatoxin B1, zearalenone, deoxynivalenol and fumonisin B1 on BRL 3A rat liver cells. Toxicon: Official Journal of the International Society on Toxinology 95:6–12. doi: 10.1016/j.toxicon.2014.12.010.
- Sun, F., H. Tan, Y. Li, M. De Boevre, S. De Saeger, J. Zhou, Y. Li, Z. Rao, S. Yang, and H. Zhang. 2019. Metabolic profile, bioavailability and toxicokinetics of zearalenone-14-glucoside in rats after oral and intravenous administration by liquid chromatography high-resolution mass spectrometry and tandem mass spectrometry. International Journal of Molecular Sciences 20 (21):5473. doi: 10.3390/ijms20215473.
- Toda, K., E. Kokushi, S. Uno, A. Shiiba, H. Hasunuma, Y. Fushimi, M. Wijayagunawardane, C. Zhang, O. Yamato, M. Taniguchi, et al. 2017. Gas chromatography-mass spectrometry for metabolite profiling of Japanese black cattle naturally contaminated with zearalenone and sterigmatocystin. Toxins 9 (10):294. doi: 10.3390/toxins9100294.
- Vidal, A., G. Cano-Sancho, S. Marin, A. Ramos, and V. Sanchis. 2016. Multidetection of urinary ochratoxin a, deoxynivalenol and its metabolites: Pilot time-course study and risk assessment in Catalonia, Spain. World Mycotoxin Journal 9 (4):597–612. doi: 10.3920/WMJ2015.2006.
- Voss, K. A., and R. T. Riley. 2013. Fumonisin toxicity and mechanism of action: Overview and current perspectives. Food Safety 1 (1):2013006– doi: 10.14252/foodsafetyfscj.2013006.
- Wan, Q., Q. He, X. Deng, F. Hao, H. Tang, and Y. Wang. 2016. Systemic metabolic responses of broiler chickens and piglets to acute T-2 toxin intravenous exposure. Journal of Agricultural and Food Chemistry 64 (3):714–23. doi: 10.1021/acs.jafc.5b05076.
- Wan, Q., G. Wu, Q. He, H. Tang, and Y. Wang. 2015. The toxicity of acute exposure to t-2 toxin evaluated by the metabonomics technique. Molecular Biosystems 11 (3):882–91. doi: 10.1039/c4mb00622d.
- Wang, X., M. Fan, X. Chu, Y. Zhang, S. U. Rahman, Y. Jiang, X. Chen, D. Zhu, S. Feng, Y. Li, et al. 2018. Deoxynivalenol induces toxicity and apoptosis in piglet hippocampal nerve cells via the mapk signaling pathway. Toxicon: Official Journal of the International Society on Toxicology 155:1–8. doi: 10.1016/j.toxicon.2018.09.006.
- Wang, Q., Y. Zhang, N. Zheng, L. Guo, X. Song, S. Zhao, and J. Wang. 2019. Biological system responses of dairy cows to aflatoxin B1 exposure revealed with metabolomic changes in multiple biofluids. Toxins 11 (2):77. doi: 10.3390/toxins11020077.
- Wang, W., Y. Zhu, N. Abraham, X.-Z. Li, M. Kimber, and T. Zhou. 2021. The ribosome-binding mode of trichothecene mycotoxins rationalizes their structure—activity relationships. International Journal of Molecular Sciences 22 (4):1604. doi: 10.3390/ijms22041604.
- Warth, B., K. Preindl, P. Manser, P. Wick, D. Marko, and T. Buerki-Thurnherr. 2019. Transfer and metabolism of the xenoestrogen zearalenone in human perfused placenta. Environmental Health Perspectives 127 (10):107004. doi: 10.1289/EHP4860.
- Warth, B., P. Raffeiner, A. Granados, T. Huan, M. Fang, E. M. Forsberg, H. P. Benton, L. Goetz, C. H. Johnson, and G. Siuzdak. 2018. Metabolomics reveals that dietary xenoestrogens alter cellular metabolism induced by palbociclib/letrozole combination cancer therapy. Cell Chemical Biology 25 (3):291–300. e3. doi: 10.1016/j.chembiol.2017.12.010.
- Wild, C. P., and Y. Y. Gong. 2010. Mycotoxins and human disease: A largely ignored global health issue. Carcinogenesis 31 (1):71–82. doi: 10.1093/carcin/bgp264.
- Wu, M., H. Xiao, W. Ren, J. Yin, J. Hu, J. Duan, G. Liu, B. Tan, X. Xiong, A. O. Oso, et al. 2014. An NMR-based metabolomic approach to investigate the effects of supplementation with glutamic acid in piglets challenged with deoxynivalenol. PloS One 9 (12):e113687. doi: 10.1371/journal.pone.0113687.
- Xia, K., X. He, Q. Dai, W.-H. Cheng, X. Qi, M. Guo, Y. Luo, K. Huang, C. Zhao, and W. Xu. 2014. Discovery of systematic responses and potential biomarkers induced by ochratoxin a using metabolomics. Food Additives & Contaminants. Part A, Chemistry, Analysis, Control, Exposure & Risk Assessment 31 (11):1904–13. doi: 10.1080/19440049.2014.957249.
- Xu, Y., J. Ji, H. Wu, F. Pi, I. Blaženović, Y. Zhang, and X. Sun. 2019. Untargeted GC-TOFMS-based cellular metabolism analysis to evaluate ozone degradation effect of deoxynivalenol. Toxicon: Official Journal of the International Society on Toxicology 168:49–57. doi: 10.1016/j.toxicon.2019.06.022.
- Zanic-Grubisić, T., R. Zrinski, I. Cepelak, J. Petrik, B. Radić, and S. Pepeljnjak. 2000. Studies of ochratoxin a-induced inhibition of phenylalanine hydroxylase and its reversal by phenylalanine. Toxicology and Applied Pharmacology 167 (2):132–9. doi: 10.1006/taap.2000.8987.
- Zhang, L., Y. Ye, Y. An, Y. Tian, Y. Wang, and H. Tang. 2011. Systems responses of rats to aflatoxin B1 exposure revealed with metabonomic changes in multiple biological matrices. Journal of Proteome Research 10 (2):614–23. doi: 10.1021/pr100792q.
- Zöllner, P., and B. Mayer-Helm. 2006. Trace mycotoxin analysis in complex biological and food matrices by liquid chromatography–atmospheric pressure ionisation mass spectrometry. Journal of Chromatography A 1136 (2):123–69. doi: 10.1016/j.chroma.2006.09.055.
- Zuberi, Z., M. N. Eeza, J. Matysik, J. P. Berry, and A. Alia. 2019. NMR-based metabolic profiles of intact zebrafish embryos exposed to aflatoxin B1 recapitulates hepatotoxicity and supports possible neurotoxicity. Toxins 11 (5):258. doi: 10.3390/toxins11050258.