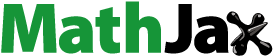
Abstract
The SSB protein of Escherichia coli functions to bind single-stranded DNA wherever it occurs during DNA metabolism. Depending upon conditions, SSB occurs in several different binding modes. In the course of its function, SSB diffuses on ssDNA and transfers rapidly between different segments of ssDNA. SSB interacts with many other proteins involved in DNA metabolism, with 22 such SSB-interacting proteins, or SIPs, defined to date. These interactions chiefly involve the disordered and conserved C-terminal residues of SSB. When not bound to ssDNA, SSB can aggregate to form a phase-separated biomolecular condensate. Current understanding of the properties of SSB and the functional significance of its many intermolecular interactions are summarized in this review.
The E. coli SSB protein: overview
Cells store genetic material in the form of duplex DNA. To access the information sequestered in the interior of the duplex, stretches of the duplex must be unwound to form single-stranded (ss) DNA. Once unwound, ssDNA is rapidly and tightly bound by the ssDNA-binding protein (SSB). The protein functions as a tetramer. Each monomer has a calculated molecular weight of 18,843 from its gene sequence, or 18,710 as it is found in vivo after removal of its N-terminal methionine residue (177 amino acid residues). SSB-ssDNA complexes serve several important functions. These include preventing reannealing of ssDNA to assure polymerases and other proteins have access to template strand base information, protecting ssDNA from degradation, and recruiting a large network of proteins to genomic targets to organize and coordinate cellular genome maintenance (Meyer and Laine Citation1990; Lohman and Ferrari Citation1994; Shereda et al. Citation2008; Antony and Lohman Citation2019).
The essential E. coli SSB has served as a prototype for examining SSB proteins in bacteria for many decades. The protein contains an N-terminal oligosaccharide/oligonucleotide-binding (OB) domain (112 amino acid residues) and an intrinsically disordered C-terminal tail (). The OB domains mediate SSB homo-tetramerization and contain the binding sites for ssDNA. As is described more fully in a later section of this review, E. coli SSB exhibits several DNA binding modes that can accommodate 35–65 bases of ssDNA depending upon a number of factors in the binding reaction. The different binding modes exhibit a wide range of cooperative binding to ssDNA, including negative cooperativity within a tetramer, as well as both nearest neighbor and non-nearest neighbor cooperativity between tetramers on long ssDNA (Lohman and Overman Citation1985; Bujalowski and Lohman Citation1986; Lohman et al. Citation1986; Bujalowski et al. Citation1988; Ferrari et al. Citation1994; Roy et al. Citation2007; Bell et al. Citation2015; Kozlov et al. Citation2015, Citation2017, Citation2019, Citation2022). SSB binding modes are also influenced by binding of SSB to partner proteins, also referred to as SSB interacting proteins (SIPs) (Wessel et al. Citation2013; Bhattacharyya et al. Citation2014; Mills et al. Citation2017; Shinn et al. Citation2019)
Figure 1. E. coli SSB structure and binding modes on ssDNA. (A) A model of an EcSSB tetramer. Opposing subunits of the tetrameric core in cyan (front) and green (back) are shown with intrinsically disordered tails drawn in. (B) A schematic of one EcSSB subunit (177 aa), composed of an N-terminal DNA binding domain, OB fold (112 aa), intrinsically disordered linker, IDL (57 aa), and 8 residue conserved acidic tip (DFDDDIPF). (C) The SSB C-terminus sequence, including the IDL and Ct motif (italic). Positively and negatively charged residues are shown in blue and red, respectively, and glycines are shown in green. (D) A model of SSB-ssDNA complex in the (SSB)65 binding mode, with 65 nts of DNA (orange ribbon) wrapped around the SSB tetramer (C-terminus unresolved). (E) A proposed model for the (SSB)35 binding mode, in which two SSB tetramers interact with 70 nt long DNA (orange tube) using an average of only two subunits per tetramer (C-terminus not shown).
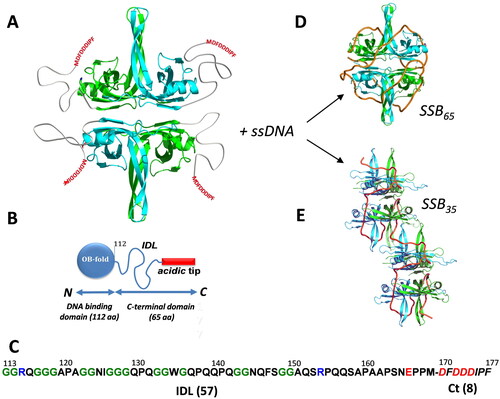
The SSB C-terminal tail is unresolved in full-length SSB crystal structures (Raghunathan et al. Citation1997; Matsumoto et al. Citation2000; Raghunathan et al. Citation2000; Savvides et al. Citation2004). The tail contains two distinct regions: a highly conserved C-terminal tip, referred to as the SSB-Ct (8 amino acid residues), and an intrinsically disordered linker (IDL) that connects the SSB OB domain to the SSB-Ct (57 amino acid residues) (). The SSB-Ct is amphipathic in nature, containing a mix of hydrophobic and charged residues (Asp-Phe-Asp-Asp-Asp-Ile-Pro-Phe) and is sometimes referred to as the ‘acidic tip’ due to the presence of several Asp residues (). The SSB-Ct motif is essential for E. coli survival (Curth et al. Citation1996; Kozlov et al. Citation2015) and is responsible for direct interactions with a network of at least 22 different DNA metabolism proteins (Kelman et al. Citation1998; Genschel et al. Citation2000; Handa P et al. Citation2001; Witte et al. Citation2003; Cadman and McGlynn Citation2004; Han et al. Citation2006; Hobbs et al. Citation2007; Hodskinson et al. Citation2007; Shereda et al. Citation2007; Arad et al. Citation2008; Buss et al. Citation2008; Lu and Keck Citation2008; Suski and Marians Citation2008; Shereda et al. Citation2009; Marceau et al. Citation2011; Page et al. Citation2011; Ryzhikov et al. Citation2011; Cheng et al. Citation2012; Furukohri et al. Citation2012; Naue et al. Citation2013; Wessel et al. Citation2013; Petzold et al. Citation2015; Chen et al. Citation2016; Chang et al. Citation2022). Deletion of the entire motif (SSBΔC8) or just the C-terminal Phe (SSBΔF or SSBΔC1) is lethal, unless cells also express a wild type copy of ssb (Curth et al. Citation1996; Kozlov et al. Citation2015). A proline to serine substitution of the penultimate residue of the SSB-Ct (allele ssb-113) results in a strain that exhibits DNA damage hypersensitivity and temperature-sensitive growth (Greenberg et al. Citation1974; Greenberg and Donch Citation1974; Meyer et al. Citation1982; Wang and Smith Citation1982; Johnson Citation1984). SSB variants have been generated in which either two or four monomers are covalently linked (Antony et al. Citation2013). The variant with two monomers linked forms a stable dimer with four OB folds and two acidic Ct-tails. The variant with four monomers linked forms stable monomer with four OB folds and one Ct-tail. The first of these variants, possessing only two SSB-Ct tails, is functional in vivo. However, the variant with only one SSB-Ct in what is structurally a stable tetramer of OB folds is not (Antony et al. Citation2013).
Unlike the OB and SSB-Ct elements, the function of the SSB IDL is less clear. The IDL is not conserved among bacterial SSBs in sequence or length, although it is generally of low complexity with few charged residues (Kozlov et al. Citation2015; Bonde et al. Citation2023) (). Large insertions or deletions can be made to the IDL (Curth et al. Citation1996; Kozlov et al. Citation2015; Dubiel et al. Citation2020; Bonde et al. Citation2023). Studies in vitro have implicated the IDL in cooperative ssDNA binding (Kozlov et al. Citation2015, Citation2017, Citation2019) and phase separation (Harami et al. Citation2020; Kozlov et al. Citation2022), but the relevance of these functions in vivo is not clear, given the lack of conservation or essentiality of the IDL. A role for the IDL in binding to SIPs has also been proposed, focusing in part on several PXXP motifs that appear in the IDL of the E. coli SSB (Bianco Citation2017, Citation2020, Citation2021; Bianco et al. Citation2017; Bianco and Lyubchenko Citation2017; Nigam et al. Citation2018; Ding et al. Citation2020). However, interactions between SSB variants with large IDL deletions (including 37 of the IDL residues and all of the PXXP motifs) and at least two SIPs are unperturbed in vitro, and such deletions are well tolerated in vivo, suggesting that the IDL is not critical for protein interactions (Shinn et al. Citation2019; Bonde et al. Citation2023). There does appear to be a requirement for a minimum IDL length. A deletion of 47 amino acid residues results in detectable defects in some DNA repair pathways, although addition of a randomized set of 10 amino acid residues relieves the deficiencies (Sandler et al. Citation2024).
E. coli in log phase growth conditions have estimated SSB concentrations of between 500 tetramers per cell in minimal media to over 3,000 tetramers per cell in rich media (Bobst et al. Citation1985; Li et al. Citation2014; Zhao et al. Citation2019; Dubiel et al. Citation2020). The amount of ssDNA in the cell during growth in rich media is estimated to be ∼1.3% of the available DNA (Pham et al. Citation2022). This would represent about 60,000 nucleotides of a 4.6 million base pair E. coli genome, or enough to bind ∼1,000 SSB tetramers. Indeed, mapping studies have shown that SSB binds available ssDNA in E. coli, with much of the SSB associated with the lagging strand template that must be transiently exposed as it is replicated discontinuously (Pham et al. Citation2023).
This review will provide an overview of the historic and recent findings concerning the structure, functions, and mechanisms of E. coli SSB. The review has a particular emphasis on the role of the SSB C-terminus, on which a large number of recent studies have focused. Each of the 22 known SSB-protein interactions is described and the implications of these interactions in the context of DNA replication, repair, and recombination pathways are discussed.
SSB-ssDNA binding modes
It is well established that the E. coli SSB tetramer can bind to ssDNA in multiple modes that differ in their occluded site sizes, the extents of ssDNA wrapping and protein–protein cooperativity. These modes, (SSB)35, (SSB)40, (SSB)56 and (SSB)65, are denoted by a subscript that indicates their occluded site sizes, i.e. the number of nucleotides of ssDNA that are occluded upon binding (Lohman and Overman Citation1985; Bujalowski and Lohman Citation1986; Lohman et al. Citation1986). The different occluded site sizes relate to the relative degree of ssDNA wrapping around the SSB tetramer. The (SSB)35 mode involves the least wrapping, with ssDNA interacting with an average of only two SSB subunits, and the (SSB)65 mode shows the most wrapping, with ssDNA interacting with all four subunits (Lohman et al. Citation1988; Raghunathan et al. Citation2000; Suksombat et al. Citation2015). The relative populations of these modes on ssDNA is dependent upon the protein/DNA ratio (i.e. binding density), solution conditions (including temperature, pH, salt concentration, and cation and anion type and charge (Bujalowski et al. Citation1988)), and SIP binding (Wessel et al. Citation2013; Bhattacharyya et al. Citation2014; Mills et al. Citation2017).
The most well studied binding modes are the (SSB)35 and (SSB)65 modes (). The (SSB)35 mode is favored at low salt concentrations and high SSB/DNA binding densities, whereas the (SSB)65 mode is favored at higher salt concentrations (> 200 mM monovalent salt or >2 mM Mg2+ salts; Bujalowski and Lohman Citation1986). Polyamines, such as spermine and spermidine, also promote the (SSB)65 mode at low mM concentrations (Wei et al. Citation1992). The salt dependence of the binding mode transitions is due partly to a salt-dependent negative cooperativity for ssDNA binding to the SSB tetramer (Lohman and Bujalowski Citation1988; Bujalowski and Lohman Citation1989a, Citation1989b). This results in much higher affinity of ssDNA to the first two SSB subunits and weaker affinity to the third and fourth subunits. The topology of ssDNA wrapping in the (SSB)65 and (SSB)35 modes () has been inferred from crystal structures of SSB bound to two molecules of the oligodeoxynucleotide (dC)35 (Raghunathan et al. Citation2000). ssDNA bound in the (SSB)65 topology follows a path resembling the seams on a baseball or tennis ball. This topology is consistent with single DNA molecule optical tweezer studies that used force applied to the ends of a (dT)70 oligonucleotide to unwrap the DNA from a fully wrapped SSB tetramer in the (SSB)65 mode (Suksombat et al. Citation2015). However, recent experiments suggest that SSB can accommodate other wrapping topologies when bound to non-canonical ssDNA (Kozlov and Lohman Citation2021). This is noteworthy since a structure of the Plasmodium falciparum SSB tetramer bound to two molecules of the oligodeoxynucleotide (dT)35 shows the opposite polarity than observed for the E. coli SSB tetramer (Antony, Weiland, et al. Citation2012). Interestingly, the P. falciparum SSB tetramer binds only in the (SSB)65 and (SSB)56 modes (Antony, Kozlov, et al. Citation2012).
Suksombat et al. (Citation2015) observed even lower binding modes, such as a ∼17 nt state when ssDNA wrapping is inhibited upon applying force on the ssDNA. While these modes are likely unstable in the absence of an applied force, these partially wrapped states are likely on the pathway to formation of the (SSB)35 binding mode.
Functional roles of the SSB binding modes
Although an SSB tetramer is able to bind in multiple binding modes in vitro, it has been difficult to determine the possible functional roles of the modes in vivo. It has been suggested that the (SSB)35 mode would be preferred for use during DNA replication, based on two criteria: 1) its high nearest neighbor cooperativity (Lohman et al. Citation1986; Ferrari et al. Citation1994; Kozlov et al. Citation2019), and 2) its ability to undergo a direct or intersegment transfer between two DNA molecules or two distant segments of the same DNA molecule (Kozlov and Lohman Citation2002). High nearest neighbor cooperativity has been thought to be important during DNA replication since this promotes clustering of SSB proteins along the ssDNA, which would protect the transient ssDNA intermediates from nucleases that might act at ssDNA gaps. A direct transfer mechanism occurs without a free SSB intermediate and involves the formation of an intermediate with two segments (molecules) of ssDNA bound simultaneously to one SSB tetramer (). The ability of the SSB tetramer to undergo a direct transfer between two ssDNA molecules is much more efficient from the (SSB)35 mode since this mode has unoccupied subunits readily available to bind a second ssDNA as an intermediate in the transfer reaction. Hence, it was suggested that SSB tetramers might be recycled from one Okazaki fragment to the next via such a direct transfer mechanism (Kozlov and Lohman Citation2002). Such a mechanism would circumvent the slow dissociation rate of SSB from ssDNA that is too slow to keep pace with DNA replication. In fact, recent single molecule studies have demonstrated that such a direct transfer mechanism does occur to recycle SSB protein during DNA replication, both in vitro and in vivo (Spenkelink et al. Citation2019).
Figure 2. E. coli SSB tetramers are dynamic on ssDNA. (A) EcSSB tetramers can undergo direct transfer (intersegment transfer) of ssDNA molecules through an intermediate where both the donor and recipient ssDNA molecules are bound simultaneously to a single SSB tetramer. (B) SSB tetramers can diffuse along ssDNA via a sliding or reptation mechanism, disrupting DNA secondary structure and facilitating access to ssDNA by other genome maintenance proteins. (C) SSB tetramers can be pushed uni-directionally along ssDNA by an ATP-dependent ssDNA translocase without requiring a specific interaction between SSB and the translocase, allowing reorganization or clearance of SSB on ssDNA.
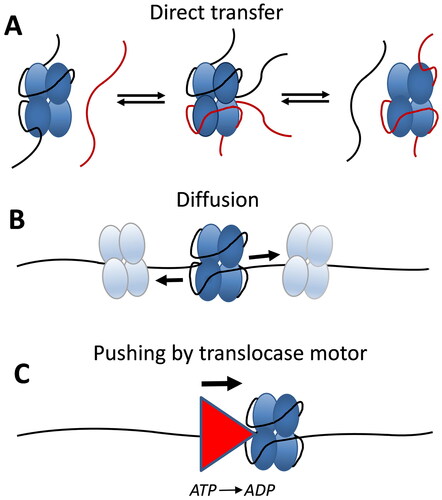
Is the fully wrapped SSB-ssDNA binding mode essential?
If the (SSB)35 mode is preferred during DNA replication, is a fully wrapped (SSB)56 or (SSB)65 binding mode required for E. coli survival? Waldman et al. (Citation2016) attempted to answer this question by designing an SSB tetramer that can only bind in the partially wrapped (SSB)35 mode. Each of the four SSB subunits contains an OB-fold that provides the ssDNA binding site. This study used a dimeric SSB construct containing all four OB-folds, but with two sets of covalently linked subunits (Antony et al. Citation2013). Mutations were then made to eliminate ssDNA binding in two of the four OB-folds thus preventing formation of fully wrapped DNA complexes in vitro. These SSB constructs still retained a wild-type-like, salt-dependent shift in cooperative binding to ssDNA and they complement wild-type SSB in vivo indicating that a fully wrapped binding mode is not essential for function. These results do not preclude some normal function for a fully wrapped mode, but only indicate that E. coli can survive without use of this binding mode.
SSB binding mode dynamics
On polymeric ssDNA, the SSB binding modes can interconvert slowly in a protein concentration dependent equilibrium (Lohman and Overman Citation1985). Roy et al. (Citation2007) used single molecule FRET approaches to examine the salt-dependent dynamics of the SSB concentration dependent interconversion of the (SSB)35 and (SSB)65 binding modes on short ssDNA ((dT)70) that could accommodate one or two SSB tetramers. One interesting finding was that deletion of C-terminal tail shifts the binding mode equilibrium to favor the highly cooperative (SSB)35 mode. Single molecule optical tweezers have been used to study the dynamics of wrapping/unwrapping of ssDNA from a single SSB tetramer on short ssDNA ((dT)70), (Suksombat et al. Citation2015) as well as multiple SSB tetramers on polymeric ssDNA (Naufer et al. Citation2021) demonstrating the kinetics of binding mode interconversion.
SSB-ssDNA binding cooperativity
Negative cooperativity within an SSB tetramer
Individual SSB tetramers display a salt-dependent negative cooperativity (Lohman and Bujalowski Citation1988, Citation1994; Bujalowski and Lohman Citation1989a, Citation1989b; Ferrari et al. Citation1997). This intra-tetramer negative cooperativity results in binding of ssDNA with higher affinity to the first and second SSB subunits, but weaker binding to the third and fourth SSB subunits. The negative cooperativity is most extreme at low [NaCl] concentrations and is weakened, although not eliminated, at higher [NaCl] (Bujalowski and Lohman Citation1989b). The salt dependence of this negative cooperativity partly explains the salt-dependent transition from the (SSB)35 to the (SSB)65 binding mode since ssDNA interacts with only two SSB subunits on average in the (SSB)35 mode, whereas ssDNA interacts with all four SSB subunits in the (SSB)65 mode ().
SSB displays both nearest neighbor and non-nearest neighbor positive cooperativity
SSB tetramers can also display positive cooperativity that promotes protein cluster formation on long, polymeric ssDNA (). The bacteriophage T4 gene 32 protein, the first replicative SSB protein discovered and characterized (Alberts and Frey Citation1970), was shown to bind long polymeric ssDNA with high nearest neighbor positive cooperativity and thus can form protein clusters along ssDNA (Alberts and Frey Citation1970; Kowalczykowski et al. Citation1981). Based on this finding, it has been expected that SSB proteins involved in DNA replication would generally display such high cooperativity. The earliest studies of E. coli SSB protein binding to long ssDNA using electron microscopy showed clear evidence of SSB clustering and high nearest neighbor cooperativity (Sigal et al. Citation1972). In fact, E. coli SSB tetramers can display several different levels of ssDNA binding cooperativity that reflect the properties of the different ssDNA binding modes and thus are dependent on solution conditions, especially salt type and concentration. These different cooperativities range from the high ‘unlimited’ nearest neighbor cooperativity associated with the (SSB)35 mode (Griffith et al. Citation1984; Lohman et al. Citation1986; Ferrari et al. Citation1994), similar to that observed for the T4 gene 32 protein, a ‘limited’ cooperativity resulting in dimers of tetramers (octamers) associated with the (SSB)65 mode in NaCl or KCl (Griffith et al. Citation1984; Lohman et al. Citation1986; Overman et al. Citation1988; Kozlov et al. Citation2019), and a non-nearest neighbor cooperativity associated with the (SSB)65 mode (Bell et al. Citation2015; Kozlov et al. Citation2017; ). Bell et al. (Citation2015) observed a reversible compaction of ssDNA in single DNA molecule experiments beyond what occurs due to formation of the fully wrapped (SSB)65 binding mode. It was later shown that this additional compaction is dependent upon the C-terminal tails and is promoted only in salts containing acetate or glutamate, and not chloride (Kozlov et al. Citation2019). The effects of glutamate are important since glutamate is the major monovalent anion in the E. coli cytoplasm (Leirmo et al. Citation1987; Richey et al. Citation1987).
Figure 3. E. coli SSB cooperativity. SSB tetramers display a range of cooperative interactions, including nearest neighbor (NN) and non-nearest neighbor (NNN) cooperativity.
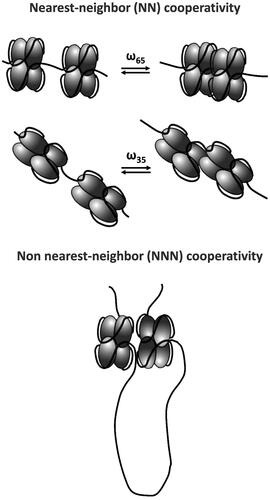
The highly cooperative DNA binding mode observed at high SSB to DNA ratios and low [NaCl] reflects the (SSB)35 mode, but this transitions to a low cooperative DNA binding mode at higher [NaCl], reflecting the (SSB)65 mode (Griffith et al. Citation1984; Lohman et al. Citation1986; Hamon et al. Citation2007). Quantitative estimates of the nearest neighbor cooperativity parameters (ω values) associated with the different binding modes are summarized in . As yet, there is no quantitative model to describe the non-nearest neighbor cooperative binding. All cooperative interactions of E. coli SSB protein are dependent on the intrinsically disordered SSB C-terminus (Kozlov et al. Citation2015, Citation2019). A complete understanding of the structural basis for the different levels of ssDNA binding cooperativity is still lacking, and the cooperativity associated with the (SSB)56 mode has yet to be characterized.
Table 1. Quantitative estimates of the nearest-neighbor cooperativity parameters (ω values) associated with the different binding modes (see ).
Structural features of cooperativity
There is limited understanding of the structural features that are important for cooperative binding of SSB tetramers to ssDNA. As mentioned above, the intrinsically disordered C-terminal tails are necessary for cooperativity (Kozlov et al. Citation2015, Citation2017, Citation2019). This may involve direct interactions between the C-terminal tails, but also between the C-terminal acidic tip and the ssDNA binding sites of adjacent SSB tetramers. The low complexity, high glycine content and low charge composition of the E. coli SSB IDL are all important in promoting cooperative interactions. By contrast, an SSB from Plasmodium falciparum, which has an IDL that is more highly charged with fewer glycines, as well as a chimera in which the E. coli IDL is replaced with the P. falciparum IDL, show neither cooperativity (Kozlov et al. Citation2015, Citation2017) nor the ability to phase separate (Kozlov et al. Citation2022).
Dubiel et al. (Dubiel et al. Citation2019) determined crystal structures of the free and ssDNA bound forms of Bacillus subtilis SSB tetramer, SsbA. A ssDNA bridge was observed between two SsbA tetramers, along with an intermolecular interface, termed the ‘bridge interface’ in which OB domains from adjacent tetramers dock against each other. Mutations made in the equivalent region of E. coli SSB protein showed reduced ssDNA binding cooperativity, and also altered the ssDNA binding mode distributions compared to wild type E. coli SSB. Interestingly, the addition of glutamate did not increase the cooperativity of the E. coli SSB bridge variants.
Curth et al. (Curth et al. Citation1993) have shown that a W54S mutation in E. coli SSB protein favors the (SSB)35 binding mode compared to WT SSB tetramers. However, this effect is not due to an increase in nearest neighbor cooperativity, but rather to an increase in the intra-tetramer negative cooperativity that disfavors the fully wrapped (SSB)56 and (SSB)65 binding modes (Ferrari et al. Citation1997).
SSB dynamics on ssDNA
SSB diffusion on ssDNA
For many years, SSB-ssDNA complexes were viewed as being static, inert complexes. This presented a problem in that the dissociation rate of SSB-ssDNA complexes would be too slow to keep pace with the much faster rates of DNA replication. However, it is now clear that SSB proteins display a range of dynamic interactions on ssDNA. Transient kinetic data suggested that bacteriophage T4 gene 32 protein is able to diffuse along ssDNA (Lohman and Kowalczykowski Citation1981; Lohman Citation1984a, Citation1984b). Based on NMR evidence, Römer et al. (Citation1984) first suggested that E. coli SSB tetramers were mobile along ssDNA and proposed a rolling model for SSB diffusion. Direct evidence and quantitation of SSB tetramer diffusion along ssDNA was obtained using single molecule fluorescence approaches and a one-dimensional diffusion coefficient of 270 nt2/s (37 °C) was estimated (Roy et al. Citation2009). SSB diffusion appears to occur via a sliding or reptation mechanism, rather than a rolling mechanism (Zhou et al. Citation2011). Reptation is proposed to occur when a small loop bulge of ∼3 nucleotides forms via thermal fluctuations in the ssDNA bound to the SSB. This bulge then propagates on the SSB surface via a random walk, i.e. the bulge diffuses. If the bulge is able to diffuse to the other end of the SSB bound DNA, then the SSB protein will have been repositioned on the ssDNA ().
The ability of SSB to diffuse along ssDNA is functionally important in that it provides a mechanism by which it can transiently disrupt secondary structure (e.g. DNA hairpins) and promote RecA filament growth on ssDNA (Roy et al. Citation2009). Single molecule approaches have shown that the major human SSB equivalent, Replication Protein A (RPA), also has the ability to diffuse along ssDNA, with a significantly higher diffusion coefficient (∼5,000 nt2/s at 37 °C) (Nguyen et al. Citation2014). RPA can also transiently disrupt small DNA hairpin structures (Nguyen et al. Citation2014). A reptation mechanism has also been suggested for RPA diffusion (Mishra et al. Citation2020). This ability of SSB proteins to diffuse along ssDNA also allows it to be repositioned along ssDNA, facilitating access to ssDNA by other genome maintenance proteins.
Direct transfer of SSB between DNA molecules or intersegment transfer between distant regions of the same DNA molecule
As discussed above, SSB tetramers are capable of undergoing a direct transfer between two ssDNA molecules, a donor and a recipient, or an intersegment transfer between distant sites within a polymeric ssDNA molecule (Schneider and Wetmur Citation1982; Kozlov and Lohman Citation2002; Lee et al. Citation2014). A direct transfer mechanism occurs without a free SSB protein intermediate and involves the formation of an intermediate in which the donor and recipient ssDNA molecules are bound simultaneously to a single SSB tetramer (). Schneider and Wetmur (Citation1982) first proposed that E. coli SSB protein can undergo a direct transfer from a donor ssDNA to a recipient ssDNA. Kozlov and Lohman (Citation2002) showed that direct transfer of SSB tetramers was much more efficient if the donor ssDNA is bound in the (SSB)35 binding mode since there are two free subunits available for binding of the recipient ssDNA. Single molecule studies of SSB dynamics on polymeric ssDNA detected an apparent diffusion coefficient that was 600 times larger than observed on short ssDNA (Lee et al. Citation2014). It was suggested that this was due to the ability of SSB tetramers to move via intersegment transfer between distant regions of polymeric ssDNA.
Rapid exchange of SSB proteins on ssDNA
Another aspect of SSB-ssDNA dynamics first demonstrated by Kunzelmann et al. (Citation2010) using ensemble stopped-flow methods, is that SSB tetramers bound to ssDNA can undergo an exchange reaction with free SSB tetramers that is much more rapid than SSB-ssDNA dissociation in the absence of free SSB (Kunzelmann et al. Citation2010). This process was later studied using single molecule methods (Naufer et al. Citation2021) and has been shown to occur for RPA as well (Gibb et al. Citation2014). This reaction is analogous to the direct transfer mechanism discussed above except that the SSB tetramers play the role of donor and recipient relative to the ssDNA (). Although this is sometimes described as a mechanism to attain rapid dissociation of SSB from ssDNA, it is more precisely an exchange reaction and the resulting ssDNA product is still saturated with SSB protein. However, this process is likely important in that it allows other proteins to access ssDNA by displacing SSB proteins even when the ssDNA is saturated with SSB protein.
Chemo-mechanical pushing of SSB by an ATP-dependent ssDNA translocase
An interesting aspect of SSB dynamics is the demonstration that E. coli SSB tetramers, as well as eukaryotic RPA proteins, can be ‘pushed’ directionally along ssDNA by ssDNA translocase motor proteins (Sokoloski et al. Citation2016; Mersch et al. Citation2023) (). This pushing reaction is ATP dependent due to the requirement of ATP hydrolysis by the translocase motors. However, no specific interaction between the SSB protein and the translocase is required since pushing was observed with three different translocases, two 3′→ 5′ translocases from E. coli (Rep and UvrD) and one 5′→ 3′ translocase from yeast (Pif1). Such chemo-mechanical pushing by Pif1 of the eukaryotic SSB, RPA, has also been observed (Mersch et al. Citation2023). This pushing occurs by an active mechanism in that the rate of pushing is determined by the translocation rate of the motor enzyme (Sokoloski et al. Citation2016; Mersch et al. Citation2023). The ability of a translocase motor to push SSB proteins chemo-mechanically along ssDNA provides a potential mechanism for reorganization and clearance of tightly bound SSBs from ssDNA. In fact, another ssDNA translocase, human HelB, is able to displace multiple RPA proteins from ssDNA using its translocase activity (Hormeno et al. Citation2022).
Function and effects of the SSB C-terminus
Effect of the C-terminal tails and SIPs on the SSB binding modes
The C-terminal tails of the SSB protein are the primary sites of interactions with SSB interacting proteins (SIPs) (Shereda et al. Citation2008; Kozlov, Jezewska, et al. Citation2010; Shinn et al. Citation2019; Bonde et al. Citation2023), but they also play a role in regulating ssDNA binding affinity (Kozlov, Cox, Lohman Citation2010), binding cooperativity (Kozlov et al. Citation2015, Citation2019) and the SSB binding mode preference (Roy et al. Citation2007). These C-terminal tails are highly flexible, intrinsically disordered, and relatively compact (Savvides et al. Citation2004; Kozlov et al. Citation2015; Green et al. Citation2016). Deletion of the acidic C-terminal tails on the SSB tetramer shifts the binding mode preference to favor the (SSB)35 mode (Roy et al. Citation2007). This is due to an increase in the negative cooperativity for ssDNA binding to the third and fourth SSB subunits which results in stabilization of the (SSB)35 mode (Kozlov, Cox, Lohman Citation2010). Interestingly, the binding of SIPs, PriA and PriC (Wessel et al. Citation2013; Bhattacharyya et al. Citation2014) and RecQ (Mills et al. Citation2017), to the acidic tips of the C-terminal tails also shifts the binding mode preference to favor the (SSB)35 mode. This may result from a sequestering of the acidic tip by these SIPs. A recent study also suggests that sites of DNA damage can alter the SSB binding mode preference (Morse et al. Citation2023).
Are all four C-terminal tails required for SSB function?
Each wild type SSB tetramer has four flexible C-terminal tails. However, some similar SSB proteins from other organisms, such a Deinococcus radiodurans, are dimeric. The dimer contains four OB-folds (Bernstein et al. Citation2004) and displays a salt-dependent DNA binding mode transition (George et al. Citation2012), similar to that observed for E. coli SSB tetramers. This raises the question of how many C-terminal tails are needed for SSB function in E. coli. Antony et al. (Citation2013) addressed this question by designing SSB variants with either two or all four subunits covalently linked. Each variant still retains all four OB-folds and ssDNA binding affinity. A dimer containing monomers with two covalently linked SSB subunits contains only two C-terminal tails and a ‘monomer’ with all four subunits covalently linked possesses only one C-terminal tail. E. coli that express the SSB variant with only two tails can survive, recover faster from exposure to DNA damaging agents, but accumulate more mutations. However, expression of a single-tailed SSB is dominant lethal. A single-tailed SSB shows defects in the coupling of leading and lagging strand DNA replication and does not support replication restart in vitro, providing a plausible explanation for the lethality observed in vivo. These results also suggest that a single wild type SSB tetramer must interact simultaneously with multiple protein partners (SIPs) during at least some essential function in genome maintenance.
The network of SSB-interacting proteins
SSB has emerged as a central hub in intricate protein-protein networks, engaging in direct interactions with an array of partner proteins mediated by the SSB-Ct. These interactions are pivotal for coordinating a diverse repertoire of cellular functions that proceed through ssDNA intermediates, ranging from DNA replication to repair and recombination processes.
The crystal structures of seven different SSB-Ct-bound SIPs have been reported: Exonuclease I (Lu and Keck Citation2008), PriA (Bhattacharyya et al. Citation2014), RecO (Ryzhikov et al. Citation2011), uracil DNA glycosylase (unpublished, PDB ID: 3UF7), ribonuclease HI (Petzold et al. Citation2015), DnaG primase (Lo Citation2012, PDB ID: 6CBS), and the chi subunit of the replicative polymerase holoenzyme (Marceau et al. Citation2011). The ribonuclease HI/SSB-Ct interface is shown in as an example. The structural analysis to date has highlighted the fact that SIPs have evolved SSB-Ct binding sites on different domains/folds – i.e. there is no single ‘SSB-binding’ domain. Instead, it appears that multiple SIPs that require access to SSB/ssDNA substrates have adapted similar strategies for docking onto SSB by evolving SSB-Ct binding pockets as needed, serving as an intriguing example of convergent evolution.
Figure 4. SSB/SIP binding interfaces revealed by structural studies. (A) Ribonuclease H/SSB-Ct interface. SSB-Ct residues are shown as sticks. Ribonuclease H residues shown in sticks form the interaction surface for binding SSB. (B) Notable interactions between SIPs and SSB-Ct are highlighted. SIP residues that interact with specific elements of the SSB-Ct are noted; these are an aggregate of all interactions that have been observed in SIP/SSB-Ct crystal structures determined to date.
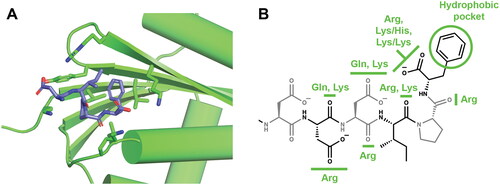
Comparison of SIP/SSB-Ct complex structures has revealed common features that support interaction with the SSB-Ct (). The first is a ‘basic lip’ element that interacts with the alpha-carboxyl group of the C-terminal end of SSB. Basic lips can be comprised of 1-2 Arg side chains or combinations of Lys/His or two Lys side chains, and sequence changes to the basic lip element generally strongly destabilize SIP/SSB interaction in vitro and/or in vivo. The second element is a hydrophobic pocket that accommodates the side chain of the C-terminal-most Phe residue. The final element is a ‘basic ridge’ that is poised to interact with the side chains of Asp residues or carbonyl groups within the SSB-Ct. Direct interactions between the SSB-Ct Asp side chains and SIPs are rarely observed in crystal structures likely due to high salt concentrations that often are required for crystallization.
The following sections focus on the structural, mechanistic, and functional underpinnings that underlie the molecular associations between SSB and SIPs. Each interaction is summarized in the context of its broader implications in cellular physiology and genome maintenance. We aim to provide a comprehensive perspective on how SSB/SIP interactions collectively shape the cellular landscape and to identify commonalities, as well as unique characteristics, that define each of the 22 SSB/protein interactions (listed in ).
Table 2. SSB interacting proteins and the effects of each interaction.
Replication restart proteins: PriA, PriB, and PriC
PriA
PriA (replication factor Y, protein n′; 81.7 kDa) is a 3′→ 5′ ssDNA translocase and helicase that belongs to the superfamily 2 (SF2) family of helicases (Lasken and Kornberg Citation1988). PriA was discovered with several other primosomal proteins (PriB, DnaT, PriC, DnaB, and DnaC) for its requirement in the replication of the bacteriophage φX174 genome (Wickner and Hurwitz Citation1975; Shlomai and Kornberg Citation1980a, Citation1980b). The primary function in PriA appears to be in the initiation of origin-independent replication restart at stalled replication forks, where PriA is proposed to recognize stalled forks in a structure specific manner (reviewed in Windgassen, Leroux, et al. Citation2018). Multiple replication restart pathways have been defined in E. coli, two of which involve PriA: the PriA/PriB/DnaT and PriA/PriC/DnaT pathways (Windgassen, Wessel, et al. Citation2018). The PriA/PriB/DnaT pathway is the most commonly used replication restart pathway in E. coli, and PriA mutations often produce severe phenotypes, including SOS DNA damage response induction, slow growth in rich media, and sensitivity to DNA damaging agents (Lee and Kornberg Citation1991; Nurse et al. Citation1991; Sandler Citation1996; McCool and Sandler Citation2001; Sandler et al. Citation2001; Michel and Sandler Citation2017). Deletion of the priA gene funnels most replication restart into a pathway entirely reliant on PriC (Michel and Sandler Citation2017).
PriA interacts with SSB via the SSB-Ct. Surface plasmon resonance (SPR) binding experiments with PriA and a peptide containing the 15 C-terminal residues of SSB reveal a dissociation constant (Kd) of 2.4 ± 1.3 μΜ, and this interaction is weakened when the peptide contains the ssb113 mutation (Kd = 6.9 ± 2 μΜ) (Cadman and McGlynn Citation2004). Isothermal titration calorimetry (ITC) binding experiments have been performed with PriA and SSB-Ct peptides, full length SSB, and DNA-bound PriA/SSB in a variety of buffers (Kozlov, Jezewska, et al. Citation2010). These experiments reveal dissociation constants ranging from high nanomolar (at 20 mM NaCl) to low micromolar (in buffers containing 200 mM NaCl). Pre-binding PriA to ssDNA does not affect the interaction between PriA and an SSB-Ct peptide, but PriA has a higher affinity for DNA-bound SSB (Kozlov, Jezewska, et al. Citation2010). No binding was detected between PriA and C-terminal SSB peptides containing the SSB113 sequence change or a peptide synthesized in a sequence-randomized order (Kozlov, Jezewska, et al. Citation2010). The SSB-Ct-bound crystal structure of PriA from Klebsiella pneumoniae, which shares 88% sequence identity with and complements the loss of E. coli PriA, has been solved, revealing Arg697 to be a key residue of the SSB binding pocket on PriA (Bhattacharyya et al. Citation2014).
Helicase activity of PriA is coupled to ATP hydrolysis, and both the ATPase activity and branched DNA unwinding activity of PriA is stimulated by SSB (Cadman and McGlynn Citation2004; Tan and Bianco Citation2021). Stimulation of PriA by SSB is species specific, and this may be dependent on the glycine composition of SSB IDLs (Huang and Huang Citation2018). SSBΔC10 (removing the C-terminal 10 residues of SSB) and SSB113 variants inhibit PriA fork unwinding (Cadman and McGlynn Citation2004). SSB allows PriA to unwind dsDNA substrates longer than 40 bps (Lasken and Kornberg Citation1988) and it plays a role in assisting PriA helicase domain engagement of the lagging strand in replication fork structures (Windgassen, Leroux, et al. Citation2018). While an interaction with SSB affects PriA activity, PriA also impacts SSB-DNA interactions by stabilizing the SSB35 DNA binding mode over the SSB65 mode (Bhattacharyya et al. Citation2014). PriA R697A is unable to modulate SSB DNA binding modes (Bhattacharyya et al. Citation2014).
Loss of the PriA/SSB interaction affects PriA activity in cells. However, PriA SSB binding pocket mutants, priA341 (R697A), priA344 (R697E), and priA345 (Q701E), do not exhibit any phenotypes by themselves (Klimova and Sandler Citation2020). When the binding pocket mutations are combined with a ΔpriB mutation, cells have defects in nucleoid partitioning and show an increase in SOS induction and cell length, which can be partially suppressed by overexpression of SSB (Klimova and Sandler Citation2020).
PriB
PriB is an ∼11 kDa dimeric ssDNA binding protein that shares structural and sequence similarity to SSB (Lopper et al. Citation2004) and may have arisen from an SSB gene duplication event (Ponomarev et al. Citation2003). PriB is involved in the PriA/PriB/DnaT replication restart pathway and binds to both DNA and PriA, assisting with the formation of a tertiary complex with DnaT and subsequent loading of DnaB, the replicative helicase in E. coli (Heller and Marians Citation2005; Lopper et al. Citation2007). Yeast two-hybrid (Y2H) studies have revealed that PriB interacts with PriA, DnaT, itself, and SSB (Huang et al. Citation2013). PriB residues K82, K84, and K89 in the L45 loop of the protein may be involved in interaction with SSB (Huang et al. Citation2013).
PriC
PriC (∼20 kDa) is involved in the PriA/PriC/DnaT and PriC/Rep replication restart pathways. It was first identified as a potential SIP in an SSB tandem affinity purification (TAP) screen and confirmed to interact with SSB through yeast two-hybrid and biochemical experiments (Wessel et al. Citation2013). Yeast two-hybrid experiments reveal that inclusion of either SSB113 or PriC variants that neutralize residues Arg121 and Arg155 disrupt the PriC/SSB complex (Wessel et al. Citation2013). Residues Arg129, Phe118, and Tyr152 have also been proposed to help form the SSB binding pocket on PriC (Aramaki et al. Citation2015). PriC binds to an SSB-Ct peptide with a Kd of 3.7 ± 0.7 μM and a 1:1 monomeric stoichiometry, as measured by ITC (Wessel et al. Citation2013). Shinn et al. also measured this by ITC under different solution conditions, yielding Kd (pH 8, 10 mM NaCl) (Shinn et al. Citation2019). As with the yeast two-hybrid results, inclusion of SSB113 or SSBΔF peptides or PriC R121A and PriC R155A variants resulted in a loss of PriC/SSB-Ct complex formation (Wessel et al. Citation2013).
The PriC/SSB interaction has important biological implications on replication restart. The interaction is required for PriC-mediated loading of the DnaB replicative helicase onto DNA in vitro, and the inclusion of SSB113, SSBΔF, PriC R121A, or PriC R155A variants blocks DnaB loading (Wessel et al. Citation2013). Similar to the PriA/SSB interaction, PriC modulates SSB-ssDNA interactions and stabilizes the SSB35 mode over the SSB65 DNA binding mode, potentially making ssDNA available for DnaB loading (Wessel et al. Citation2013). Cells expressing the PriC R121A and PriC R155A variants do not exhibit phenotypes on their own, but this is due to the primary replication restart pathway (PriA/PriB/DnaT) remaining intact. Combining the priC mutations with a deletion of priB results in cells with severely reduced viability that readily acquire suppressor mutations (Wessel et al. Citation2013; Klimova and Sandler Citation2020). The cells are highly filamented and exhibit high levels of SOS induction (Klimova and Sandler Citation2020).
PriC can interact with Rep protein to activate the helicase activity of the Rep monomer. In the absence of PriC, a Rep monomer is a rapid ssDNA translocase, but has no helicase activity (Brendza et al. Citation2005). Rep helicase activity requires Rep dimerization (Chao and Lohman Citation1990; Cheng et al. Citation2001) or deletion of an auto-inhibitory domain (Cheng et al. Citation2002; Brendza et al. Citation2005). PriC can self-assemble to form dimers and tetramers and the PriC dimer can activate the helicase activity of the Rep monomer (Nguyen et al. Citation2021). However, interaction of PriC with the C-terminal acidic tip of SSB eliminates the ability of PriC to activate Rep by stabilizing the PriC monomer (Nguyen et al. Citation2021).
Interactions with the replisome: DNA polymerase III (χ) and DnaG
DNA polymerase III (χ)
Interaction between the χ subunit (15 kDa) of the DNA polymerase III holoenzyme (Pol III HE) processivity clamp loader and SSB has been well characterized. The χ/SSB interaction is important for several aspects of DNA replication, including the coupling of leading and lagging strand DNA synthesis, loading of the β-clamp onto DNA by the clamp loader, and replisomal stability (Glover and McHenry Citation1998; Kelman et al. Citation1998; Marceau et al. Citation2011; Newcomb et al. Citation2022). The χψ subunits of the clamp loader complex co-elute with SSB in gel filtration experiments, and SPR experiments revealed a 2.7 μM Kd for χψ/SSB (Glover and McHenry Citation1998). Omitting the χ subunit results in loss of interaction between the Pol III HE and SSB, attributing the interaction between the clamp loader complex and SSB to the χ subunit (Kelman et al. Citation1998). Indeed, the χ subunit alone co-migrates with SSB in gel filtration experiments (Kelman et al. Citation1998).
Removal of the χ subunit from Pol III HE also destabilizes DNA synthesis in moderate to high salt conditions (Glover and McHenry Citation1998; Kelman et al. Citation1998), and this effect is attributed to loss of the interaction with SSB, as substitution of SSB with the SSB113 variant produces the same destabilization observed with omission of χ. The χ/SSB interaction enhances SSB/ssDNA binding as seen by a lowering of the melting temperature of dsDNA (Witte et al. Citation2003). The inverse is also true; the interaction enhances clamp loader–DNA interactions by increasing the lifetime of the clamp loader–clamp complex on DNA (Newcomb et al. Citation2022). This allows the clamp loader to remodel SSB-DNA interactions such that the β-clamp can be loaded onto DNA (Newcomb et al. Citation2022). This function is blocked by the SSBΔF variant.
The χ subunit alone binds SSB with a Kd of 0.33 μM (Kelman et al. Citation1998). This Kd increases to 16.8 μM when binding to SSB113 is measured (Kelman et al. Citation1998), indicating the specificity of the interaction for an intact SSB-Ct. Interestingly, the interaction between χ and SSB113 is temperature sensitive. Binding is readily measured at 24 °C–25 °C by SPR and ITC but not detected by either technique at 37 °C or 40 °C (Yuzhakov et al. Citation1999; Marceau et al. Citation2011). χ binding to an SSB-Ct peptide comprising the 15 C-terminal residues of SSB was also observed (Kelman et al. Citation1998). Binding between wild type and variant χ and SSB protein and peptides has also been extensively measured by ITC (Kozlov, Jezewska, et al. Citation2010; Marceau et al. Citation2011) and analytical ultracentrifugation (Naue et al. Citation2011), revealing Kd values in the 0.5 μM to 10 μM range for wild type sequences. Residues that comprise the SSB binding pocket on χ were identified through a combination of docking (Fedorov et al. Citation2006) and crosslinking experiments (Naue et al. Citation2011). These studies revealed roles for χ residues V117, Y131, T143 in forming the hydrophobic pocket that accommodates the C-terminal Phe side chain of the SSB-Ct and for charged residues R128, K132, Arg135 in electrostatic interactions with the SSB-Ct. The binding pocket was further confirmed by an X-ray crystal structure of χψ bound to the last 4 residues of the SSB-Ct (Marceau et al. Citation2011).
Incorporation of SSB binding pocket mutations in χ (holC) on the chromosome leads to several cellular defects. These defects include temperature sensitivity, reduced DNA replication rates, SOS induction, chromosome partitioning defects, and cell filamentation (Marceau et al. Citation2011). These results highlight the importance of the χ/SSB interaction during normal DNA replication processes.
DnaG
Pol III HE cannot initiate DNA chain synthesis de novo. Rather, DnaG primase (60 kDa) is needed for priming Pol III HE DNA elongation by generating RNA primers on the DNA template (Rowen and Kornberg Citation1978). This process occurs repeatedly during DNA replication, as lagging strand synthesis requires an RNA primer for each Okazaki fragment. DnaG, like the χ subunit of Pol III HE, interacts with SSB, and this interaction is instrumental in coordinating the transition from primase activity by DnaG to DNA elongation by Pol III (Yuzhakov et al. Citation1999). DnaG and χ compete for SSB binding, as they both bind to the SSB-Ct (Yuzhakov et al. Citation1999; Naue et al. Citation2013). ITC experiments in different buffer conditions have measured the DnaG/SSB Kd to be between 4 and 14 μM (Naue et al. Citation2013). The SSB binding site on DnaG has been mapped to the C-terminal helicase binding domain of the protein. The same domain in DnaG binds to the replicative helicase (DnaB); however, the SSB-Ct and DnaB binding surfaces differ (Naue et al. Citation2013). Nonetheless it appers that DnaB and SSB cannot bind simultaneously to DnaG (Lo Citation2012 and Ute Curth, personal communication). Cross-linking, NMR experiments have identified key residues within the SSB-Ct binding pocket, which include K447, K518, and R452 (Naue et al. Citation2013). This binding site was further confirmed by a crystal structure of a DnaG-SSB-Ct chimeric protein (Lo Citation2012, PDB ID: 6CBS).
Translesion synthesis (TLS) polymerases: DNA polymerases II, IV, and V
DNA polymerase II (pol II)
Pol II (89.9 kDa) is one of three TLS polymerases in E. coli that allows lesion bypass during DNA replication. All three TLS polymerases are upregulated during the SOS response and have known interactions with SSB. Pol II contains both polymerase and exonuclease activities (Kornberg and Gefter Citation1971), and it was the first protein reported to interact with SSB (Sigal et al. Citation1972; Molineux and Gefter Citation1974). SSB stimulates Pol II DNA synthesis on templates with long regions of ssDNA and is required, along with the β-clamp and γ complex, for processivity and bypass of abasic sites (Sigal et al. Citation1972; Weiner et al. Citation1975; Bonner et al. Citation1992). Likewise, the presence of SSB inhibits nuclease activity of DNA polymerase I and Pol III HE but has a stimulatory effect on Pol II nuclease at saturating concentrations (Molineux and Gefter Citation1974). The Pol II/SSB interaction also allows Pol II binding to internal ssDNA regions (Molineux and Gefter Citation1974).
DNA polymerase IV (pol IV)
The most abundant TLS polymerase, Pol IV (encoded by dinB; 39.5 kDa), comprises a catalytic domain and C-terminal ‘little finger’ domain that interacts with the β-clamp (Bunting et al. Citation2003). An interaction between Pol IV and SSB was uncovered through pull-down experiments (Furukohri et al. Citation2012). This interaction is specific for the SSB-Ct motif, as SSBΔC8 does not copurify with Pol IV in pull-down assays (Furukohri et al. Citation2012). Full-length SSB stimulates Pol IV DNA synthesis activity, while SSBΔC8-coated ssDNA inhibits Pol IV activity and processivity. Similarly, Pol IV TLS is stimulated by interaction with SSB, evidenced by reduced TLS rates when variant SSB (SSBΔF), no SSB, or an interaction-deficient Pol IV is used in the reaction (Chang et al. Citation2022). The SSB-Ct peptide alone does not stimulate Pol IV activity, and addition of SSB-Ct peptide inhibits Pol IV primer elongation rates on SSB-coated DNA as the peptide competes with full-length SSB for Pol IV binding (Furukohri et al. Citation2012).
Pol IV binding to ssDNA-bound SSB has been measured in fluorescence polarization (FP) assays, revealing a Kd of 2 ± 0.4 μΜ (Chang et al. Citation2022). A Pol IV variant (T120P) that exhibits a loss of interaction with SSB in vitro was identified from a collection of Pol IV point mutations that suppress overexpression toxicity (Chang et al. Citation2022). Pol IV T120P exhibits reduced TLS activity, and cells expressing Pol IV T120P are induced for SOS and sensitized to DNA damaging agents nitrofurazone (NFZ) and methylmethanesulfonate (MMS) (Chang et al. Citation2022). After MMS treatment, a Pol IV fluorescent fusion (Pol IV-PAmCherry) is enriched eight-fold at replisomes (marked by signal from a C-terminal fluorescent fusion, SSB-mYPet), while the T120P variant is only enriched two-fold (Chang et al. Citation2022). This enrichment is specific to the Pol IV/SSB interaction and not a consequence of other Pol IV/protein interactions. However, the Pol IV T120P variant also exhibits reduced binding to the Pol III HE core subunits which negatively impacts the ability of Pol IV to exchange with Pol III HE (Scotland et al. Citation2015), and it is not known whether defects in SSB binding and/or Pol III HE binding lead to the NFZ and MMS sensitivity in Pol IV T120P expressing cells (Scotland et al. Citation2022). Enrichment of Pol IV at replication forks is not specific to conditions where the induced DNA damaged results in lesions Pol IV is known to bypass; enrichment is also observed after UV exposure and hydroxyurea treatment (which does not sensitize Pol IV mutants) (Thrall et al. Citation2022). This indicates Pol IV, as well as other repair factors, are recruited to the stalled folks in a general, not specific, manner (Thrall et al. Citation2022).
DNA polymerase V (Pol V)
Pol V TLS polymerase (70 kDa) is encoded by the umuC and umuD genes. During TLS, the RecA recombinase promotes autocleavage of UmuD to UmuD′ (Burckhardt et al. Citation1988; McDonald et al. Citation1998; Nohmi et al. Citation1988; Shinagawa et al. Citation1988). Two UmuD′ subunits come together with one UmuC to form Pol V. Pol V-mediated TLS relies on several SSB functions. The first is a requirement for the tetrameric form of SSB. The second requirement is for SSB to increase the accessibility of the 3′ primer terminus. However, this second requirement is not specific to a Pol V/SSB interaction because T4 gp32 SSB also allows primer extension up to an abasic site (Arad et al. Citation2008). Finally, Pol V directly interacts with SSB (Sarov-Blat and Livneh Citation1998) via the SSB-Ct, and this direct interaction appears to be responsible for stimulating TLS activity, as Pol V TLS activity is reduced in the presence of SSB113 (Arad et al. Citation2008).
Interactions with DNA recombination factors: RecQ, RecJ, RecO, RadD, RecG, and RarA
Several proteins involved in both the initiation of recombination, as well as during late-stage recombination, are SSB protein partners. Whereas the RecBCD nuclease-helicase (initiates double-strand DNA break repair in E. coli) does not interact with SSB, many key proteins in the RecFOR pathway (repairs ssDNA gaps) form direct interactions with SSB. The RecF, RecO, and RecR proteins are primarily involved in the identification and recombinational DNA repair of lesion-containing post-replication gaps (Cox et al. Citation2023). Given the substrate for this repair process is an SSB-coated single strand gap, it is perhaps unsurprising that proteins involved in the process that interact with SSB. Those identified to date are RecQ (Harmon and Kowalczykowski Citation2001; Shereda et al. Citation2007), RecJ (Costes et al. Citation2010; Cheng et al. Citation2016), RecO (Hobbs et al. Citation2007), RadD (Chen et al. Citation2016), RecG (Buss et al. Citation2008; Bonde et al. Citation2023), and RarA (previously MgsA) (Page Citation2012).
RecQ
RecQ (68.4 kDa) is a 3′→ 5′ helicase. In vitro, RecQ can function in concert with RecJ at DNA ends by unwinding DNA, a process that may be important in the context of double strand DNA break repair (Morimatsu and Kowalczykowski Citation2014). To initiate the repair of double strand DNA breaks by the RecFOR or RecBCD recombination pathways, the DNA strand ends must first be processed and resected. In E. coli, double-strand DNA end processing is primarily performed by the RecBCD nuclease-helicase (Amundsen et al. Citation1990; Kowalczykowski and Roman Citation1990; Anderson and Kowalczykowski Citation1997; Arnold and Kowalczykowski Citation1999; Singleton et al. Citation2004; Dillingham and Kowalczykowski Citation2008). In the absence of the RecBCD complex, the RecQ/RecJ system within the RecFOR pathway can provide a less effective backup (Takahashi et al. Citation1993; Cromie and Leach Citation2001; Ivancic-Bace et al. Citation2005). RecQ and RecJ can coordinate their activities in vitro to process a variety of double-strand DNA ends, including blunt ends and 5′ or 3′ overhangs (Handa N et al. Citation2009; Morimatsu and Kowalczykowski Citation2014). However, in vivo, only RecJ (a 5′→ 3′ ssDNA nuclease) appears to be needed, with RecQ or other helicases presumably opening the duplex to provide RecJ a ssDNA substrate (Ivancic-Bace et al. Citation2005).
As indicated, RecQ function during double strand break repair is secondary to the RecBCD helicase/nuclease. Other cellular functions of RecQ, including the resolution or reversal of joint molecules formed during post-replication gap repair and the disruption or suppression of illegitimate recombination events are probably the primary roles of RecQ in wild type cells (Harmon and Kowalczykowski Citation1998; Harami et al. Citation2017; Jain, Wood, Cox Citation2021). In concert with topoisomerase III, RecQ resolves converging replication forks (Suski and Marians Citation2008) and is involved in the supercoiling and catenation of DNA (Harmon et al. Citation1999, Citation2003). In the context of post-replication gap repair, RecQ and RecJ do not collaborate but instead act at very different stages of the process. Strains lacking the functions of the RarA, RuvB, and RecQ enzymes, thus blocking all paths to resolve RecA-generated joint molecules behind the fork, are inviable (Jain, Wood, Cox Citation2021). This outcome is suppressed by deletion of recF, recO, or recJ genes (Jain, Wood, Cox Citation2021), indicating that RecJ is involved in the early establishment of RecA filaments in the gap, as are the RecFOR proteins (Jain, Wood, Cox Citation2021).
RecQ helicase activity is stimulated by SSB in a variety of contexts (Umezu and Nakayama Citation1993; Shereda et al. Citation2007; Suski and Marians Citation2008; Mills et al. Citation2017; Bagchi et al. Citation2018), and interaction with SSB induces a faster RecQ DNA unwinding mode (Bagchi et al. Citation2018). This effect is specific, as SSBΔC8, T4 gp32, and S. cerevisiae RPA all inhibit RecQ activity (Shereda et al. Citation2007). The RecQ/SSB interaction also remodels SSB/ssDNA interactions, allowing RecQ to gain access to ssDNA (Mills et al. Citation2017).
RecQ interacts with SSB through the SSB-Ct (Shereda et al. Citation2007), and the SSB-Ct is important for colocalizing RecQ to replisomes in Bacillus subtilis (Lecointe et al. Citation2007). ITC binding experiments measure a Kd of ∼6 μM for the interaction between RecQ and full-length SSB (Shereda et al. Citation2007). An SSB113 peptide variant has greatly reduced binding to RecQ, and a peptide with a mixed SSB-Ct sequence does not result in any detectable binding (Shereda et al. Citation2007), providing further support for the specificity of the interaction. The SSB binding site on RecQ has been mapped to the winged-helix (WH) domain of the protein by NMR (Shereda et al. Citation2009). Sequence changes to RecQ WH residues R425, R499, or R503 result in significantly reduced interaction with SSB. SSB stimulation of helicase activity of these variants is also reduced (Shereda et al. Citation2009). Interestingly, SSB can still stimulate a RecQ variant that is missing the WH domain, suggesting RecQ and SSB may have an additional interaction site (Bagchi et al. Citation2018).
RecJ
RecJ (63.4 kDa) is responsible for resecting 5′ ssDNA ends in preparation for recombination (Han et al. Citation2006). RecJ exonuclease activity is stimulated on ssDNA pre-bound by SSB (Han et al. Citation2006). This stimulation likely occurs via a direct interaction between RecJ and SSB because T4 gp32 is unable to produce the same stimulatory effect, suggesting that DNA binding activity/the melting of DNA secondary structures in not sufficient for stimulation (Han et al. Citation2006). RecJ, SSB, and ssDNA form a ternary complex, and SSB enhances RecJ interactions with ssDNA (Costes et al. Citation2010; Cheng et al. Citation2016). The RecJ/SSB binding interface has not been uncovered, but the interaction is also observed in TAP assays in E. coli (Shereda et al. Citation2007) and B. subtilis (Costes et al. Citation2010). RecJ/SSB interaction is required for RecJ degradation of SSB-bound ssDNA (Han et al. Citation2006). Extensive resection of DNA ends by RecQ and RecJ in the context of double strand DNA break repair is blocked by SSBΔC8 (Morimatsu and Kowalczykowski Citation2014). In post-replication gap repair, RecJ and RecQ have very different roles. RecJ is required (without RecQ) for initiation steps (presumably to enlarge the gap), whereas RecQ plays a role in some pathways for joint molecule resolution later in the process (Ivancic-Bace et al. Citation2005; Jain, Wood, Cox Citation2021).
RecO
Lesion-containing post-replication gaps are identified by RecF (40.5 kDa) and RecR (22 kDa), most likely via an interaction with the DnaN β-clamp (Henry, Kaur, et al. Citation2023; Henry, Mbele, Cox Citation2023). RecO (27.3 kDa) and RecR are required to load RecA onto SSB-coated ssDNA, which otherwise inhibits RecA access to the DNA (Umezu et al. Citation1993; Umezu and Kolodner Citation1994). Among these proteins, only RecO interacts with SSB, forming a complex that requires the SSB-Ct (Umezu and Kolodner Citation1994; Hobbs et al. Citation2007). RecO was first reported to directly interact with SSB in SPR and sedimentation experiments (Umezu and Kolodner Citation1994). The interaction is abolished in vitro when an SSB variant lacking 8 C-terminal amino acid residues is used, implicating the C-terminus in the interaction (Hobbs et al. Citation2007). An X-ray crystal structure of RecO bound to an SSB-Ct peptide has been determined, revealing a binding pocket on RecO framed by residues F228, R210, and R132 (Ryzhikov et al. Citation2011). RecO binds to the SSB-Ct peptide with a Kd of 0.06 ± 0.01 μM in FP experiments (Ryzhikov et al. Citation2011). The RecO/SSB interaction has also been extensively measured using ITC, where binding between RecO and SSB C-terminal peptides of varying lengths, along with full-length SSB, yield Kd values in the 0.02 − 0.8 μM range (Shinn et al. Citation2019, Citation2021, Citation2023). These experiments indicate that RecO is unusual among SIPs in that, in addition to interacting with the SSB-Ct, RecO also interacts with the OB domain (Shinn et al. Citation2019). The structural basis of the RecO/SSB OB domain interface remains to be determined.
RecO is able to efficiently reanneal complementary ssDNA strands that are both individually bound by SSB (but not RPA or T4 gp32), suggesting that RecO modulates SSB-DNA interactions by interacting with SSB (Kantake et al. Citation2002). Inclusion of SSBΔC8 in the reaction inhibits RecO-mediated DNA reannealing (Ryzhikov et al. Citation2011). Interaction with the SSB-Ct with RecO prevents RecO-ssDNA aggregation (Shinn et al. Citation2023). RecOR complexes can also bind ssDNA via RecO, and aggregation is suppressed even in the absence of the SSB-Ct peptide, demonstrating an allosteric effect of RecR on RecO binding to ssDNA. Under conditions where RecO binds ssDNA but does not form aggregates, SSB-Ct binding enhances the affinity of RecO for ssDNA. For RecOR complexes bound to ssDNA, a shift in RecOR complex equilibrium toward a RecR4O complex upon binding SSB-Ct is also observed (Shinn et al. Citation2023).
Once RecA is loaded onto gapped DNA and initiates strand invasion, several proteins have been implicated in accelerating or antagonizing strand exchange and in the processing of the branched recombination intermediate molecules that are formed. Some of these proteins also interact with SSB, including RadD, RecG, and RarA.
RadD
RadD (66.4 kDa) is a putative SF2 helicase that stimulates RecA-mediated strand exchange (Bonde et al. Citation2022). RadD interaction with SSB was initially identified in a TAP assay and confirmed by Y2H and ammonium sulfate co-precipitation (Chen et al. Citation2016). RadD co-precipitation requires the SSB-Ct (Chen et al. Citation2016). Similarly, full-length SSB and SSB-Ct peptide stimulate RadD DNA-independent ATP hydrolysis activity by lowering the KM and increasing the Vmax for the reaction, while SSBΔC8, SSBΔF, or mixed sequenced peptides do not stimulate RadD ATPase (Chen et al. Citation2016). RadD binds to SSB-Ct peptide with a Kd of 5.4 ± 1.3 μM (Garcia et al. Citation2023). Structural analysis has helped define the ATPase site for RadD (Garcia et al. Citation2022; Tian et al. Citation2023), while modeling has been used to help define the DNA binding site (Tian et al. Citation2023).
The SSB binding pocket on RadD has recently been identified using modeling approaches and validated biochemically, revealing a hydrophobic pocket framed by residues R21, R49, and R145 (Garcia et al. Citation2023). RadD variants with charge reversal mutations to any of these three Arg residues in RadD display a loss of interaction with SSB in co-precipitation experiments and in SSB-Ct binding as measured by FP (Garcia et al. Citation2023). The ATP hydrolysis activity of the variants is not stimulated by SSB (Garcia et al. Citation2023). Interestingly, loss of RadD/SSB interaction does not impact RadD activity in stimulating RecA-mediated strand exchanged, a reaction in which SSB is present, but other RadD functions are impaired (Garcia et al. Citation2023). Loss of radD in E. coli does not produce strong phenotypes, and this is also true for cells expressing chromosomally encoded radD binding pocket mutant alleles. However, when these alleles are combined with loss of recG, the resulting cells are synergistically induced for the SOS response and are sensitized to nitrofurazone and ciprofloxacin (Garcia et al. Citation2023). Collectively, these studies indicate that interaction with SSB is important for some, but not all, RadD functions in cells.
RecG
RecG (76 kDa) is an SF2 helicase that has been implicated in resolving branched recombination intermediates (Whitby et al. Citation1993, Citation1994; Whitby and Lloyd Citation1995; Romero et al. Citation2020) and in preventing over-replication of the genome at sites of DSBs and in the terminus region of the chromosome (Rudolph et al. Citation2008, Citation2009, Citation2010, Citation2013; Azeroglu et al. Citation2016). RecG interacts with SSB in ammonium sulfate co-precipitation and pull-down experiments. The SSB interaction is weakened with the SSB113 or SSBΔC8 variants, indicating the interaction occurs through the SSB-Ct (Buss et al. Citation2008; Yu et al. Citation2016). The SSB interaction stabilizes RecG/ssDNA interactions (Slocum et al. Citation2007; Buss et al. Citation2008), facilitates RecG binding to replication forks (Sun et al. Citation2015), and localizes RecG to replication forks in B. subtilis (Lecointe et al. Citation2007). Interaction with SSB has been reported to stimulate RecG ATPase activity (Slocum et al. Citation2007; Abd Wahab et al. Citation2013).
In contrast to early studies that showed a dependence on the SSB-Ct for the RecG/SSB interaction, pull-down experiments where wild-type and variant His-tagged SSB and native RecG proteins are overexpressed have suggested that the interaction could occur between the SSB IDL and RecG wedge (OB) domain (Ding et al. Citation2020). However, a recent investigation into this proposed binding mode using purified proteins in ITC and FP studies has affirmed that RecG only interacts with the SSB-Ct (Bonde et al. Citation2023). Modeling suggested that the SSB binding pocket on RecG was framed by R467, R474, and R614 in the helicase domain of the protein. Charge reversal mutations to any of the three Arg residues results in loss of the RecG/SSB interaction in vitro and in a two-hybrid assay whereas changes in the RecG wedge domain had no impact. Cells expressing the recG binding pocket mutants are induced for SOS, sensitized to UV exposure, and display increased filamentation (Bonde et al. Citation2023). Deletions encompassing nearly all of the IDL generate SSB variants that support wild type growth and DNA repair capacity in vivo (Bonde et al. Citation2023). Taken together, these results confirm that RecG interacts primarily with the SSB-Ct. Further, the RecG/SSB-Ct interaction is important for many RecG DNA repair functions in vivo (Bonde et al. Citation2023).
RarA
Replication-associated recombination protein or RarA (previously also referred to as MgsA; 49.6 kDa) is a tetrameric DNA repair factor that belongs to the AAA+ family of proteins (Barre et al. Citation2001; Sherratt et al. Citation2004; Shibata et al. Citation2005; Stanage et al. Citation2017). RarA is involved in RecA-independent recombination (Jain, Wood, et al. Citation2021), as well as in later stages of RecFOR-mediated recombination (Jain, Wood, Cox Citation2021). The RarA/SSB interaction was identified by a TAP assay, and binding was confirmed and measured using SPR, revealing a Kd = 0.36 ± 0.02 μΜ (Page et al. Citation2011). The interaction is highly dependent on the SSB-Ct, as RarA has greatly reduced binding to SSBΔC8, SSBΔF, or SSB-mixed (randomized SSB-Ct sequence) variants (Page et al. Citation2011). Interestingly, SSB inhibits DNA-dependent ATPase activity of RarA on ssDNA by competing for DNA binding (Page et al. Citation2011). However, B. subtilis RarA/SsbA interaction results in RarA ATP hydrolysis stimulation (Carrasco et al. Citation2018). The SSB-Ct binding site on RarA has not yet been identified.
Interactions with DNA repair factors: AlkB, DinG, Exonuclease I, topoisomeraase III, uracil DNA glycosylase
AlkB
AlkB (27 kDa) aids in the repair of methyl DNA and RNA adducts through oxidative demethylation (Trewick et al. Citation2002). Some evidence suggests that AlkB may interact with residues 152-169 of SSB IDL (Nigam et al. Citation2018). AlkB repair of long ssDNA substrates improves in the presence of SSB by lowering the KM for the AlkB reaction (Nigam et al. Citation2018), but it is unclear whether this is due to the interaction between AlkB/SSB or due to SSB binding to ssDNA. The SSB binding site on AlkB has not been determined.
DinG
DinG (81 kDa) is a 5′→ 3′ SF2 helicase that unwinds several DNA substrates, although its in vivo role has remained mysterious (Voloshin and Camerini-Otero Citation2007; Boubakri et al. Citation2010). DinG and SSB interact in the absence of ssDNA in size exclusion chromatography experiments (Cheng et al. Citation2012). Mutation of the SSB C-terminal Phe residue to a Cys results in a variant protein (SSB F177C) that no longer co-elutes with DinG, and the same pattern is observed in ammonium sulfate co-precipitation experiments (Cheng et al. Citation2012). DinG helicase activity is stimulated by SSB but inhibited by SSB F177C and gp32 (Cheng et al. Citation2012). Collectively, these results indicate the DinG/SSB interaction is mediated by the SSB-Ct and stimulation of DinG requires an interaction with SSB.
Exonuclease I (ExoI)
ExoI (54.5 kDa) is a 3′→5′ exonuclease with roles in methyl directed mismatch repair (Burdett et al. Citation2001), suppression of illegitimate recombination (Yamaguchi et al. Citation2000), and processing of converging replication forks (Wendel et al. Citation2018). ExoI forms a complex with SSB (Molineux and Gefter Citation1975), and this interaction is mediated by the SSB-Ct (Genschel et al. Citation2000; Lu and Keck Citation2008; Lu et al. Citation2009). Binding between ExoI and SSB C-terminal variants or SSB-Ct variant peptides is greatly reduced (Genschel et al. Citation2000; Lu and Keck Citation2008; Lu et al. Citation2009). ExoI binding to an SSB-Ct peptide measured by FP had a Kd of 136 ± 11 nM (Lu and Keck Citation2008). An ExoI co-crystal structure with SSB-Ct peptide revealed two SSB-Ct associated with ExoI. The potential binding pockets were tested biochemically, revealing only one relevant binding site, framed by residues R148, Y207, and R316 (Lu and Keck Citation2008). ExoI nuclease and deoxyribophosphodiesterase activity is stimulated by SSB (Molineux and Gefter Citation1975; Sandigursky et al. Citation1996), and stimulation requires the physical interaction between full-length SSB with an intact SSB-Ct sequence and ExoI (Lu and Keck Citation2008; Lu et al. Citation2011).
RNase HI
Ribonuclease HI (RNase HI; 17 kDa) digests RNA in RNA-DNA hybrids and plays a role in R-loop removal, blocking DNA replication initiation outside of oriC, and assisting in the removal of RNA primers (Miller et al. Citation1973; Ogawa et al. Citation1984; Ogawa and Okazaki Citation1984). The RNase HI interaction was discovered through a TAP assay and confirmed by measuring the Kd (2.0 ± 0.2 μΜ) of the interaction between purified proteins with ITC (Petzold et al. Citation2015). No binding is detected between RNase HI and SSBΔF or SSB113 variants (Petzold et al. Citation2015). The interaction stimulates RNase HI RNA–DNA hybrid hydrolysis by lowering the reaction KM (Petzold et al. Citation2015). The SSB binding pocket on RNase HI was identified by NMR spectroscopy and X-ray crystallography. The pocket on RNase HI is framed by several well-conserved Arg and Lys residues, including K3, R29, R31, K33, and K60 (Petzold et al. Citation2015; ).
The RNase HI/SSB interaction is important for RNase HI function in cells. The interaction localizes RNase HI to replication forks, and cells expressing an RNase HI binding pocket mutant (encoded by rnhA-K60E) do not exhibit RNase HI focus formation (Wolak et al. Citation2020). This interaction is also important in RNase HI-mediated R-loop removal that clear DNA replication barriers, but the interaction is not important for RNase HI activity in blocking replication initiation at R-loops or in degrading RNA primers (Wolak et al. Citation2020). A rnhA-K60E strain does not share the same synthetic lethality phenotypes as ΔrnhA (Wolak et al. Citation2020). However, expression of rnhA-K60E in Δrep cells leads to rich media-dependent synergistic phenotypes, including a defect in plating efficiency, SOS induction, and cell filamentation (Wolak et al. Citation2020).
Topoisomerase III (top III)
Top III (74 kDa) is a type-IA topoisomerase that functions with RecQ to influence DNA supercoiling and catenation and the resolution of converging replication forks (Digate and Marians Citation1988; Harmon et al. Citation2003; Suski and Marians Citation2008). Top III interacts directly with SSB in ammonium sulfate co-precipitation assays, and its precipitation relies on an intact SSB-Ct (Suski and Marians Citation2008). The Top III/SSB interaction is important for Top III activity in unlinking late replication intermediates (Suski and Marians Citation2008). Top III associates with the replication fork, and this association is achieved by both the Top III/SSB and Top III/DnaX interactions (Lee et al. Citation2019). The SSB binding pocket on Top III has not yet been identified.
Uracil DNA glycosylase (UDG)
UDG (encoded by ung, 24.9 kDa) is involved in base excision repair by removing uracil from DNA and leaving an abasic site (Lindahl Citation1974; Lindahl et al. Citation1977), which is further processed and repaired. The presence of SSB has varying effects on UDG-mediated excision of uracil depending on the type of DNA substrate. In tetraloop hairpin substrates, excision is enhanced at certain positions within the loop but inhibited on single-stranded unstructured DNA substrates (Kumar and Varshney Citation1997; Purnapatre et al. Citation1999).
UDG directly interacts with SSB in a species-specific manner (Handa et al. Citation2001; Acharya and Varshney Citation2002), and the binding of the two proteins has been measured with SPR, revealing a Kd of 0.17 μM (Purnapatre et al. Citation1999; Handa et al. Citation2001). The interaction relies on the SSB C-terminus, based on two-hybrid and gel shift assays with chimeric proteins (Handa et al. Citation2001; Acharya and Varshney Citation2002). Structures of E. coli and D. radiodurans UDG bound to the SSB-Ct peptides are available in the Protein Data Bank (3UF7 and 3UFM).
Interactions with other proteins: Exonuclease IX, vRNA polymerase
Exonuclease IX (exo IX)
Exo IX (encoded by ygdG, 28 kDa) is a flap endonuclease (Anstey-Gilbert et al. Citation2013) that shares 60% sequence identity with the N-terminal region of DNA polymerase I (Hodskinson et al. Citation2007) and is synthetically lethal with polA mutations (Fukushima et al. Citation2007). SSB was identified as an Exo IX protein partner in pull-down assays, and the interaction was confirmed in chemical crosslinking experiments in the absence of DNA (Hodskinson et al. Citation2007). The Exo IX/SSB interaction has not been further characterized.
vRNA polymerase
Coliphage N4 virion RNA (vRNA) polymerase (382.5 kDa) is injected into cells during N4 infection and is involved in the synthesis of early N4 RNAs (Falco et al. Citation1977). vRNA polymerase relies on SSB to carry out its activity in cells, implying that a physical interaction between the two may exist. SSB is required for vRNAP-mediated N4 early transcription on supercoiled templates (Markiewicz et al. Citation1992; Glucksmann-Kuis et al. Citation1996). This effect is specific to E. coli SSB (Markiewicz et al. Citation1992; Glucksmann-Kuis et al. Citation1996) and relies on the 13 C-terminal residues of SSB (Davydova and Rothman-Denes Citation2003).
SSB protein network summary
Properties of proteins within the SSB interactome are listed in . Examination of the collective network of SSB-protein interactions reveals many common elements across the different interactions. 1) Interactions that have been extensively characterized reveal a conserved mode of SSB recognition in which partner proteins dock onto the highly conserved SSB-Ct motif. In addition to their association with the SSB-Ct, RecQ and RecO may also interact with SSB weakly at additional sites, likely involving the SSB OB domain, whereas AlkB may interact outside of the SSB-Ct. SSB-Ct binding sites in structurally-characterized complexes are comprised of hydrophobic pockets that bind the side chain of the C-terminal Phe from SSB along with electropositive elements that complement the negative charges of the alpha-carboxyl group and Asp side chains from the SSB-Ct. 2) In cases where SSB/partner protein interaction affinity has been measured, dissociation constants for the interactions fall within the range of 0.02 μM (RecO) to 14 μM (DnaG), with most being low μM. 3) Interaction with SSB has been closely linked to stimulation of partner protein activity. 4) For a number of proteins, interaction with SSB results in remodeling of SSB/ssDNA interactions, allowing partner proteins to preferentially access ssDNA, whereas non-SIPs are blocked from ssDNA access. 5) Interaction with SSB has been linked to localization of partner proteins to DNA substrates, such as replication forks. 6) Mutations that alter the SSB-Ct sequence have severe consequences in cells, highlighting the importance of SSB/SIP interactions. Collectively, these patterns describe the mechanisms employed by SSB to coordinate genome maintenance events through its vast network of interactions in cells.
As already noted, deletion of 37 of 57 amino acid residues of the SSB IDL has essentially no effect on cell survival, growth, or resistance to DNA damaging agents (Shinn et al. Citation2019, Bonde et al. Citation2023, Sandler et al. Citation2024). Deletion of 47 residues, or the insertion of an mTur2 fluorescent protein domain in the middle of the IDL, results in measurable defects in some DNA repair pathways and some reduced SIP interactions (Bonde et al. Citation2023, Sandler et al. Citation2024). In particular, the PriA-PriB1 and PriA-C replication restart, as well as the RecFOR and RecG-dependent recombination pathways are sensitive to a 47-residue reduction in IDL length (Sandler et al. Citation2024). Defects are relieved when 10 randomized amino acids are added back to SSB variants lacking 47 amino acid residues of the IDL (Bonde et al. Citation2023, Sandler et al. Citation2024). A deletion of all 57 amino acids of the IDL renders the cell inviable. These results may speak to a need for a minimum flexible portion of the IDL to be present to make the acidic SSB-Ct accessible to some or all SIPs.
SSB phase separation
Over the past decade, it has become clear that numerous protein and protein–nucleic acid complexes can form biomolecular condensates and that these may have functional properties in vivo (Banani et al. Citation2017; Shin and Brangwynne Citation2017; Pappu et al. Citation2023). E. coli SSB protein can also undergo phase separation to form such condensates under physiological salt conditions (Harami et al. Citation2020; Kozlov et al. Citation2022; ). SSB phase separation requires its intrinsically disordered C-terminal tails but is inhibited upon binding ssDNA. SSB phase separation in vitro is promoted by increasing concentrations of potassium glutamate, whereas it is inhibited by increasing concentrations of KCl. This is notable since glutamate is the major monovalent anion in bacteria (Leirmo et al. Citation1987; Richey et al. Citation1987). Glutamate generally promotes macromolecular assemblies due to its preferential exclusion, relative to water, from protein surfaces, in particular from glycine and polypeptide backbone amide groups, whereas chloride interacts favorably with those groups (Leirmo et al. Citation1987; Cheng et al. Citation2016; Sengupta et al. Citation2016; Kozlov et al. Citation2022). The E. coli SSB IDL is not highly charged and is rich in glycine residues, and potassium glutamate promotes amide-amide interactions and thus its phase separation. In contrast, the IDL of the P. falciparum SSB is highly charged and contains few glycines, and does not undergo phase separation (Kozlov et al. Citation2015).
Figure 5. Phase separation of SSB. SSB can undergo phase separation to form a biomolecular condensate that is disrupted upon binding ssDNA. SSB phase separation is impacted both by DNA binding and anion type. Phase separation is dependent on the presence of most parts of the IDL, as SSB variants with large IDL deletions do not undergo this process. SIP interaction with SSB may not be limited to SSB that is complexed with ssDNA. Interaction of SIPs with SSB that is phase separated and thus not bound to ssDNA may function in vivo to segregate and store SIPS in a transiently inactive form.
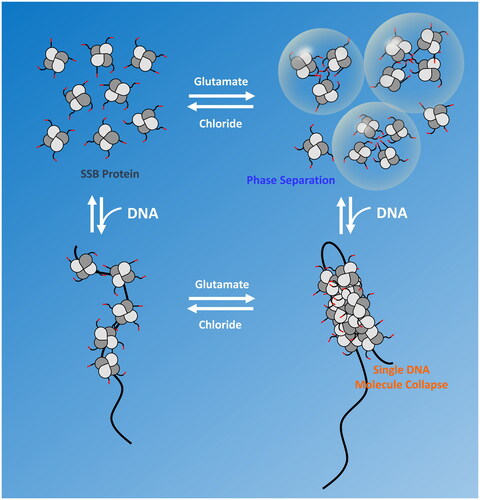
A recent study using structured illumination super resolution microscopy combined with C-terminally GFP-labeled SSB showed that in E. coli cells, SSB localizes to DNA replication forks, but also forms multiple DNA-free protein foci near the inner cell membrane (Zhao et al. Citation2019). However, other studies using widefield fluorescence microscopy on cells expressing SSB proteins labeled on their C-terminus with fluorescent proteins or an SSB variant in which a fluorescent protein was inserted into the IDL region showed SSB foci only at replication forks (Dubiel et al. Citation2019; Spenkelink et al. Citation2019). Although further research is needed, Harami et al. (Citation2020) have speculated that SSB phase separation, and its inhibition by ssDNA binding, may provide a mechanism to sequester SSB protein in vivo until it is needed for genome maintenance activity. SSB interacting proteins (SIPs), such as RecQ and RecO, have also been observed in the SSB condensates (Harami et al. Citation2020), hence this may also provide a mechanism to sequester SIPs until they are needed. Sequestration may be especially critical in cases such as RecO. In the absence of constraints, a RecOR complex will load RecA protein onto any SSB-coated ssDNA (Sakai and Cox Citation2009; Cox et al. Citation2023). However, loading RecA into any gap, including the thousands that are formed on the lagging strand during replication, could result in genomic chaos. Instead, RecO function must be constrained until a lesion-containing postreplication gap that requires repair is identified by RecF (Sakai and Cox Citation2009; Cox et al. Citation2023; Henry, Kaur, et al. Citation2023; Henry, Mbele, Cox Citation2023). The molecular condensates formed by SSB within the cell could well be the needed sequestration mechanism.
When DNA damage is unusually high, such as in the SOS response, the amount of DNA that is single-stranded increases a great deal (Pham et al. Citation2022). The associated binding of most or all of the unbound SSB may serve to also bring in a range of needed DNA repair functions.
Closing summary
SSB is a critical cellular protein in bacteria, binding ssDNA and interacting with a range of other proteins involved in DNA metabolism. The interactions of SSB with other proteins are dominated by interactions with the SSB C-terminus. However, SSB has the capacity to slide along the DNA and transfer rapidly between regions of ssDNA. Its interactions with other proteins are rendered more complex as it is pushed along the DNA or evicted from ssDNA by various polymerases and helicases. SSB may even be recruited to sites where it is needed by means of its interactions with other proteins. In at least some cases, the function of proteins involved in DNA metabolism is highly dependent on that protein’s interaction with SSB. Interactions of many proteins with biomolecular condensates of SSB may serve to sequester the proteins until they are needed for DNA repair or other functions in DNA metabolism.
Disclosure statement
No potential conflict of interest was reported by the author(s).
Additional information
Funding
References
- Abd Wahab S, Choi M, Bianco PR. 2013. Characterization of the ATPase activity of RecG and RuvAB proteins on model fork structures reveals insight into stalled DNA replication fork repair. J Biol Chem. 288(37):26397–26409. doi: 10.1074/jbc.M113.500223.
- Acharya N, Varshney U. 2002. Biochemical properties of single-stranded DNA-binding protein from Mycobacterium smegmatis, a fast-growing mycobacterium and its physical and functional interaction with uracil DNA glycosylases. J Mol Biol. 318(5):1251–1264. doi: 10.1016/s0022-2836(02)00053-0.
- Alberts BM, Frey L. 1970. T4 bacteriophage gene 32: a structural protein in the replication and recombination of DNA. Nature. 227(5265):1313–1318. doi: 10.1038/2271313a0.
- Amundsen SK, Neiman AM, Thibodeaux SM, Smith GR. 1990. Genetic dissection of the biochemical activities of RecBCD enzyme. Genetics. 126(1):25–40. doi: 10.1093/genetics/126.1.25.
- Anderson DG, Kowalczykowski SC. 1997. The translocating RecBCD enzyme stimulates recombination by directing RecA protein onto ssDNA in a chi-regulated manner. Cell. 90(1):77–86. doi: 10.1016/s0092-8674(00)80315-3.
- Anstey-Gilbert CS, Hemsworth GR, Flemming CS, Hodskinson MRG, Zhang J, Sedelnikova SE, Stillman TJ, Sayers JR, Artymiuk PJ. 2013. The structure of Escherichia coli ExoIX-implications for DNA binding and catalysis in flap endonucleases. Nucleic Acids Res. 41(17):8357–8367. doi: 10.1093/nar/gkt591.
- Antony E, Kozlov AG, Nguyen B, Lohman TM. 2012. Plasmodium falciparum SSB tetramer binds single-stranded DNA only in a fully wrapped mode. J Mol Biol. 420(4-5):284–295. doi: 10.1016/j.jmb.2012.04.022.
- Antony E, Lohman TM. 2019. Dynamics of E. coli single stranded DNA binding (SSB) protein-DNA complexes. Semin Cell Dev Biol. 86:102–111. doi: 10.1016/j.semcdb.2018.03.017.
- Antony E, Weiland EA, Korolev S, Lohman TM. 2012. Plasmodium falciparum SSB tetramer wraps single-stranded DNA with similar topology but opposite polarity to E. coli SSB. J Mol Biol. 420(4-5):269–283. doi: 10.1016/j.jmb.2012.04.021.
- Antony E, Weiland E, Yuan Q, Manhart CM, Nguyen B, Kozlov AG, McHenry CS, Lohman TM. 2013. Multiple C-terminal tails within a single E. coli SSB homotetramer coordinate DNA replication and repair. J Mol Biol. 425(23):4802–4819. doi: 10.1016/j.jmb.2013.08.021.
- Arad G, Hendel A, Urbanke C, Curth U, Livneh Z. 2008. Single-stranded DNA-binding protein recruits DNA polymerase V to primer termini on RecA-coated DNA. J Biol Chem. 283(13):8274–8282. doi: 10.1074/jbc.M710290200.
- Aramaki T, Abe Y, Furutani K, Katayama T, Ueda T. 2015. Basic and aromatic residues in the C-terminal domain of PriC are involved in ssDNA and SSB binding. J Biochem. 157(6):529–537. doi: 10.1093/jb/mvv014.
- Arnold DA, Kowalczykowski SC. 1999. RecBCD helicase/nuclease. Encyclopedia of Life Sciences. London: Nature Publishing Group. (http://www.els.net.).
- Azeroglu B, Mawer JSP, Cockram CA, White MA, Hasan AMM, Filatenkova M, Leach DRF. 2016. RecG directs DNA synthesis during double-strand break repair. PLoS Genet. 12(2):e1005799. doi: 10.1371/journal.pgen.1005799.
- Bagchi D, Manosas M, Zhang WT, Manthei KA, Hodeib S, Ducos B, Keck JL, Croquette V. 2018. Single molecule kinetics uncover roles for E. coli RecQ DNA helicase domains and interaction with SSB. Nucleic Acids Res. 46(16):8500–8515. doi: 10.1093/nar/gky647.
- Banani SF, Lee HO, Hyman AA, Rosen MK. 2017. Biomolecular condensates: organizers of cellular biochemistry. Nat Rev Mol Cell Biol. 18(5):285–298. doi: 10.1038/nrm.2017.7.
- Barre FX, Søballe B, Michel B, Aroyo M, Robertson M, Sherratt D. 2001. Circles: the replication-recombination-chromosome segregation connection. Proc Natl Acad Sci U S A. 98(15):8189–8195. doi: 10.1073/pnas.111008998.
- Bell JC, Liu B, Kowalczykowski SC. 2015. Imaging and energetics of single SSB-ssDNA molecules reveal intramolecular condensation and insight into RecOR function. Elife. 4:e08646. doi: 10.7554/eLife.08646.
- Bernstein DA, Eggington JM, Killoran MP, Misic AM, Cox MM, Keck JL. 2004. Crystal structure of the D. radiodurans single-stranded DNA binding protein suggests a novel mechanism for coping with DNA damage. Proc Natl Acad Sci U S A. 101(23):8575–8580. doi: 10.1073/pnas.0401331101.
- Bhattacharyya B, George NP, Thurmes TM, Zhou RB, Jani N, Wessel SR, Sandler SJ, Ha T, Keck JL. 2014. Structural mechanisms of PriA-mediated DNA replication restart. Proc Natl Acad Sci U S A. 111(4):1373–1378. doi: 10.1073/pnas.1318001111.
- Bianco PR. 2017. The tale of SSB. Prog Biophys Mol Biol. 127:111–118. doi: 10.1016/j.pbiomolbio.2016.11.001.
- Bianco PR. 2020. DNA helicase-SSB interactions critical to the regression and restart of stalled DNA replication forks in Escherichia coli. Genes (Basel). 11(5):471. doi: 10.3390/genes11050471.
- Bianco PR. 2021. The mechanism of action of the SSB interactome reveals it is the first OB-fold family of genome guardians in prokaryotes. Protein Sci. 30(9):1757–1775. doi: 10.1002/pro.4140.
- Bianco PR, Lyubchenko YL. 2017. SSB and the RecG DNA helicase: an intimate association to rescue a stalled replication fork. Protein Sci. 26(4):638–649. doi: 10.1002/pro.3114.
- Bianco PR, Pottinger S, Tan HY, Nguyenduc T, Rex K, Varshney U. 2017. The IDL of E. coli SSB links ssDNA and protein binding by mediating protein-protein interactions. Protein Sci. 26(2):227–241. doi: 10.1002/pro.3072.
- Bobst EV, Bobst AM, Perrino FW, Meyer RR, Rein DC. 1985. Variability in the nucleic acid binding site size and the amount of single-stranded DNA binding protein in Escherichia coli. FEBS Lett. 181(1):133–137. doi: 10.1016/0014-5793(85)81128-5.
- Bonde NJ, Henry C, Wood EA, Cox MM, Keck JL. 2023. Interaction with the carboxy-terminal tip of SSB is critical for RecG function in E. coli. Nucleic Acids Res. 51(8):3735–3753. doi: 10.1093/nar/gkad162.
- Bonde NJ, Romero ZJ, Chitteni-Pattu S, Cox MM. 2022. RadD is a RecA-dependent accessory protein that accelerates DNA strand exchange. Nucleic Acids Res. 50(4):2201–2210. doi: 10.1093/nar/gkac041.
- Bonde NJ, Wood EA, Myers KS, Place M, Keck JL, Cox MM. 2023. Identification of recG genetic interactions in Escherichia coli by transposon sequencing. J Bacteriol. 205(12):e00184-23. doi: 10.1128/jb.00184-23.
- Bonner CA, Stukenberg PT, Rajagopalan M, Eritja R, O’Donnell M, McEntee K, Echols H, Goodman MF. 1992. Processive DNA synthesis by DNA polymerase II mediated by DNA polymerase III accessory proteins. J Biol Chem. 267(16):11431–11438. doi: 10.1016/S0021-9258(19)49928-6.
- Boubakri H, de Septenville AL, Viguera E, Michel B. 2010. The helicases DinG, Rep and UvrD cooperate to promote replication across transcription units in vivo. Embo J. 29(1):145–157. doi: 10.1038/emboj.2009.308.
- Brendza KM, Cheng W, Fischer CJ, Chesnik MA, Niedziela-Majka A, Lohman TM. 2005. Autoinhibition of Escherichia coli Rep monomer helicase activity by its 2B subdomain. Proc Natl Acad Sci U S A. 102(29):10076–10081. doi: 10.1073/pnas.0502886102.
- Bujalowski W, Lohman TM. 1986. Escherichia coli single-strand binding protein forms multiple, distinct complexes with single-stranded DNA. Biochemistry. 25(24):7799–7802. doi: 10.1021/bi00372a003.
- Bujalowski W, Lohman TM. 1987. Limited co-operativity in protein-nucleic acid interactions. A thermodynamic model for the interactions of Escherichia coli single strand binding protein with single-stranded nucleic acids in the "beaded", (SSB)65 mode. J Mol Biol. 195(4):897–907. doi: 10.1016/0022-2836(87)90493-1.
- Bujalowski W, Lohman TM. 1989a. Negative co-operativity in Escherichia coli single strand binding protein-oligonucleotide interactions. I. Evidence and a quantitative model. J Mol Biol. 207(1):249–268. doi: 10.1016/0022-2836(89)90454-3.
- Bujalowski W, Lohman TM. 1989b. Negative co-operativity in Escherichia coli single strand binding protein-oligonucleotide interactions. II. Salt, temperature and oligonucleotide length effects. J Mol Biol. 207(1):269–288. doi: 10.1016/0022-2836(89)90455-5.
- Bujalowski W, Overman LB, Lohman TM. 1988. Binding mode transitions of Escherichia coli single strand binding protein-single-stranded DNA complexes. Cation, anion, pH, and binding density effects. J Biol Chem. 263(10):4629–4640. doi: 10.1016/S0021-9258(18)68829-5.
- Bunting KA, Roe SM, Pearl LH. 2003. Structural basis for recruitment of translesion DNA polymerase Pol IV/DinB to the β-clamp. Embo J. 22(21):5883–5892. doi: 10.1093/emboj/cdg568.
- Burckhardt SE, Woodgate R, Scheuermann RH, Echols H. 1988. UmuD mutagenesis protein of Escherichia coli: overproduction, purification, and cleavage by RecA. Proc Natl Acad Sci U S A. 85(6):1811–1815. doi: 10.1073/pnas.85.6.1811.
- Burdett V, Baitinger C, Viswanathan M, Lovett ST, Modrich P. 2001. In vivo requirement for RecJ, ExoVII, ExoI, and ExoX in methyl-directed mismatch repair. Proc Natl Acad Sci U S A. 98(12):6765–6770. doi: 10.1073/pnas.121183298.
- Buss JA, Kimura Y, Bianco PR. 2008. RecG interacts directly with SSB: implications for stalled replication fork regression. Nucleic Acids Res. 36(22):7029–7042. doi: 10.1093/nar/gkn795.
- Cadman CJ, McGlynn P. 2004. PriA helicase and SSB interact physically and functionally. Nucleic Acids Res. 32(21):6378–6387. doi: 10.1093/nar/gkh980.
- Carrasco B, Seco EM, López-Sanz M, Alonso JC, Ayora S. 2018. Bacillus subtilis RarA modulates replication restart. Nucleic Acids Res. 46(14):7206–7220. doi: 10.1093/nar/gky541.
- Chang S, Thrall ES, Laureti L, Piatt SC, Pagès V, Loparo JJ. 2022. Compartmentalization of the replication fork by single-stranded DNA-binding protein regulates translesion synthesis. Nat Struct Mol Biol. 29(9):932–941. doi: 10.1038/s41594-022-00827-2.
- Chao K, Lohman TM. 1990. DNA and nucleotide-induced conformational changes in the Escherichia coli Rep and helicase II (UvrD) proteins. J Biol Chem. 265(2):1067–1076. doi: 10.1016/S0021-9258(19)40159-2.
- Chen SH, Byrne-Nash RT, Cox MM. 2016. Escherichia coli RadD protein functionally interacts with the single-stranded DNA-binding protein. J Biol Chem. 291(39):20779–20786. doi: 10.1074/jbc.M116.736223.
- Cheng W, Brendza KM, Gauss GH, Korolev S, Waksman G, Lohman TM. 2002. The 2B domain of the Escherichia coli Rep protein is not required for DNA helicase activity. Proc Natl Acad Sci U S A. 99(25):16006–16011. doi: 10.1073/pnas.242479399.
- Cheng ZS, Caillet A, Ren BB, Ding HG. 2012. Stimulation of Escherichia coli DNA damage inducible DNA helicase DinG by the single-stranded DNA binding protein SSB. FEBS Lett. 586(21):3825–3830. doi: 10.1016/j.febslet.2012.09.032.
- Cheng X, Guinn EJ, Buechel E, Wong R, Sengupta R, Shkel IA, Record MT. 2016. Basis of protein stabilization by K Glutamate: unfavorable interactions with carbon, oxygen groups. Biophys J. 111(9):1854–1865. doi: 10.1016/j.bpj.2016.08.050.
- Cheng W, Hsieh J, Brendza KM, Lohman TM. 2001. E. coliRep oligomers are required to initiate DNA unwinding in vitro. J Mol Biol. 310(2):327–350. doi: 10.1006/jmbi.2001.4758.
- Cheng KY, Xu H, Chen XY, Wang LY, Tian B, Zhao Y, Hua YJ. 2016. Structural basis for DNA 5’-end resection by RecJ. Elife. 5:e14294. doi: 10.7554/eLife.14294.
- Costes A, Lecointe F, McGovern S, Quevillon-Cheruel S, Polard P. 2010. The C-terminal domain of the bacterial SSB protein acts as a DNA maintenance hub at active chromosome replication forks. PLoS Genet. 6(12):e1001238. doi: 10.1371/journal.pgen.1001238.
- Cox MM, Goodman MF, Keck JL, Oijen A, Lovett ST, Robinson A. 2023. Generation and repair of postreplication gaps in Escherichia coli. Microbiol Mol Biol Rev. 87(2):e00078–00022. doi: 10.1128/mmbr.00078-22.
- Cromie GA, Leach DRF. 2001. Recombinational repair of chromosomal DNA double-strand breaks generated by a restriction endonuclease. Mol Microbiol. 41(4):873–883. doi: 10.1046/j.1365-2958.2001.02553.x.
- Curth U, Genschel J, Urbanke C, Greipel J. 1996. In vitro and in vivo function of the C-terminus of Escherichia coli single-stranded DNA binding protein. Nucleic Acids Res. 24(14):2706–2711. doi: 10.1093/nar/24.14.2706.
- Curth U, Greipel J, Urbanke C, Maass G. 1993. Multiple binding modes of the single-stranded DNA binding protein of Escherichia coli as detected by tryptophan fluorescence and site-directed mutagenesis. Biochemistry. 32(10):2585–2591. doi: 10.1021/bi00061a016.
- Davydova EK, Rothman-Denes LB. 2003. Escherichia coli single-stranded DNA-binding protein mediates template recycling during transcription by bacteriophage N4 virion RNA polymerase. Proc Natl Acad Sci U S A. 100(16):9250–9255. doi: 10.1073/pnas.1133325100.
- Digate RJ, Marians KJ. 1988. Identification of a potent decatenating enzyme from Escherichia coli. J Biol Chem. 263(26):13366–13373.
- Dillingham MS, Kowalczykowski SC. 2008. RecBCD enzyme and the repair of double-stranded DNA breaks. Microbiol Mol Biol Rev. 72(4):642–671, Table of Contents. doi: 10.1128/MMBR.00020-08.
- Ding WF, Tan HY, Zhang JX, Wilczek LA, Hsieh KR, Mulkin JA, Bianco PR. 2020. The mechanism of Single strand binding protein-RecG binding: implications for SSB interactome function. Protein Sci. 29(5):1211–1227. doi: 10.1002/pro.3855.
- Dubiel K, Henry C, Spenkelink LM, Kozlov AG, Wood EA, Jergic S, Dixon NE, van Oijen AM, Cox MM, Lohman TM, et al. 2020. Development of a single-stranded DNA-binding protein fluorescent fusion toolbox. Nucleic Acids Res. 48(11):6053–6067. doi: 10.1093/nar/gkaa320.
- Dubiel K, Myers AR, Kozlov AG, Yang O, Zhang JC, Ha T, Lohman TM, Keck JL. 2019. Structural mechanisms of cooperative DNA binding by bacterial single-stranded DNA-binding proteins. J Mol Biol. 431(2):178–195. doi: 10.1016/j.jmb.2018.11.019.
- Falco SC, Laan KV, Rothman-Denes LB. 1977. Virion-associated RNA polymerase required for bacteriophage N4 development. Proc Natl Acad Sci U S A. 74(2):520–523. doi: 10.1073/pnas.74.2.520.
- Fedorov R, Witte G, Urbanke C, Manstein DJ, Curth U. 2006. 3D structure of Thermus aquaticus single-stranded DNA-binding protein gives insight into the functioning of SSB proteins. Nucleic Acids Res. 34(22):6708–6717. doi: 10.1093/nar/gkl1002.
- Ferrari ME, Bujalowski W, Lohman TM. 1994. Cooperative binding of Escherichia coli SSB to single-stranded DNA in the (SSB)(35) binding mode. J Mol Biol. 236(1):106–123. doi: 10.1006/jmbi.1994.1122.
- Ferrari ME, Fang J, Lohman TM. 1997. A mutation in E. coli SSB protein (W54S) alters intra-tetramer negative cooperativity and inter-tetramer positive cooperativity for single-stranded DNA binding. Biophys Chem. 64(1-3):235–251. doi: 10.1016/s0301-4622(96)02223-5.
- Fukushima S, Itaya M, Kato H, Ogasawara N, Yoshikawa H. 2007. Reassessment of the in vivo functions of DNA polymerase I and RNaseH in bacterial cell growth. J Bacteriol. 189(23):8575–8583. doi: 10.1128/JB.00653-07.
- Furukohri A, Nishikawa Y, Akiyama MT, Maki H. 2012. Interaction between Escherichia coli DNA polymerase IV and single-stranded DNA-binding protein is required for DNA synthesis on SSB-coated DNA. Nucleic Acids Res. 40(13):6039–6048. doi: 10.1093/nar/gks264.
- Garcia MAO, Satyshur KA, Cox MM, Keck JL. 2022. X-ray crystal structure of the Escherichia coli RadD DNA repair protein bound to ADP reveals a novel zinc ribbon domain. PLoS One. 17(4):10.
- Garcia MAO, Wood EA, Keck JL, Cox MM. 2023. Interaction with single-stranded DNA-binding protein modulates Escherichia coli RadD DNA repair activities. J Biol Chem. 299(6):104773. doi: 10.1016/j.jbc.2023.104773.
- Genschel J, Curth U, Urbanke C. 2000. Interaction of E. coli single-stranded DNA binding protein (SSB) with exonuclease I. The carboxy-terminus of SSB is the recognition site for the nuclease. Biol Chem. 381(3):183–192. doi: 10.1515/BC.2000.025.
- George NP, Ngo KV, Chitteni-Pattu S, Norais CA, Battista JR, Cox MM, Keck JL. 2012. Structure and cellular dynamics of Deinococcus radiodurans single-stranded DNA (ssDNA)-binding protein (SSB)-DNA complexes. J Biol Chem. 287(26):22123–22132. doi: 10.1074/jbc.M112.367573.
- Gibb B, Ye LF, Gergoudis SC, Kwon YH, Niu HY, Sung P, Greene EC. 2014. Concentration-dependent exchange of replication protein A on single-stranded DNA revealed by single-moleculeimaging. PLoS One. 9(2):e87922. doi: 10.1371/journal.pone.0087922.
- Glover BP, McHenry CS. 1998. The χψ subunits of DNA polymerase III holoenzyme bind to single-stranded DNA-binding protein (SSB) and facilitate replication of an SSB-coated template. J Biol Chem. 273(36):23476–23484. doi: 10.1074/jbc.273.36.23476.
- Glucksmann-Kuis MA, Dai X, Markiewicz P, Rothman-Denes LB. 1996. E. coli SSB activates N4 virion RNA polymerase promoters by stabilizing a DNA hairpin required for promoter recognition. Cell. 84(1):147–154. doi: 10.1016/s0092-8674(00)81001-6.
- Green M, Hatter L, Brookes E, Soultanas P, Scott DJ. 2016. Defining the intrinsically disordered C-terminal domain of SSB reveals DNA-mediated compaction. J Mol Biol. 428(2 Pt A):357–364. doi: 10.1016/j.jmb.2015.12.007.
- Greenberg J, Berends LJ, Donch J, Green MHL. 1974. exrB-malB-linked gene in Excherichia coli B involved in sensitivity to radiation and filament formation. Genet Res. 23(2):175–184. doi: 10.1017/s0016672300014798.
- Greenberg J, Donch J. 1974. Sensitivity to elevated temperatures in exrB strains of Escherichia coli. Mutat Res. 25(3):403–405. doi: 10.1016/0027-5107(74)90070-0.
- Griffith JD, Harris LD, Register J. 1984. Visualization of SSB-ssDNA complexes active in the assembly of stable RecA-DNA filaments. Cold Spring Harb Symp Quant Biol. 49(0):553–559. doi: 10.1101/sqb.1984.049.01.062.
- Hamon L, Pastré D, Dupaigne P, Le Breton C, Le Cam E, Piétrement O. 2007. High-resolution AFM imaging of single-stranded DNA-binding (SSB) protein-DNA complexes. Nucleic Acids Res. 35(8):e58–e58. doi: 10.1093/nar/gkm147.
- Han ES, Cooper DL, Persky NS, Sutera VA, Whitaker RD, Montello ML, Lovett ST. 2006. RecJ exonuclease: substrates, products and interaction with SSB. Nucleic Acids Res. 34(4):1084–1091. doi: 10.1093/nar/gkj503.
- Handa P, Acharya N, Varshney U. 2001. Chimeras between single-stranded DNA-binding proteins from Escherichia coli and Mycobacterium tuberculosis reveal that their C-terminal domains interact with uracil DNA glycosylases. J Biol Chem. 276(20):16992–16997. doi: 10.1074/jbc.M100393200.
- Handa N, Morimatsu K, Lovett ST, Kowalczykowski SC. 2009. Reconstitution of initial steps of dsDNA break repair by the RecF pathway of E. coli. Genes Dev. 23(10):1234–1245. doi: 10.1101/gad.1780709.
- Harami GM, Kovács ZJ, Pancsa R, Pálinkás J, Baráth V, Tárnok K, Málnási-Csizmadia A, Kovács M. 2020. Phase separation by ssDNA binding protein controlled via protein-protein and protein-DNA interactions. Proc Natl Acad Sci U S A. 117(42):26206–26217. doi: 10.1073/pnas.2000761117.
- Harami GM, Seol Y, In J, Ferencziová V, Martina M, Gyimesi M, Sarlós K, Kovács ZJ, Nagy NT, Sun Y, et al. 2017. Shuttling along DNA and directed processing of D-loops by RecQ helicase support quality control of homologous recombination. Proc Natl Acad Sci U S A. 114(4):E466–E475. doi: 10.1073/pnas.1615439114.
- Harmon FG, Brockman JP, Kowalczykowski SC. 2003. RecQ helicase stimulates both DNA catenation and changes in DNA topology by topoisomerase III. J Biol Chem. 278(43):42668–42678. doi: 10.1074/jbc.M302994200.
- Harmon FG, DiGate RJ, Kowalczykowski SC. 1999. RecQ helicase and topoisomerase III comprise a novel DNA strand passage function: a conserved mechanism for control of DNA recombination. Mol Cell. 3(5):611–620. doi: 10.1016/s1097-2765(00)80354-8.
- Harmon FG, Kowalczykowski SC. 1998. RecQ helicase, in concert with RecA and SSB proteins, initiates and disrupts DNA recombination. Genes Dev. 12(8):1134–1144. doi: 10.1101/gad.12.8.1134.
- Harmon FG, Kowalczykowski SC. 2001. Biochemical characterization of the DNA helicase activity of the Escherichia coli RecQ helicase. J Biol Chem. 276(1):232–243. doi: 10.1074/jbc.M006555200.
- Heller RC, Marians KJ. 2005. The disposition of nascent strands at stalled replication forks dictates the pathway of replisome loading during restart. Mol Cell. 17(5):733–743. doi: 10.1016/j.molcel.2005.01.019.
- Henry C, Kaur G, Cherry ME, Henrikus SS, Bonde NJ, Sharma N, Beyer HA, Wood EA, Chitteni-Pattu S, van Oijen AM, et al. 2023. RecF protein function in targeting RecFOR to postreplication gaps II: RecF interaction with replisomes. Nucleic Acids Res. 51(11):5714–5742. doi: 10.1093/nar/gkad310.
- Henry C, Mbele N, Cox MM. 2023. RecF protein function in targeting RecFOR to postreplication gaps I: DNA binding specificities and requirements of RecF and RecFR. Nucleic Acids Res. 51(11):5699–5713. doi: 10.1093/nar/gkad311.
- Hobbs MD, Sakai A, Cox MM. 2007. SSB protein limits RecOR binding onto single-stranded DNA. J Biol Chem. 282(15):11058–11067. doi: 10.1074/jbc.M611007200.
- Hodskinson MRG, Allen LM, Thomson DP, Sayers JR. 2007. Molecular interactions of Escherichia coli ExoIX and identification of its associated 3’-5’ exonuclease activity. Nucleic Acids Res. 35(12):4094–4102. doi: 10.1093/nar/gkm396.
- Hormeno S, Wilkinson OJ, Alcart-Ramos C, Moreno-Herrero F. 2022. Human HELB is a processive motor protein that catalyzes RPA clearance from single-stranded DNA. Proc. Natl. Acad. Sci USA. 119(15):e2112376119.
- Huang YH, Huang CY. 2018. The glycine-rich flexible region in SSB is crucial for PriA stimulation. RSC Adv. 8(61):35280–35288. doi: 10.1039/c8ra07306f.
- Huang YH, Lin MJ, Huang CY. 2013. Yeast two-hybrid Analysis of PriB-interacting proteins in replication restart primosome: a proposed PriB-SSB interaction model. Protein J. 32(6):477–483. doi: 10.1007/s10930-013-9509-y.
- Ivancic-Bace I, Salaj-Smic E, Brcic-Kostic K. 2005. Effects of recJ, recQ, and recFOR mutations on recombination in nuclease-deficient recB recD double mutants of Escherichia coli. J Bacteriol. 187(4):1350–1356. doi: 10.1128/JB.187.4.1350-1356.2005.
- Jain K, Wood EA, Cox MM. 2021. The rarA gene as part of an expanded RecFOR recombination pathway: negative epistasis and synthetic lethality with ruvB, recG, and recQ. PLoS Genet. 17(12):e1009972. doi: 10.1371/journal.pgen.1009972.
- Jain K, Wood EA, Romero ZJ, Cox MM. 2021. RecA-independent recombination: dependence on the Escherichia coli RarA protein. Mol Microbiol. 115(6):1122–1137. doi: 10.1111/mmi.14655.
- Johnson BF. 1984. Two-dimensional electrophoretic analysis of the regulation of SOS proteins in three ssb mutants. Arch Microbiol. 138(2):106–112. doi: 10.1007/BF00413009.
- Kantake N, Madiraju M, Sugiyama T, Kowalczykowski SC. 2002. Escherichia coli RecO protein anneals ssDNA complexed with its cognate ssDNA-binding protein: a common step in genetic recombination. Proc Natl Acad Sci U S A. 99(24):15327–15332. doi: 10.1073/pnas.252633399.
- Kelman Z, Yuzhakov A, Andjelkovic J, O’Donnell M. 1998. Devoted to the lagging strand-the subunit of DNA polymerase III holoenzyme contacts SSB to promote processive elongation and sliding clamp assembly. Embo J. 17(8):2436–2449. doi: 10.1093/emboj/17.8.2436.
- Klimova AN, Sandler SJ. 2020. Mutational analysis of residues in PriA and PriC affecting their ability to interact with SSB in Escherichia coli K-12. J Bacteriol. 202(23):14. doi: 10.1128/JB.00404-20.
- Kornberg T, Gefter ML. 1971. Purification and DNA synthesis in cell-free extracts - properties of DNA polymerase II. Proc Natl Acad Sci U S A. 68(4):761–764. doi: 10.1073/pnas.68.4.761.
- Kowalczykowski SC, Lonberg N, Newport JW, von Hippel PH. 1981. Interactions of bacteriophage T4-coded Gene 32 protein with nucleic acids. 1. characteriation of the binding interactions. J Mol Biol. 145(1):75–104. doi: 10.1016/0022-2836(81)90335-1.
- Kowalczykowski SC, Roman LJ. 1990. Reconstitution of homologous pairing activity dependent upon the combined activities of purified E. coli RecA, RecBCD, and SSB proteins. In: Richardson CC, Lehman IR, editors. Molecular mechanisms in DNA replication and recombination. New York: Alan R. Liss; p. 357–373.
- Kozlov AG, Cheng X, Zhang HS, Shinn MK, Weiland E, Nguyen B, Shkel IA, Zytkiewicz E, Finkelstein IJ, Record MT, et al. 2022. How glutamate promotes liquid-liquid phase separation and DNA binding cooperativity of E. coli SSB protein. J Mol Biol. 434(9):167562. doi: 10.1016/j.jmb.2022.167562.
- Kozlov AG, Cox MM, Lohman TM. 2010. Regulation of Single-stranded DNA Binding by the C Termini of Escherichia coli Single-stranded DNA-binding (SSB) Protein. J Biol Chem. 285(22):17246–17252. doi: 10.1074/jbc.M110.118273.
- Kozlov AG, Jezewska MJ, Bujalowski W, Lohman TM. 2010. Binding specificity of Escherichia coli single-stranded DNA binding protein for the χ subunit of DNA pol III holoenzyme and PriA helicase. Biochemistry. 49(17):3555–3566. doi: 10.1021/bi100069s.
- Kozlov AG, Lohman TM. 2002. Kinetic mechanism of direct transfer of Escherichia coli SSB tetramers between single-stranded DNA molecules. Biochemistry. 41(39):11611–11627. doi: 10.1021/bi020361m.
- Kozlov AG, Lohman TM. 2021. Probing E. coli SSB protein-DNA topology by reversing DNA backbone polarity. Biophys J. 120(8):1522–1533. doi: 10.1016/j.bpj.2021.02.025.
- Kozlov AG, Shinn MK, Lohman TM. 2019. Regulation of nearest-neighbor cooperative binding of E. coli SSB protein to DNA. Biophys J. 117(11):2120–2140. doi: 10.1016/j.bpj.2019.09.047.
- Kozlov AG, Shinn MK, Weiland EA, Lohman TM. 2017. Glutamate promotes SSB protein-protein Interactions via intrinsically disordered regions. J Mol Biol. 429(18):2790–2801. doi: 10.1016/j.jmb.2017.07.021.
- Kozlov AG, Weiland E, Mittal A, Waldman V, Antony E, Fazio N, Pappu RV, Lohman TM. 2015. Intrinsically disordered C-Terminal tails of E. coli single-stranded DNA binding protein regulate cooperative binding to single-stranded DNA. J Mol Biol. 427(4):763–774. doi: 10.1016/j.jmb.2014.12.020.
- Kumar NV, Varshney U. 1997. Contrasting effects of single stranded DNA binding protein on the activity of uracil DNA glycosylase from Escherichia coli towards different DNA substrates. Nucleic Acids Res. 25(12):2336–2343. doi: 10.1093/nar/25.12.2336.
- Kunzelmann S, Morris C, Chavda AP, Eccleston JF, Webb MR. 2010. Mechanism of interaction between single-stranded DNA binding protein and DNA. Biochemistry. 49(5):843–852. doi: 10.1021/bi901743k.
- Lasken RS, Kornberg A. 1988. The promosomal protein n’ of Escherichia coli is a DNA helicase. J Biol Chem. 263(12):5512–5518.
- Lecointe F, Sérèna C, Velten M, Costes A, McGovern S, Meile J-C, Errington J, Ehrlich SD, Noirot P, Polard P. 2007. Anticipating chromosomal replication fork arrest: SSB targets repair DNA helicases to active forks. Embo J. 26(19):4239–4251. doi: 10.1038/sj.emboj.7601848.
- Lee EH, Kornberg A. 1991. Replication deficiencies in priA mutants of Escherichia coli lacking the primosomal replication n’ protein. Proc Natl Acad Sci U S A. 88(8):3029–3032. doi: 10.1073/pnas.88.8.3029.
- Lee KS, Marciel AB, Kozlov AG, Schroeder CM, Lohman TM, Ha T. 2014. Ultrafast redistribution of E. coli SSB along long single-stranded DNA via intersegment transfer. J Mol Biol. 426(13):2413–2421. doi: 10.1016/j.jmb.2014.04.023.
- Lee CM, Wang GS, Pertsinidis A, Marians KJ. 2019. Topoisomerase III acts at the replication fork to remove precatenanes. J Bacteriol. 201(7):e00563–00518. doi: 10.1128/JB.00563-18.
- Leirmo S, Harrison C, Cayley DS, Burgess RR, Record MT.Jr. 1987. Replacement of potassium chloride by potassium glutamate dramatically enhances protein-DNA interactions in vitro. Biochemistry. 26(8):2095–2101. doi: 10.1021/bi00382a006.
- Li GW, Burkhardt D, Gross C, Weissman JS. 2014. Quantifying absolute protein synthesis rates reveals principles underlying allocation of cellular resources. Cell. 157(3):624–635. doi: 10.1016/j.cell.2014.02.033.
- Lindahl T. 1974. N-glycosidase from Escherichia coli that releases free uracil from DNA containing deaminated cytosine residues. Proc Natl Acad Sci U S A. 71(9):3649–3653. doi: 10.1073/pnas.71.9.3649.
- Lindahl T, Ljungquist S, Siegert W, Nyberg B, Sperens B. 1977. DNA N-glycosidases - properties of uracil N-glycosidase from Escherichia coli. J Biol Chem. 252(10):3286–3294.
- Lo ATY. 2012. Protein dynamics on the lagging strand during DNA synthesis. [dissertation]. Wollongong, Australia: School of Chemistry, University of Wollongong. https://ro.uow.edu.au/theses/3684.
- Lohman TM. 1984a. Kinetics and mechanism of dissociation of cooperatively bound T4—gene 32-protein-single-strand DNA complexes.1. Irreversible dissociatioin induced by sodium chloride concentration jumps. Biochemistry. 23(20):4656–4665. doi: 10.1021/bi00315a022.
- Lohman TM. 1984b. Kinetics and mechanism of dissociation of cooperatively bound T4—gene 32-protein-single-strand DNA complexes. 2. Changes in mechanism as a function of sodium chloride concentration and other solution variables. Biochemistry. 23(20):4665–4675. doi: 10.1021/bi00315a023.
- Lohman TM, Bujalowski W. 1988. Negative cooperativity within individual tetramers of Escherichia coli single strand binding protein is responsible for the transition between the (SSB)35 and (SSB)56 DNA binding modes. Biochemistry. 27(7):2260–2265. doi: 10.1021/bi00407a002.
- Lohman TM, Bujalowski W. 1994. Effects of base composition on the negatoive cooperativity and binding mode transitions of Escherichia coli SSB-single-stranded DNA complexes. Biochemistry. 33(20):6167–6176. doi: 10.1021/bi00186a016.
- Lohman TM, Bujalowski W, Overman LB, Wei TF. 1988. Interactions of the Escherichia coli single-strand binding (SSB) protein with SS nucleic acids- binding mode transitions and equilibrium binding studies. Biochem Pharmacol. 37(9):1781–1782. doi: 10.1016/0006-2952(88)90443-1.
- Lohman TM, Ferrari ME. 1994. Escherichia coli single-stranded DNA-binding protein: multiple DNA-binding modes and cooperativities. Annu Rev Biochem. 63(1):527–570. doi: 10.1146/annurev.bi.63.070194.002523.
- Lohman TM, Kowalczykowski SC. 1981. Kinetics and mechanism of the association of the bacteriophage T4 gene 32 (heliz destabilizing) protein with single-stranded nucleic acids - evidence for protein translocation. J Mol Biol. 152(1):67–109. doi: 10.1016/0022-2836(81)90096-6.
- Lohman TM, Overman LB. 1985. Two binding modes in Escherichia coli single strand binding protein-single stranded DNA complexes. Modulation by NaCl concentration. J Biol Chem. 260(6):3594–3603.
- Lohman TM, Overman LB, Datta S. 1986. Salt-dependent changes in the DNA binding co-operativity of Escherichia coli single strand binding protein. J Mol Biol. 187(4):603–615. doi: 10.1016/0022-2836(86)90338-4.
- Lopper M, Boonsombat R, Sandler SJ, Keck JL. 2007. A hand-off mechanism for primosome assembly in replication restart. Mol Cell. 26(6):781–793. doi: 10.1016/j.molcel.2007.05.012.
- Lopper M, Holton JM, Keck JL. 2004. Crystal structure of PriB, a component of the Escherichia coli replication restart primosome. Structure. 12(11):1967–1975. doi: 10.1016/j.str.2004.09.004.
- Lu D, Keck JL. 2008. Structural basis of Escherichia coli single-stranded DNA-binding protein stimulation of exonuclease I. Proc Natl Acad Sci U S A. 105(27):9169–9174. doi: 10.1073/pnas.0800741105.
- Lu D, Myers AR, George NP, Keck JL. 2011. Mechanism of Exonuclease I stimulation by the single-stranded DNA-binding protein. Nucleic Acids Res. 39(15):6536–6545. doi: 10.1093/nar/gkr315.
- Lu D, Windsor MA, Gellman SH, Keck JL. 2009. Peptide inhibitors identify roles for SSB C-terminal residues in SSB/Exonuclease I complex formation. Biochemistry. 48(29):6764–6771. doi: 10.1021/bi900361r.
- Marceau AH, Bahng S, Massoni SC, George NP, Sandler SJ, Marians KJ, Keck JL. 2011. Structure of the SSB-DNA polymerase III interface and its role in DNA replication. Embo J. 30(20):4236–4247. doi: 10.1038/emboj.2011.305.
- Markiewicz P, Malone C, Chase JW, Rothman-Denes LB. 1992. Escherichia coli single-stranded DNA binding protein is a supercoiled template-dependent transcriptional activator of N4 virion RNA-polymerase. Genes Dev. 6(10):2010–2019. doi: 10.1101/gad.6.10.2010.
- Matsumoto T, Morimoto Y, Shibata N, Kinebuchi T, Shimamoto N, Tsukihara T, Yasuoka N. 2000. Roles of functional loops and the C-terminal segment of a single-stranded DNA binding protein elucidated by x-ray structure analysis. J Biochem. 127(2):329–335. doi: 10.1093/oxfordjournals.jbchem.a022611.
- McCool JD, Sandler SJ. 2001. Effects of mutations involving cell division, recombination, and chromosome dimer resolution on a priA2: : kan mutant. Proc Natl Acad Sci U S A. 98(15):8203–8210. doi: 10.1073/pnas.121007698.
- McDonald JP, Maury EE, Levine AS, Woodgate R. 1998. Regulation of UmuD cleavage: role of the amino-terminal tail. J Mol Biol. 282(4):721–730. doi: 10.1006/jmbi.1998.2044.
- Mersch KN, Sokoloski JE, Nguyen B, Galletto R, Lohman TM. 2023. Helicase" activity promoted through dynamic interactions between a ssDNA translocase and a diffusing SSB protein. Proc Natl Acad Sci U S A. 120(15):e2216777120. doi: 10.1073/pnas.2216777120.
- Meyer RR, Laine PS. 1990. The single-stranded DNA-binding protein of Escherichia coli. Microbiol Rev. 54(4):342–380. doi: 10.1128/mr.54.4.342-380.1990.
- Meyer RR, Rein DC, Glassberg J. 1982. The product of the lexC gene of Escherichia coli is single-stranded DNA-binding protein. J Bacteriol. 150(1):433–435. doi: 10.1128/jb.150.1.433-435.1982.
- Michel B, Sandler SJ. 2017. Replication restart in bacteria. J Bacteriol. 199(13):e00102-17. doi: 10.1128/JB.00102-17.
- Miller RC, Taylor DM, Mackay K, Smith HW. 1973. Replication of T4 DNA in Escherichia coli treated with toluene. J Virol. 12(6):1195–1203. doi: 10.1128/JVI.12.6.1195-1203.1973.
- Mills M, Harami GM, Seol Y, Gyimesi M, Martina M, Kovács ZJ, Kovács M, Neuman KC. 2017. RecQ helicase triggers a binding mode change in the SSB-DNA complex to efficiently initiate DNA unwinding. Nucleic Acids Res. 45(20):11878–11890. doi: 10.1093/nar/gkx939.
- Mishra G, Bigman LS, Levy Y. 2020. ssDNA diffuses along replication protein A via a reptation mechanism. Nucleic Acids Res. 48(4):1701–1714. doi: 10.1093/nar/gkz1202.
- Molineux IJ, Gefter ML. 1974. Properties of Escherichia coli DNA binding (unwinding) protein - interaction with DNA polymerase and DNA. Proc Natl Acad Sci U S A. 71(10):3858–3862. doi: 10.1073/pnas.71.10.3858.
- Molineux IJ, Gefter ML. 1975. Properties of the Escherichia coli DNA-binding (unwinding) protein interaction with nucleolytic enzymes and DNA. J Mol Biol. 98(4):811–825. doi: 10.1016/s0022-2836(75)80012-x.
- Morimatsu K, Kowalczykowski SC. 2014. RecQ helicase and RecJ nuclease provide complementary functions to resect DNA for homologous recombination. Proc Natl Acad Sci U S A. 111(48):E5133–E5142. doi: 10.1073/pnas.1420009111.
- Morse M, Navarro Roby F, Kinare M, McIsaac J, Williams MC, Beuning PJ. 2023. DNA damage alters binding conformations of E. coli single-stranded DNA-binding protein. Biophys J. 122(19):3950–3958. doi: 10.1016/j.bpj.2023.08.018.
- Naue N, Beerbaum M, Bogutzki A, Schmieder P, Curth U. 2013. The helicase-binding domain of Escherichia coli DnaG primase interacts with the highly conserved C-terminal region of single-stranded DNA-binding protein. Nucleic Acids Res. 41(8):4507–4517. doi: 10.1093/nar/gkt107.
- Naue N, Fedorov R, Pich A, Manstein DJ, Curth U. 2011. Site-directed mutagenesis of the χ subunit of DNA polymerase III and single-stranded DNA-binding protein of E. coli reveals key residues for their interaction. Nucleic Acids Res. 39(4):1398–1407. doi: 10.1093/nar/gkq988.
- Naufer MN, Morse M, Möller GB, McIsaac J, Rouzina I, Beuning PJ, Williams MC. 2021. Multiprotein E. coli SSB ssDNA complex shows both stable binding and rapid dissociation due to interprotein interactions. Nucleic Acids Res. 49(3):1532–1549. doi: 10.1093/nar/gkaa1267.
- Newcomb ESP, Douma LG, Morris LA, Bloom LB. 2022. The Escherichia coli clamp loader rapidly remodels SSB on DNA to load clamps. Nucleic Acids Res. 50(22):12872–12884. doi: 10.1093/nar/gkac1169.
- Nguyen B, Shinn MK, Weiland E, Lohman TM. 2021. Regulation of E. coli Rep helicase activity by PriC. J Mol Biol. 433(15):167072. doi: 10.1016/j.jmb.2021.167072.
- Nguyen B, Sokoloski J, Galletto R, Elson EL, Wold MS, Lohman TM. 2014. Diffusion of human replication protein A along single-stranded DNA. J Mol Biol. 426(19):3246–3261. doi: 10.1016/j.jmb.2014.07.014.
- Nigam R, Mohan M, Shivange G, Dewangan PK, Anindya R. 2018. Escherichia coli AlkB interacts with single-stranded DNA binding protein SSB by an intrinsically disordered region of SSB. Mol Biol Rep. 45(5):865–870. doi: 10.1007/s11033-018-4232-6.
- Nohmi T, Battista JR, Dodson LA, Walker GC. 1988. RecA-mediated cleavage activates UmuD for mutagenesis: mechanistic relationship between transcriptional derepression and posttranslational activation. Proc Natl Acad Sci U S A. 85(6):1816–1820. doi: 10.1073/pnas.85.6.1816.
- Nurse P, Zavitz KH, Marians KJ. 1991. Inactivation of the Escherichia coli priA DNA replication protein induces the SOS response. J Bacteriol. 173(21):6686–6693. doi: 10.1128/jb.173.21.6686-6693.1991.
- Ogawa T, Okazaki T. 1984. Function of RNase H in DNA replication revealed by RNase H defective mutants of Escherichia coli. Mol Gen Genet. 193(2):231–237. doi: 10.1007/BF00330673.
- Ogawa T, Pickett GG, Kogoma T, Kornberg A. 1984. RNase H confers specificity in the DnaA-dependent intitiation of replication at the unique origin of the Escherichia coli chromosome in vivo and in vitro. Proc Natl Acad Sci U S A. 81(4):1040–1044. doi: 10.1073/pnas.81.4.1040.
- Overman LB, Bujalowski W, Lohman TM. 1988. Equilibrium binding of Escherichia coli single strand binding protein to single-stranded nucleic acids in the (SSB)65 binding mode - cation and anion effects and polynucleotide specificity. Biochemistry. 27(1):456–471. doi: 10.1021/bi00401a067.
- Page AN. 2012. Structural and biochemical studies on the Escherichia coli protein MgsA [dissertation]. Madison, Wisconsin: University of Wisconsin-Madison.
- Page AN, George NP, Marceau AH, Cox MM, Keck JL. 2011. Structure and biochemical activities of Escherichia coli MgsA. J Biol Chem. 286(14):12075–12085. doi: 10.1074/jbc.M110.210187.
- Pappu RV, Cohen SR, Dar F, Farag M, Kar M. 2023. Phase transitions of associative biomacromolecules. Chem Rev. 123(14):8945–8987. doi: 10.1021/acs.chemrev.2c00814.
- Petzold C, Marceau AH, Miller KH, Marqusee S, Keck JL. 2015. Interaction with single-stranded DNA-binding protein stimulates Escherichia coli ribonuclease HI enzymatic activity. J Biol Chem. 290(23):14626–14636. doi: 10.1074/jbc.M115.655134.
- Pham P, Shao J, Cox MM, Goodman MF. 2022. Genomic landscape of single-stranded DNA gapped intermediates in Escherichia coli. Nucleic Acids Res. 50(2):937–951. doi: 10.1093/nar/gkab1269.
- Pham P, Wood EA, Cox MM, Goodman MF. 2023. RecA and SSB genome-wide distribution in ssDNA gaps and ends in Escherichia coli. Nucleic Acids Res. 51(11):5527–5546. doi: 10.1093/nar/gkad263.
- Ponomarev VA, Makarova KS, Aravind L, Koonin EV. 2003. Gene duplication with displacement and rearrangement: origin of the bacterial replication protein PriB from the single-stranded DNA-Binding protein SSB. J Mol Microbiol Biotechnol. 5(4):225–229. doi: 10.1159/000071074.
- Purnapatre K, Handa P, Venkatesh J, Varshney U. 1999. Differential effects of single-stranded DNA binding proteins (SSBs) on uracil DNA glycosylases (UDGs) from Escherichia coli and mycobacteria. Nucleic Acids Res. 27(17):3487–3492. doi: 10.1093/nar/27.17.3487.
- Raghunathan S, Kozlov AG, Lohman TM, Waksman G. 2000. Structure of the DNA binding domain of E. coli SSB bound to ssDNA. Nat Struct Biol. 7(8):648–652. doi: 10.1038/77943.
- Raghunathan S, Ricard CS, Lohman TM, Waksman G. 1997. Crystal structure of the homo-tetrameric DNA binding domain of Escherichia coli single-stranded DNA-binding protein determined by multiwavelength x-ray diffraction on the selenomethionyl protein at 2.9-A resolution. Proc Natl Acad Sci U S A. 94(13):6652–6657. doi: 10.1073/pnas.94.13.6652.
- Richey B, Cayley DS, Mossing MC, Kolka C, Anderson CF, Farrar TC, Record MT.Jr. 1987. Variability of the intracellular ionic environment of Escherichia coli. Differences between in vitro and in vivo effects of ion concentrations on protein-DNA interactions and gene expression. J Biol Chem. 262(15):7157–7164. doi: 10.1016/S0021-9258(18)48218-X.
- Römer R, Schomburg U, Krauss G, Maass G. 1984. Escherichia coli single-stranded DNA binding proteins is mobile on DNA- H1-NMR study of its interaction with oligonucleotide and polynucleotide. Biochemistry. 23(25):6132–6137. doi: 10.1021/bi00320a036.
- Romero ZJ, Chen SH, Armstrong T, Wood EA, van Oijen A, Robinson A, Cox MM. 2020. Resolving Toxic DNA repair intermediates in every E. coli replication cycle: critical roles for RecG, Uup and RadD. Nucleic Acids Res. 48(15):8445–8460. doi: 10.1093/nar/gkaa579.
- Rowen L, Kornberg A. 1978. Primase DnaG protein of Escherichia coli - enzyme which starts DNA chains. J Biol Chem. 253(3):758–764.
- Roy R, Kozlov AG, Lohman TM, Ha T. 2007. Dynamic structural rearrangements between DNA binding modes of E. coli SSB protein. J Mol Biol. 369(5):1244–1257. doi: 10.1016/j.jmb.2007.03.079.
- Roy R, Kozlov AG, Lohman TM, Ha T. 2009. SSB protein diffusion on single-stranded DNA stimulates RecA filament formation. Nature. 461(7267):1092–1097. doi: 10.1038/nature08442.
- Rudolph CJ, Upton AL, Briggs GS, Lloyd RG. 2010. Is RecG a general guardian of the bacterial genome? DNA Repair (Amst). 9(3):210–223. doi: 10.1016/j.dnarep.2009.12.014.
- Rudolph CJ, Upton AL, Harris L, Lloyd RG. 2009. Pathological replication in cells lacking RecG DNA translocase. Mol Microbiol. 73(3):352–366. doi: 10.1111/j.1365-2958.2009.06773.x.
- Rudolph CJ, Upton AL, Lloyd RG. 2008. Maintaining replication fork integrity in UV-irradiated Escherichia coli cells. DNA Repair (Amst). 7(9):1589–1602. doi: 10.1016/j.dnarep.2008.06.012.
- Rudolph CJ, Upton AL, Stockum A, Nieduszynski CA, Lloyd RG. 2013. Avoiding chromosome pathology when replication forks collide. Nature. 500(7464):608–611. doi: 10.1038/nature12312.
- Ryzhikov M, Koroleva O, Postnov D, Tran A, Korolev S. 2011. Mechanism of RecO recruitment to DNA by single-stranded DNA binding protein. Nucleic Acids Res. 39(14):6305–6314. doi: 10.1093/nar/gkr199.
- Sakai A, Cox MM. 2009. RecFOR and RecOR as distinct RecA loading pathways. J Biol Chem. 284(5):3264–3272. doi: 10.1074/jbc.M807220200.
- Sandigursky M, Mendez F, Bases RE, Matsumoto T, Franklin WA. 1996. Protein-protein interactions between the Escherichia coli single-stranded DNA-binding protein and exonuclease I. Radiat Res. 145(5):619–623. doi: 10.2307/3579281.
- Sandler SJ. 1996. Overlapping functions for recF and priA in cell viability and UV-inducible SOS expression are distinguished by dnaC809 in Escherichia coli K-12. Mol Microbiol. 19(4):871–880. doi: 10.1046/j.1365-2958.1996.429959.x.
- Sandler SJ, Bonde NJ, Wood EA, Cox MM, Keck JL. 2024. The intrinsically disordered linker in the single-stranded DNA-binding protein influences DNA replication restart and recombination pathways in Escherichia coli K-12. J Bacteriol. In press.
- Sandler SJ, McCool JD, Do TT, Johansen RU. 2001. PriA mutations that affect PriA-PriC function during replication restart. Mol Microbiol. 41(3):697–704. doi: 10.1046/j.1365-2958.2001.02547.x.
- Sarov-Blat L, Livneh Z. 1998. The mutagenesis protein MucB interactions with single strand DNA binding protein and induces a major conformational change in its complex with single-stranded DNA. J Biol Chem. 273(10):5520–5527. doi: 10.1074/jbc.273.10.5520.
- Savvides SN, Raghunathan S, Fütterer K, Kozlov AG, Lohman TM, Waksman G. 2004. The C-terminal domain of full-length E. coli SSB is disordered even when bound to DNA. Protein Sci. 13(7):1942–1947. doi: 10.1110/ps.04661904.
- Schneider RJ, Wetmur JG. 1982. Kinetics of transfer of Escherichia coli single-strand deoxynucleic acid binding protein between single-stranded deoxynucleic acid molecules. Biochemistry. 21(4):608–615. doi: 10.1021/bi00533a002.
- Scotland MK, Heltzel JMH, Kath JE, Choi JS, Berdis AJ, Loparo JJ, Sutton MD. 2015. A genetic selection for dinB mutants reveals an interaction between DNA polymerase IV and the replicative polymerase that Is required for translesion synthesis. PLoS Genet. 11(9):e1005507. doi: 10.1371/journal.pgen.1005507.
- Scotland MK, Homiski C, Sutton MD. 2022. During translesion synthesis, Escherichia coli DinB89 (T120P) alters interactions of DinB (Pol IV) with Pol III subunit assemblies and SSB, but not with the β clamp. J Bacteriol. 204(4):e00611–00621. doi: 10.1128/jb.00611-21.
- Sengupta R, Pantel A, Cheng X, Shkel I, Peran I, Stenzoski N, Raleigh DP, Record MT. 2016. Positioning the intracellular salt potassium glutamate in the Hofmeister Series by chemical unfolding studies of NTL9. Biochemistry. 55(15):2251–2259. doi: 10.1021/acs.biochem.6b00173.
- Shereda RD, Bernstein DA, Keck JL. 2007. A central role for SSB in Escherichia coli RecQ DNA helicase function. J Biol Chem. 282(26):19247–19258. doi: 10.1074/jbc.M608011200.
- Shereda RD, Kozlov AG, Lohman TM, Cox MM, Keck JL. 2008. SSB as an organizer/mobilizer of genome maintenance complexes. Crit Rev Biochem Mol Biol. 43(5):289–318. doi: 10.1080/10409230802341296.
- Shereda RD, Reiter NJ, Butcher SE, Keck JL. 2009. Identification of the SSB binding site on E. coli RecQ reveals a conserved surface for binding SSB’s C-terminus. J Mol Biol. 386(3):612–625. doi: 10.1016/j.jmb.2008.12.065.
- Sherratt DJ, Søballe B, Barre F-X, Filipe S, Lau I, Massey T, Yates J. 2004. Recombination and chromosome segregation. Philos Trans R Soc Lond B Biol Sci. 359(1441):61–69. doi: 10.1098/rstb.2003.1365.
- Shibata T, Hishida T, Kubota Y, Han YW, Iwasaki H, Shinagawa H. 2005. Functional overlap between RecA and MgsA (RarA) in the rescue of stalled replication forks in Escherichia coli. Genes Cells. 10(3):181–191. doi: 10.1111/j.1365-2443.2005.00831.x.
- Shin Y, Brangwynne CP. 2017. Liquid phase condensation in cell physiology and disease. Science. 357(6357):eaaf4382. doi: 10.1126/science.aaf4382.
- Shinagawa H, Iwasaki H, Kato T, Nakata A. 1988. RecA protein-dependent cleavage of UmuD protein and SOS mutagenesis. Proc Natl Acad Sci U S A. 85(6):1806–1810. doi: 10.1073/pnas.85.6.1806.
- Shinn MK, Chaturvedi SK, Kozlov AG, Lohman TM. 2023. Allosteric effects of E. coli SSB and RecR proteins on RecO protein binding to DNA. Nucleic Acids Res. 51(5):2284–2297. doi: 10.1093/nar/gkad084.
- Shinn MK, Kozlov AG, Lohman TM. 2021. Allosteric effects of SSB C-terminal tail on assembly of E. coli RecOR proteins. Nucleic Acids Res. 49(4):1987–2004. doi: 10.1093/nar/gkaa1291.
- Shinn MK, Kozlov AG, Nguyen B, Bujalowski WM, Lohman TM. 2019. Are the intrinsically disordered linkers involved in SSB binding to accessory proteins? Nuc Acids Res. 47(16):8581–8594.
- Shlomai J, Kornberg A. 1980a. A prepriming DNA replication enzyme of Escherichia coli. I. Purification of protein n’: a sequence-specific, DNA-dependent ATPase. J Biol Chem. 255(14):6789–6793. doi: 10.1016/S0021-9258(18)43641-1.
- Shlomai J, Kornberg A. 1980b. A prepriming DNA replication enzyme of Escherichia coli. II. Actions of protein n’: a sequence-specific, DNA-dependent ATPase. J Biol Chem. 255(14):6794–6798. doi: 10.1016/S0021-9258(18)43642-3.
- Sigal N, Delius H, Kornberg T, Gefter ML, Alberts B. 1972. A DNA-unwinding protein isolated from Escherichia coli: its interaction with DNA and with DNA polymerases. Proc Natl Acad Sci U S A. 69(12):3537–3541. doi: 10.1073/pnas.69.12.3537.
- Singleton MR, Dillingham MS, Gaudier M, Kowalczykowski SC, Wigley DB. 2004. Crystal structure of RecBCD enzyme reveals a machine for processing DNA breaks. Nature. 432(7014):187–193. doi: 10.1038/nature02988.
- Slocum SL, Buss JA, Kimura Y, Bianco PR. 2007. Characterization of the ATPase activity of the Escherichia coli RecG protein reveals that the preferred cofactor is negatively supercoiled DNA. J Mol Biol. 367(3):647–664. doi: 10.1016/j.jmb.2007.01.007.
- Sokoloski JE, Kozlov AG, Galletto R, Lohman TM. 2016. Chemo-mechanical pushing of proteins along single-stranded DNA. Proc Natl Acad Sci U S A. 113(22):6194–6199. doi: 10.1073/pnas.1602878113.
- Spenkelink LM, Lewis JS, Jergic S, Xu ZQ, Robinson A, Dixon NE, van Oijen AM. 2019. Recycling of single-stranded DNA-binding protein by the bacterial replisome. Nucleic Acids Res. 47(8):4111–4123. doi: 10.1093/nar/gkz090.
- Stanage TH, Page AN, Cox MM. 2017. DNA flap creation by the RarA/MgsA protein of Escherichia coli. Nuc Acids Res. 45(5):2724–2735.
- Suksombat S, Khafizov R, Kozlov AG, Lohman TM, Chemla YR. 2015. Structural dynamics of E. coli single-stranded DNA binding protein reveal DNA wrapping and unwrapping pathways. Elife. 4:e08193. doi: 10.7554/eLife.08193.
- Sun ZQ, Tan HY, Bianco PR, Lyubchenko YL. 2015. Remodeling of RecG helicase at the DNA replication fork by SSB protein. Sci Rep. 5(1):9625. doi: 10.1038/srep09625.
- Suski C, Marians KJ. 2008. Resolution of converging replication forks by RecQ and topoisomerase III. Mol Cell. 30(6):779–789. doi: 10.1016/j.molcel.2008.04.020.
- Takahashi NK, Kusano K, Yokochi T, Kitamura Y, Yoshikura H, Kobayashi I. 1993. Genetic analysis of double-strand break repair in Escherichia coli. J Bacteriol. 175(16):5176–5185. doi: 10.1128/jb.175.16.5176-5185.1993.
- Tan HY, Bianco PR. 2021. SSB facilitates fork-substrate discrimination by the PriA DNA helicase. ACS Omega. 6(25):16324–16335. doi: 10.1021/acsomega.1c00722.
- Thrall ES, Piatt SC, Chang S, Loparo JJ. 2022. Replication stalling activates SSB for recruitment of DNA damage tolerance factors. Proc Natl Acad Sci U S A. 119(41):e2208875119. doi: 10.1073/pnas.2208875119.
- Tian LF, Kuang XL, Ding K, Gao HW, Tang Q, Yan XX, Xu WQ. 2023. Biochemical and structural analyses shed light on the mechanisms of RadD DNA bnding and Its ATPase from Escherichia coli. Int J Mol Sci. 24(1):12.
- Trewick SC, Henshaw TF, Hausinger RP, Lindahl T, Sedgwick B. 2002. Oxidative demethylation by Escherichia coli AlkB directly reverts DNA base damage. Nature. 419(6903):174–178. doi: 10.1038/nature00908.
- Umezu K, Chi NW, Kolodner RD. 1993. Biochemical interaction of the Escherichia coli RecF, RecO, and RecR proteins with RecA protein and single-stranded DNA binding protein. Proc Natl Acad Sci U S A. 90(9):3875–3879. doi: 10.1073/pnas.90.9.3875.
- Umezu K, Kolodner RD. 1994. Protein interactions in genetic recombination in Escherichia coli. Interactions involving RecO and RecR overcome the inhibition of RecA by single-stranded DNA-binding protein. J Biol Chem. 269(47):30005–30013. doi: 10.1016/S0021-9258(18)43981-6.
- Umezu K, Nakayama H. 1993. RecQ DNA helicase of Escherichia coli. Characterization of the helix-unwinding activity with emphasis on the effect of single-stranded DNA-binding protein. J Mol Biol. 230(4):1145–1150. doi: 10.1006/jmbi.1993.1231.
- Voloshin ON, Camerini-Otero RD. 2007. The DinG protein from Escherichia coli is a structure-specific helicase. J Biol Chem. 282(25):18437–18447. doi: 10.1074/jbc.M700376200.
- Waldman VM, Weiland E, Kozlov AG, Lohman TM. 2016. Is a fully wrapped SSB-DNA complex essential for Escherichia coli survival? Nucleic Acids Res. 44(9):4317–4329. doi: 10.1093/nar/gkw262.
- Wang TC, Smith KC. 1982. Effects of the ssb-1 and ssb-113 mutations on survival and DNA repair in UV-irradiated delta uvrB strains of Escherichia coli K-12. J Bacteriol. 151(1):186–192. doi: 10.1128/jb.151.1.186-192.1982.
- Wei TF, Bujalowski W, Lohman TM. 1992. Cooperative binding of polyamines induces the Escherichia coli single-strand binding protein-DNA binding mode transitions. Biochemistry. 31(26):6166–6174. doi: 10.1021/bi00141a029.
- Weiner JH, Bertsch LL, Kornberg A. 1975. The deoxyribonucleic acid unwinding protein of Escherichia coli. Properties and functions in replication. J Biol Chem. 250(6):1972–1980.
- Wendel BM, Cole JM, Courcelle CT, Courcelle J. 2018. SbcC-SbcD and Exol process convergent forks to complete chromosome replication. Proc Natl Acad Sci U S A. 115(2):349–354. doi: 10.1073/pnas.1715960114.
- Wessel SR, Marceau AH, Massoni SC, Zhou RB, Ha T, Sandler SJ, Keck JL. 2013. PriC-mediated DNA replication restart requires PriC complex formation with the single-stranded DNA-binding protein. J Biol Chem. 288(24):17569–17578. doi: 10.1074/jbc.M113.478156.
- Whitby MC, Lloyd RG. 1995. Branch migration of three-strand recombination intermediates by RecG, a possible pathway for securing exchanges initiated by 3’-tailed duplex DNA. Embo J. 14(14):3302–3310. doi: 10.1002/j.1460-2075.1995.tb07337.x.
- Whitby MC, Ryder L, Lloyd RG. 1993. Reverse branch migration of Holliday junctions by RecG protein: a new mechanism for resolution of intermediates in recombination and DNA repair. Cell. 75(2):341–350. doi: 10.1016/0092-8674(93)80075-p.
- Whitby MC, Vincent SD, Lloyd RG. 1994. Branch migration of Holliday junctions: identification of RecG protein as a junction specific DNA helicase. Embo J. 13(21):5220–5228. doi: 10.1002/j.1460-2075.1994.tb06853.x.
- Wickner S, Hurwitz J. 1975. Association of phiX174 DNA-dependent ATPase activity with an Escherichia coli protein, replication factor Y, required for in vitro synthesis of phiX174 DNA. Proc Natl Acad Sci U S A. 72(9):3342–3346. doi: 10.1073/pnas.72.9.3342.
- Windgassen TA, Leroux M, Satyshur KA, Sandler SJ, Keck JL. 2018. Structure-specific DNA replication-fork recognition directs helicase and replication restart activities of the PriA helicase. Proc Natl Acad Sci U S A. 115(39):E9075–E9084. doi: 10.1073/pnas.1809842115.
- Windgassen TA, Wessel SR, Bhattacharyya B, Keck JL. 2018. Mechanisms of bacterial DNA replication restart. Nucleic Acids Res. 46(2):504–519. doi: 10.1093/nar/gkx1203.
- Witte G, Urbanke C, Curth U. 2003. DNA polymerase III chi subunit ties single-stranded DNA binding protein to the bacterial replication machinery. Nucleic Acids Res. 31(15):4434–4440. doi: 10.1093/nar/gkg498.
- Wolak C, Jun H, Soubry N, Sandler SJ, Reyes-Lamothe R, Keck JL. 2020. Interaction with single-stranded DNA-binding protein localizes ribonuclease HI to DNA replication forks and facilitates R-loop removal. Mol Microbiol. 114(3):495–509. doi: 10.1111/mmi.14529.
- Yamaguchi H, Hanada K, Asami Y, Kato J, Ikeda H. 2000. Control of genetic stability in Escherichia coli: the SbcB 3′-5′ exonuclease suppresses illegitimate recombination promoted by the RecE 5′-3′ exonuclease. Genes Cells. 5(2):101–109. doi: 10.1046/j.1365-2443.2000.00309.x.
- Yu C, Tan HY, Choi M, Stanenas AJ, Byrd AK, Raney KD, Cohan CS, Bianco PR. 2016. SSB binds to the RecG and PriA helicases in vivo in the absence of DNA. Genes Cells. 21(2):163–184. doi: 10.1111/gtc.12334.
- Yuzhakov A, Kelman Z, O’Donnell M. 1999. Trading places on DNA–a three-point switch underlies primer handoff from primase to the replicative DNA polymerase. Cell. 96(1):153–163. doi: 10.1016/s0092-8674(00)80968-x.
- Zhao TY, Liu Y, Wang ZL, He RY, Zhang JX, Xu F, Lei M, Deci MB, Nguyen J, Bianco PR. 2019. Super-resolution imaging reveals changes in Escherichia coli SSB localization in response to DNA damage. Genes Cells. 24(12):814–826. doi: 10.1111/gtc.12729.
- Zhou RB, Kozlov AG, Roy R, Zhang JC, Korolev S, Lohman TM, Ha T. 2011. SSB functions as a sliding platform that migrates on DNA via reptation. Cell. 146(2):222–232. doi: 10.1016/j.cell.2011.06.036.