Abstract
A cost-effective approach for efficient organic micropollutants (OMPs) removal is to optimize existing infrastructure at drinking water treatment plants. A promising option is rapid sand filtration (RSF), as OMPs removal has been observed in this treatment technology. However, the mechanisms and pathways involved are not fully understood and strategies to optimize removal have yet to be thoroughly explored. Therefore, this article firstly described basic RSF functions that can support OMPs removal. OMPs can be removed by chemical and biological Mn/Fe oxides or degraded co-metabolically by ammonia oxidizing bacteria and methane oxidizing bacteria. In addition, heterotrophic bacteria can metabolically transform OMPs and their transformation products. Then, we reviewed current literatures described OMPs removal in RSF, showing biodegradation can contribute significantly to OMPs removal. Thereafter, we presented strategies to improve OMPs biodegradation, including bioaugmentation, optimizing hydraulic conditions by adjusting contact time and backwashing intensity, and adding biocarriers to retain biomass during rapid flow rates. Finally, we provided recommendations for further research towards optimizing and maintaining OMPs removal in RSF for safe drinking water production. This review therefore gives a critical evaluation of RSF-based technologies for OMPs removal from drinking water and provides recommendations for further improving OMPs removal in RSF.
Graphical abstract
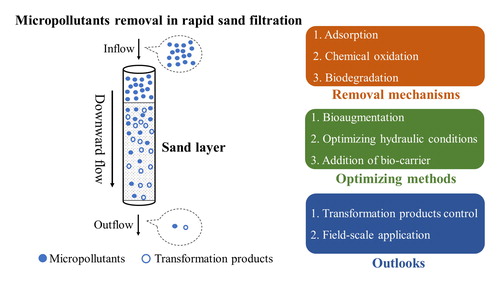
1. Introduction
In recent years, there is increasing concern about the presence of organic micropollutants (OMPs) in source waters and their effects on drinking water quality (Jones et al., Citation2005; Zuehlke et al., Citation2007). Surface water and groundwater are the main water sources for producing drinking water. OMPs, such as pesticides, pharmaceuticals and other industrially and naturally produced organic compounds, have been found in environmental waters used for drinking water production (Kolpin et al., Citation2002; Onesios et al., Citation2009; Schulman et al., Citation2002). For example, use of pesticides has resulted in the occurrence of residual pesticides in the source water of drinking water treatment plants (DWTPs) (Swartjes & van der Aa, Citation2020; Vandermaesen et al., Citation2019). Similarly, pharmaceutical residues are present in sewage treatment effluents, with concentrations in source water used for drinking water production sometimes in the range of µg/L level (Zuehlke et al., Citation2007). Other industrial compounds such as Bisphenol A (Cooper et al., Citation2011; Howdeshell et al., Citation1999), as well as naturally produced organic compounds such as microcystin toxins produced by cyanobacteria can also be found in source water (Ho et al., Citation2006; Jian et al., Citation2019; Jochimsen et al., Citation1998). The complex mixtures normally observed in surface water originate from sewage effluents from wastewater treatment plants (WWTPs) (Larsen et al., Citation2004; Onesios et al., Citation2009; Ternes et al., Citation2002), while groundwater is mainly contaminated by agricultural residues () (Ahmad et al., Citation2019; Benner et al., Citation2013). Many of the aforementioned compounds and classes of compounds are regulated in a variety of DW guidelines, for instance, the European Union generally define the maximal concentration of pesticides allowed in drinking water is 0.1 µg/L (Council Directives 98/83/EC and 2006/118/EC) (Albers et al., Citation2015; Vandermaesen et al., Citation2019); the World Health Organization has suggested its maximal concentration of Microcystin toxins is up to 1.0 µg/L (WHO, Citation1997). Thus, OMPs removal from water sources should be actively pursued at DWTPs to produce high quality potable water and protect public health.
The low concentrations of OMPs in the source water, from several ng/L to μg/L, make treatment especially challenging (Luo et al., Citation2014). Techniques such as (advanced) oxidation (Benner & Ternes, Citation2009; Lee & Gunten, Citation2010; Ormad et al., Citation2008), activated carbon (Kim & Kang, Citation2008; Piai et al., Citation2019) and membrane filtration (Cartinella et al., Citation2006; Košutić et al., Citation2005; Sarkar et al., Citation2007) have been installed at DWTPs around the globe to increase OMPs removal. While effective at removing a range of OMPs, these oxidizing processes often produce toxic transformation products (TPs) (Benner & Ternes, Citation2009; Dodd et al., Citation2010; Fatta-Kassinos et al., Citation2011; Mcdowell et al., Citation2005; Müller et al., Citation2012; Pereira et al., Citation2011). Membrane-based technologies generate brine streams which have to be treated prior to discharge (Nicolaisen, Citation2003; Sarkar et al., Citation2007). Overall, these add-on technologies have high investment, operational and energy costs.
Rapid sand filtration (RSF) is applied worldwide at DWTPs to produce healthy and safe drinking water (Zearley & Summers, Citation2012). RSFs are used for particle filtration (Craft, Citation1966), physico-chemical and biological oxidation of Fe(II), Mn(II) (Cakmakci et al., Citation2008; Tekerlekopoulou et al., Citation2013), ammonium and methane oxidation (de Vet et al., Citation2011; Hedegaard et al., Citation2018; Lee et al., Citation2014; Lopato et al., Citation2013), and As(III) removal (Gude et al., Citation2016, Citation2018). Recent studies have shown that OMPs, such as pharmaceuticals (Richter et al., Citation2008; Zearley & Summers, Citation2012) and pesticides (Feld et al., Citation2016; Hedegaard & Albrechtsen, Citation2014; Vandermaesen et al., Citation2019), can be biologically removed from drinking water in RSF. Thus, when properly optimized and operated, existing RSF can be used for the removal of OMPs at DWTPs. However, this requires a thorough understanding of the processes that contribute to OMPs removal and investigations into strategies to enhance these processes in existing RSF.
In this paper, we first introduced the primary functions of RSF in drinking water treatment processes. Thereafter we linked these basic functions to the different mechanisms by which OMPs could be removed in RSF (Section 2). In addition, we summarized and assessed the performances of RSF-based technologies for OMPs removal in drinking water production, highlighting the importance of biodegradation of OMPs by autochthonous communities (Section 3). In Section 4, we discussed several strategies for improving OMPs biodegradation via bioaugmentation, optimizing hydraulic schemes and adding bio-carriers. Finally, we evaluated the limitations of the technology and discuss the challenges during its application (Section 5). Overall, this review therefore gives a critical assessment of the current potential for OMP removal in RSF and describes future innovations necessary to improve RSF-based technologies for OMPs removal from drinking water.
2. RSF functions and OMPs removal mechanisms
2.1. Primary functions of RSF
RSF is an important treatment step at DWTPs for drinking water production, that involves physico-chemical and biological removal processes for different target contaminants (Bai et al., Citation2016). RSF typically consists of a sand bed with a height of 1.5–2.5 m, and is operated with a downward filtration velocity of 3–8 m/h (de Moel et al., Citation2006). In addition, the filters need to be backwashed periodically to prevent clogging by the retained solids, such as precipitated metal oxides and excess biomass (Albers et al., Citation2015).
The aerated groundwater or pretreated surface water are supplied to RSF, where O2 promotes the oxidative removal of dissolved Fe(II), Mn(II), ammonium and residual methane (de Moel et al., Citation2006), but also the sand bed can remove organic or/and inorganic matters (Bar-Zeev et al., Citation2012). Fe(II) may be precipitated into Fe oxides (FeOx) by either homogeneous oxidation in supernatant water layer, or by heterogeneous and biological oxidation in the filter bed, depending on pH values, O2 concentrations and filter operation (van Beek et al., Citation2012; Vries et al., Citation2017). Homogeneous Mn(II) oxidation is slow and Mn(II) mainly transfers into Mn oxides (MnOx) through biological oxidation in fresh filter bed, whereafter by autocatalytic oxidation when MnOx are accumulating on the sand grains (Bruins et al., Citation2015). Ammonium and methane are also biologically oxidized in RSF, executed by nitrifying bacteria (de Vet et al., Citation2011; Lee et al., Citation2014; Tatari et al., Citation2013) and methanotrophic bacteria (Benner et al., Citation2013; Hedegaard et al., Citation2018), respectively. In addition, with a functional biofilm developed by heterotrophic microorganisms, the dissolved and particular organic carbon organic carbon can be biologically removed within the filter bed (Bar-Zeev et al., Citation2012). Therefore, the active autochthonous bacterial communities in RSF play important roles in the removal of multiple pollutants from the source water.
2.2. OMPs removal mechanisms in RSF
In addition to these basic functions discussed in section 2.1, studies indicate that RSF has the potential to remove OMPs (Hedegaard & Albrechtsen, Citation2014; Zearley & Summers, Citation2012). The set of mechanisms for OMPs removal are to a large extent intertwined with the RSF treatment functions. Therefore, in this section, we give a comprehensive review of OMPs removal mechanisms based on the RSF primary functions. OMPs removal processes that are reported in the literature are (a combination) of the following processes (): (1) adsorption onto sand material, (2) oxidation by iron/manganese oxides, (3) biodegradation by autotrophic bacteria, and (4) biodegradation by heterotrophic bacteria.
Figure 2. Overview of OMPs removal pathways in RSF. (a) Adsorption of OMPs onto sand granules. (b) Oxidation and adsorption of OMPs by iron/manganese oxides. (c) Biodegradation of OMPs by autotrophic bacteria (nitrifying and/or methanotrophic bacteria). (d) Biodegradation of OMPs by heterotrophic bacteria.
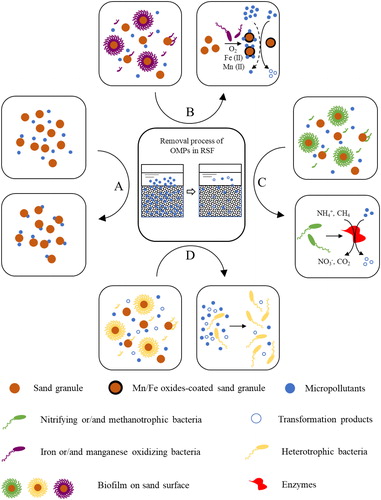
2.2.1. OMPs adsorption
Adsorption and retention of suspended particles (Ives, Citation1970), heavy metals (Liu et al., Citation2009) and organic matters (Ding et al., Citation2010) onto the surface of filter material contributes significantly to RSF performance. First, the surface of particles is often charged when placed in contact with water, resulting in electrostatic and van der Waals forces (O'Melia & Crapps, Citation1964). For example, sands particles can be charged by ionization of molecules, imperfections in the crystal lattice, direct chemical action with specific ions in the solution (O'Melia & Crapps, Citation1964), or the FeOx/MnOx accumulated on sand granules at specific pH values (Vries et al., Citation2017). In addition, hydrophobic interaction between the sand granule surface and the adsorbing molecule can be relevant for adsorption of organic matter (Ding et al., Citation2010).
OMPs can be adsorbed onto sand granules in RSF, with removal efficiencies mainly dependent on the retention time. Previous studies reported the effective removal of four compounds (galaxolide, tonalide, celestolide, and triclosan) by adsorption in RSF (Paredes et al., Citation2016). The removal efficiencies decreased more than 10% with shortening contact time from 35 min to 17 min (Paredes et al., Citation2016), indicating the importance of contact time for effective adsorption. In real RSFs with biological activity, it is challenging to separate OMPs removal by adsorption from biodegradation. Laboratory RSFs working with 14C-labelled herbicide mecoprop (MCPP) (Hedegaard et al., Citation2014) or with autoclaved columns treating microcystin (Ho et al., Citation2006) have shown that biodegradation is the dominating removal process. However, these studies do show that 10%–15% of the OMPs were removed by adsorption.
The adsorption results indicate the potential for improved filter materials to improve OMPs absorption. For example, it has been suggested that surface modification with cationic surfactants or polymers can improve the adsorbents’ capacity for organic compounds (Ding et al., Citation2010). Iron oxide-coated sand modified with hexadecyltrimethyl ammonium showed better natural organic matter adsorption than unmodified ones in both microcosm and column experiments over a wide pH range (Ding et al., Citation2010). These findings indicate that future research should focus on techniques to modify sand for improved OMPs removal.
2.2.2. OMPs removal by iron/manganese oxides
A key function of RSFs treating groundwater is the removal of Fe(II) and Mn(II), generally by a combination of chemical and biological oxidation (van Beek et al., Citation2012; Vries et al., Citation2017). The first treatment step of groundwater is usually aeration, where Fe(II) is oxidized into FeOx. Also, in this step, CO2 is removed, increasing the pH and the (chemical) oxidation rate of iron. The iron oxides are filtered out in the top layer of RSF. When aeration is limited, biological oxidation of Fe(II) can become more important. A number of iron oxidizing bacteria have been reported to participate in the biological oxidation of iron, such as: Leptothrix ochracea, Gallionella ferruginea, Toxothrix trichogenes, Thiobacillus ferrooxidans, and Crenothrix (Kirby et al., Citation1999; Michalakos et al., Citation1997; Rentz et al., Citation2007). Depending on groundwater composition, Mn(II) oxidation can also occur in RSF. As Mn(II) oxidation is a biological process, that has a slower oxidation rate than Fe(II) oxidation. Several manganese oxidizing bacteria (MnOB) have been identified on filter materials and in the water phase, including Pseudomonas sp., Streptomyces sp., and Leptothrix sp. (Bruins et al., Citation2014; Hu et al., Citation2020). The Mn and Fe oxides (MnOx and FeOx) formed and retained in RSF are able to remove OMPs by adsorption (Forrez et al., Citation2011) or by catalyzing chemical oxidation (Jian et al., Citation2019; Manoli et al., Citation2017) ().
MnOx and FeOx can be used as adsorbents, especially when present as nanoparticles which have a large surface area for OMPs adsorption (Tebo et al., Citation2004). Previous studies showed that MnO2 can adsorb pharmaceuticals and other pollutants including antibacterial agents like sulfonamides and tetracycline, as well as endocrine disruptors (Bernard et al., Citation1997; Remucal & Ginder-Vogel, Citation2014). In addition, Fe (III) can also adsorb pharmaceuticals effectively (Feitosa-Felizzola et al., Citation2009). For example, two human-used antibacterial agents, clarithromycin and roxithromycin, are strongly adsorbed (>90%) to Fe(III) (ferrihydrite). This is probably due to the fact that the macrolide antibacterial agents can be complexed onto the surface of Fe(III) (Feitosa-Felizzola et al., Citation2009). Thus, FeOx/MnOx-coated sand material can improve adsorption of OMPs in RSF.
Compared to synthetic MnOx, microbially produced MnOx (bioMnOx), as is commonly found in RSF treatments, has a unique structure, yielding a variety of advantages for OMPs oxidation (Liu et al., Citation2016; Tu et al., Citation2014). Firstly, BioMnOx are reactive in oxidation under neutral pH (∼7), instead of the acidic conditions required for the electrochemically produced MnOx (Bellusci et al., Citation2014; Remucal & Ginder-Vogel, Citation2014). Additionally, the bacteria can bind the intermediate Mn(III) compounds via ligands, thus significantly enhancing the oxidative power of a Mn-bacteria complex (Meerburg et al., Citation2012). Previous studies show that bioMnOx can efficiently remove pharmaceuticals (Forrez et al., Citation2011; Furgal et al., Citation2015) and other pollutants (Meng et al., Citation2009; Tom et al., Citation2009). Zhang et al., and (Citation2015) found that 80%–90% of diclofenac and sulfamethoxazole can be removed via oxidation by bioMnOx in biofilters. Moreover, it has also been suggested that OMPs and Mn(II) can be biologically co-removed in bioaugmented RSF (Jian et al., Citation2019). The growth of MnOB strain (Pseudomonas sp. QJX-1) can enhance the biogenic Mn(II) oxidation, and the formed bioMnOx are able to oxidize 56% microcystin-LR with the initial concentration of 3.5 µg/L within 5 min (Jian et al., Citation2019).
FeOx can also be generated by microbial activity, resulting in bioFeOx (Tom et al., Citation2009). However, the role of bioFeOx in OMPs removal in RSF has yet to be elucidated. The large surface area of bioFeOx seems to suggest that these compounds could effectively adsorb OMPs (Forrez et al., Citation2011; Tom et al., Citation2009). Future research should focus on understanding the potential of bioFeOx for OMPs removal in RSF.
2.2.3. Co-metabolic OMPs biodegradation by autotrophic bacteria
In raw groundwater, the concentrations of ammonium and methane are at hundreds µg/L, even several mg/L (Papadopoulou et al., Citation2019; Tatari et al., Citation2013). These compounds are typically removed by a combination of aeration and removal by autotrophic nitrifying and methane oxidizing bacteria (Hu et al., Citation2020; Papadopoulou et al., Citation2019). In addition to ammonium and methane removal, these autotrophic organisms also show OMPs biotransformation capacity (Batt et al., Citation2006; Papadopoulou et al., Citation2019). This co-metabolic conversion results in OMPs removal in RSF treating groundwater.
2.2.3.1. Biodegradation by nitrifying bacteria
Biological RSF is commonly used to remove ammonium from water sources by nitrification (de Vet et al., Citation2011; Kors et al., Citation1998; van der Aa et al., Citation2002). Nitrification includes two biological processes, which are conducted by ammonia oxidizing bacteria (AOB) and nitrite oxidizing bacteria, respectively (Niu et al., Citation2013). The ammonia monooxygenase enzyme has a broad substrate specificity, and not only catalyzes the oxidation reaction of ammonium, but also that of such compounds as alkanes, alkenes, aromatic and alicyclic hydrocarbons, halogenated hydrocarbons, and sulfonated hydrocarbons (Arciero et al., Citation1989; Hyman et al., Citation1988; Im & Semrau, Citation2011). It has been shown that nitrifying bacteria are capable of co-metabolizing various OMPs (Men et al., Citation2016; Roh et al., Citation2009; Shi et al., Citation2004; Sun et al., Citation2012).
Previous studies show that ammonium and OMPs can be co-removed by nitrifying bacteria in biological filters. Rattier et al., and (Citation2014) identified the role of nitrification in OMPs removal, assessing the contribution of nitrifying bacteria to OMPs biodegradation during RSF. Column-scale filters treating 33 OMPs showed removal efficiencies above 50% for 11 compounds. Moreover, the addition of the nitrification inhibiter, allylthiourea, resulted in a notable decrease in some OMPs removal (Rattier et al., Citation2014). For example, with the inhibiter, the removal of some compounds (i.e., ketoprofen, gemfibrozil, furosemide, acetaminophen, caffeine) was obviously limited and the removal of lincomycin was even stopped (Rattier et al., Citation2014). This confirmed the important role of nitrifying microorganisms in biotransformation of OMPs.
2.2.3.2. Biodegradation by methanotrophic bacteria
Removal of methane from treated groundwater in RSF is performed by methane oxidizing bacteria (MOB) (Benner et al., Citation2013; Hedegaard et al., Citation2018). Similar to the ammonia monooxygenase enzyme, methane monooxygenase enzymes also can oxidize many different OMPs (Benner et al., Citation2013; Brusseau et al., Citation1990; Colby et al., Citation1977; Sullivan et al., Citation1998).
Recent work shows the ability of MOB to co-metabolize OMPs in RSF (Benner et al., Citation2015). For instance, Hedegaard et al. (Citation2018) find that bentazone can be co-metabolically transformed into hydroxy-bentazone in a methanotrophic culture enriched from a groundwater-fed RSF in DWTP. The biodegradation efficiency of bentazone increases from 31% to 53% and formation of hydroxylated bentazone is stimulated in the presence of methane (Hedegaard et al., Citation2018). On the other hand, bentazone oxidation is reduced when the methane oxidation is inhibited by acetylene (Hedegaard et al., Citation2018). However, the investigators suggest that there are still many other bacteria in the complex community that can be responsible for bentazone oxidation (Hedegaard et al., Citation2018). Thus, the pure culture of methanotrophs should be studied further as a full enzymatic evidence of bentazone removal by methane monooxygenase enzymes (Hedegaard et al., Citation2018). In addition, the contributions of diverse microbial metabolisms on different OMPs should be investigated in future research to reveal the full biodegradation potential of RSF.
2.2.4. OMPs biodegradation by heterotrophic bacteria
In contrast to co-metabolic conversion by autotrophs, heterotrophic bacteria can use organic compounds including OMPs as primary substrates for cell growth (Tran et al., Citation2013) (). However, the low concentrations of OMPs in RSF systems typically are insufficient to support the growth of heterotrophic bacteria. Therefore, heterotrophs still need to utilize other carbon source for their metabolisms. For example, Aminobacter sp. MSH1, a 2,6-dichlorobenzamide (BAM)-degrading organism, is suggested to mainly feed on assimilable organic carbon (AOC) while biodegrading BAM (Horemans et al., Citation2017). This research showed that AOC concentrations limited MSH1 biodegradation activity, indicating that AOC addition may be required to support the growth of MSH1 (Horemans et al., Citation2017). Other studies also showed that a higher AOC concentration can improve the removal efficiencies of two microbial metabolites (geosmin and 2-methylisoborneol (MIB)), which cause the earthy and/or musty odors in potable water, in RSF (Elhadi et al., Citation2006). At high AOC concentrations of 280 µg/L, 42% of geosmin and 10% of MIB were removed, while only 14% and 1%, respectively was removed at low AOC level 28 µg/L (Elhadi et al., Citation2006). Higher AOC concentrations correlated with higher biomass concentrations, indicating the importance of AOC for biological activity and thus also OMP biodegradation (Elhadi et al., Citation2006).
Research indicates that AOC is not only important for biomass growth in RSF, but can also select for OMPs biodegradation capacity. Studies on the mineralization of BAM and its metabolite, 2,6-dichlorobenzoic acid (2,6-DCBA) in RSF units showed that 2,6-DCBA mineralization correlated with especially aromatic compounds in inflow water (Vandermaesen et al., Citation2016). They observed that the heterotrophic organisms metabolizing these AOC substrates are able to produce broad substrate enzymes that degrade similar compounds like 2,6-DCBA (Fulthorpe et al., Citation1996; Vandermaesen et al., Citation2016). Overall, these results thus show the importance of AOC in selecting for and supporting OMPs biodegradation capacity in RSF. Future research should aim at developing effective AOC-based biostimulation technologies to further exploit RSF biodegradation capacity.
3. Evaluation and discussion on OMPs removal in existing RSF system
The above removal mechanisms have been shown to be important for OMPs removal in RSF. However, a review of recent literatures show that biodegradation is the most significant removal pathway in real RSF system (). In addition, the potential of OMPs biodegradation still needs to be further exploited and improved, especially in field RSF. Here we evaluate the positive and negative aspects of using RSF-based system for OMPs removal, highlighting the importance of biodegradation for OMPs removal but also the challenges of future optimization and application.
Table 1. OMPs removal by rapid sand filtration-based technology for drinking water production.
Effective elimination of certain OMPs including pharmaceuticals and pesticides has been found during RSF-based treatment processes (). For example, in column-scale RSF, the biodegradation efficiencies of phenazone, propyphenazone and were 94% and 92%, respectively (Zuehlke et al., Citation2007). Similarly, Hedegaard et al., and (Citation2019) showed that 92% of bentazone, a widely used herbicide and often a recalcitrant groundwater contaminant, was degraded within 13 days in RSF. Moreover, Hedegaard et al. (Citation2019) suggested that various microbial groups were involved in the biodegradation: methanotrophs transformed bentazone to various hydroxylated TPs; thereafter, a diverse community was involved in the full degradation of TPs.
However, RSF performance for OMP removal is variable. Papadopoulou et al. (Citation2019) studied the biodegradation of 10 pesticides by MOB in column-scale RSF and found that removal efficiencies for diuron, isoproturon, linuron, 4-chloro-2-methylphenoxy acetic acid and MCPP were between 6.8% and 11.3%; the rest of the compounds (BAM, bromoxynil, cholrotoluron, and ioxynil) were not removed (Papadopoulou et al., Citation2019). The different elimination behaviors of OMPs in RSF were also verified by another microcosm experiment, where 42%–85% of MCPP, 15%–35% of bentazone, 7%–14% of glyphosate an 1%–3% p-nitrophenol were removed (Hedegaard & Albrechtsen, Citation2014). In addition, different OMPs show the diverse biodegradability in RSF. A steady removal of 19 compounds, including pharmaceuticals, pesticides and personal care products, has been achieved in a long-term operated RSF (Zearley & Summers, Citation2012). However, 13 compounds were recalcitrant to biodegradation (less than 15% removal), 7 compounds had slow biodegradation rates (15%–50% removal) and only 12 compounds had high biodegradation rates (greater than 50% removal) (Zearley & Summers, Citation2012). In unraveling the removal of OMPs in RSF, it is important to note that compound class and structure are not necessarily determining factors. For example, Richter et al. (Citation2008) operated a column-scale RSF to treat contaminated groundwater with three sulfonamides (para-toluenesulfonamide [p-TSA], ortho-toluenesulfonamide [o-TSA], and benzenesulfonamide [BSA]) and found that only p-TSA can be effectively removed by 93%. This indicates that compounds in the same class can also have different biodegradation efficiencies in RSF.
In addition, several studies have revealed biodegradation of OMPs in field-scale RSF. Feld et al. (Citation2016) set up a pilot RSF in a contaminated landfill-site to treat groundwater containing the three phenoxypropionate herbicides. After three months, OMPs degraders had successfully established, resulting in biodegradation of 17% of (RS)-2-(2,4-dichlorophenoxy)propanoic acid, 31% of MCPP, and 22% of 4-chlorophenoxypropanoic acid (4-CPP, the associated catabolite) (Feld et al., Citation2016). They also suggested that abiotic processes, such as adsorption and precipitation did not contribute to herbicide removal in RSF (Feld et al., Citation2016). At a groundwater-based DWTP, investigators used RSF to treat aerated groundwater (Hedegaard et al., Citation2014). In RSF, MCPP concentration decreased from 0.037 µg/L to below the detection limit of 0.01 µg/L in 63 min. Removal was by a combination of minor adsorption of MCPP in the top layer and microbial removal throughout the filter (Hedegaard et al., Citation2014).
While the above, column-scale and field-scale investigations show the potential for OMPs biodegradation in RSF, these studies insufficiently cover the full breadth of compounds and field conditions. Removal of OMPs in RSF has mainly been shown for pesticides (), as groundwater was often used as water source (). Only two pharmaceuticals (phenazone and sulfonamides) and their TPs were comprehensively studied in previous investigations (Richter et al., Citation2008; Zuehlke et al., Citation2007). Furthermore, these previous studies mainly focused on parent compounds (). However, OMPs biodegradation produces TPs with concentrations similar to parent compounds (de Jongh et al., Citation2012). Finally, most studies to date are performed on column-scale RSF setups (). Only phenazone, phenoxypropionate herbicides and their metabolites are treated in field-scale RSF ().
Figure 3. Overview of the categories of OMPs previously studied. (a) Compound type, (b) belonging to parent compound or transformation product (c) research scale. The detailed information of target OMPs is given in the supplementary information (Table S1).
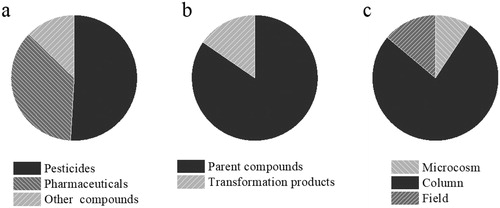
On the other hand, many researchers only focus on OMPs removal efficiency instead of understanding the participated microbial populations in OMPs biodegradation. Although above studies show that biodegradation is the most important mechanism of OMPs removal (), only heterotrophic degraders of geosmin, MIB, BAM and MCPP have been defined in previous investigations (Albers et al., Citation2015; Elhadi et al., Citation2006; Feld et al., Citation2016) (). In addition, Hedegaard et al. (Citation2018) and Papadopoulou et al. (Citation2019) find that methanotrophic bacteria can remove OMPs from groundwater, while few investigations focus on the contribution of nitrifying bacteria to OMPs biodegradation in RSF for drinking water production. Thus, future research should include a broader set of OMPs and TPs, especially focusing on field-scale operations, as well as the detailed information of involved microbial populations in OMPs biodegradation in order to fully understand the potential of RSF to biodegrade OMPs.
4. Technical solutions for improving OMPs biodegradation
Based on the aforementioned research, it is clear that RSF have the potential to remove OMPs. The involved removal mechanisms encompass a complex mixture of physical-chemical and biological processes. Furthermore, shows that biodegradation is the most important removal mechanism in RSF. In order to further exploit the potential of RSF for OMP biodegradation, different technical solutions have been proposed in this section ().
4.1. Bioaugmentation
Bioaugmentation is an approach whereby microbial consortia enriched for the capacity to (partially) degrade or mineralize OMPs are introduced into an existing treatment system (Semrany et al., Citation2012). Research has shown the potential of this approach for improving OMPs biodegradation in RSFs (Zearley & Summers, Citation2012). For example, bioaugmentation of pilot RSF with a BAM-degrading culture resulted in up to 75% removal of BAM, resulting in concentrations below drinking water regulations of 0.1 µg/L (Albers et al., Citation2015) (). However, improvements in biodegradation due to bioaugmentation lasted less than 7 days, mainly due to loss of the inoculated bacteria. According to previous studies, the success of bioaugmentation strategies depends on the ability of the strain to integrate into the existing microbial community (Zearley & Summers, Citation2012). Therefore, bioaugmentation strategies must focus on consortia adapted to oligotrophic environments found in RSF (Gözdereliler et al., Citation2013). Moreover, bioaugmentation in RSF is challenged by washout of inoculated bacteria from the filters during general backwashing (Feakin et al., Citation1995; Horemans et al., Citation2017). Thus, future research into bioaugmentation should focus on selecting consortia able to efficiently invade and be retained in RSF.
To this end, enrichment of relevant microbial consortia for bioaugmentation requires both inoculum with biodegradation capacity as well as enrichment conditions similar to those in RSF. Often biodegrading consortia or strains are selected from polluted environments, like agricultural soils, industrial wastewater, surface water and WWTP (Feakin et al., Citation1995). For example, Sørensen et al., (Citation2007) isolated the BAM-degrading bacterium (Aminobacter sp. MSH1) from the pesticides-polluted agriculture soil and enriched them in mineral salt medium with 25–50 mg/L BAM. Thereafter, the low inlet BAM concentration (0.2 µg/L) could not sufficiently support MSH1 growth in groundwater-feeding RSF, leading to an ineffective bioaugmentation (Albers et al., Citation2015). These results show the importance of cultivation and isolation conditions for the subsequent success of bioaugmentation. We suggest future research should examine how to improve isolation of bacterial strains cultivated with the target OMPs under environmentally relevant conditions so they can survive in the oligotrophic environment in the RSF.
In addition to competition and starvation in RSF, it has been found that bioaugmentation failure can also be attributed to protozoan predation (Ellegaard-Jensen et al., Citation2016). Following inoculation of specific BAM-degrading MSH1, investigators observed a rapid increase in protozoan cell density. Albers et al. (Citation2015) suggest that protozoan predation may contribute significantly to the loss of MSH1-bacteria (2 × 108–2 × 109 bacterial cells/cm3 within the first 14 days of operation). Thus, it is essential to control biomass loss from backwashing and protozoan predation, especially at the beginning of bioaugmentation. In addition, it has been reported that the porous bio-carrier can immobilize bacterial cells and protect them from protozoa predation if the size of pores is less than 6 µm (Wright et al., Citation1995). Thus, additional porous carriers can be another method to protect bacteria from protozoan predation.
4.2. Optimizing hydraulic conditions
It has been suggested that OMPs biodegradation efficiency is hampered by the poor retention of microbial communities (Samuelsen et al., Citation2017). RSF hydraulics including empty bed contact time (EBCT) and backwashing are optimized to support RSF primary functions. However, hydraulics obviously also influence retention and feeding of microbial communities, which in turn determines biodegradation activity (Paredes et al., Citation2016) and the retention of the biomass on the sand surface (Albers et al., Citation2015). In order to support biodegradation of OMPs in RSF, we propose a variety of strategies to optimize hydraulic conditions for more effective OMPs.
4.2.1. Empty bed contact time
EBCT is an expression of the time during which a water to be treated is in contact with sand material in RSF. EBCT determines both the loading rate of nutrients as well as the contact time between microbial communities and OMPs. The shorter EBCT results in higher overall biological activity due to the increase in nutrient loading rates, while simultaneously limits the contact time in which biotransformation can occur (Paredes et al., Citation2016).
The result of changing EBCT depends on the biodegradability of OMPs. For OMPs with low biodegradation rates, shorter EBCT results in lower removal efficiencies (Paredes et al., Citation2016). The eliminations of these compounds are kinetically limited, and therefore sufficient contact time is required for biodegradation to occur. On the contrary, for more readily biodegradable compounds, lower EBCT improves their removal rates, as the increased biological activity at higher nutrient loading rates compensates for the reduction in contact time. To determine the biodegradability of target compounds, biodegradation times can be firstly assessed in microcosm tests. Based on this, suitable EBCT can be further adjusted to ensure effective OMPs removal in biological RSF. If multiple target compounds with largely different required EBCT, longer EBCT should be chosen in RSF. In this case, additional nutrients can be dosed to ensure sufficient feeding for microbial communities.
4.2.2. Backwashing
To prevent clogging by precipitated metal oxides and excess biomass, RSF need to be backwashed regularly, typically by reversing the water flow and flushing with air. During this process, attached bacteria may be washed out (Walker et al., Citation2005). Albers et al. (Citation2015) find extensive loss of bioaugmented bacteria and a decrease in the removal rate of pesticides in RSF following backwashing. In contrast, established biofilms are enclosed in extracellular polymeric substances (EPS) which makes biofilms resistant toward backwashing (Costerton et al., Citation1995; Verhagen et al., Citation2011). Moreover, bioaugmentation in RSF is challenged by washout of inoculated bacteria from the filters during general backwashing (Feakin et al., Citation1995; Horemans et al., Citation2017). Hence, it is necessary to control the backwashing intensity, including velocity and frequency in the startup of filters as well as the beginning period of bioaugmentation, to encourage inoculated communities to form biofilms onto the sand granules in RSF.
4.3. Addition of bio-carrier
As above discussion in section 4.1, bioaugmentation failure is often due to insufficient retention of biomass, low level of nutrient and protozoan predation. To resolve this problem, a novel bio-carrier has been designed to protect enriched microorganisms from immediate loss (Horemans et al., Citation2017). Horemans et al. (Citation2017) showed that 94% of enriched bacteria (MSH1) cells were associated with the carrier. The immobilization of MSH1 in alginate-porous beads supported retention of the cells and higher degradation rates. Immobilized cells in carriers are exposed to less shear force in RSF compared to suspended cells, which results in an improved bioaugmentation (Horemans et al., Citation2017). Thus, if high shear stress limit biomass attachment, encapsulation or carrier material can be considered as a potential solution for enriched biomass retention (Benner et al., Citation2013). In addition, it has been suggested that the bio-carriers can be modified by adding extra nutrient to support the inside biomass growth (Horemans et al., Citation2017).
Biofilm formation and retention are pivotal for maintaining biomass in RSF and thus obtaining stable OMPs biodegradation in RSF. Previous studies show that cell surface hydrophobicity can promote the interactions with hydrophobic compounds (Dahlbäck et al., Citation1981). The hydrophobicity of the cell surface is important in adhesion because of the hydrophobic interactions between cell surface and substratum surface (Donlan & Costerton, Citation2002; Rosenberg & Kjelleberg, Citation1986). Thus, the biofilm retention in a biocarrier can be increased using hydrophobic granular material.
While bio-carriers can support biomass retention, especially during bioaugmentation, it is important to consider the effect of such carriers on RSF functioning. For example, precipitation of iron/manganese oxides on biocarriers is expected in RSF, preventing biocarriers from functioning as protective surfaces for biofilm formation. Thus, it is important to design biocarriers that maintain their function in fully operating RSF systems.
5. Outlooks
5.1. Transformation products production and control
In RSF, most of OMPs biodegradation is incomplete and transformation products (TPs) can be formed. For instance, it has been shown that phenazone, propyphenazone and demethylaminophenazone (DMAA) can be degraded during microcosm experiments with biologically active sand material collected from field-scale RSF (Zuehlke et al., Citation2007). Meanwhile, related TPs of DMAA are formed during the treatment process, such as 1-acetyl-1-methyl-2-dimethyloxamoyl-2-phenylhydrazide, 1-acetyl-1-methyl-2-phenylhydrazide, formylaminoantipyrine, and acetoaminoantipyrine (Zuehlke et al., Citation2007). In the subsequent field investigation, Zuehlke et al. (Citation2007) find that phenazone and propyphenazone have degraded into their metabolites.
Those TPs sometimes are sometimes more recalcitrant than the original OMPs. For example, degradation of the pesticide bentazone shows that large amounts of metabolites containing the benzene-ring are still present in the effluent (Hedegaard & Albrechtsen, Citation2014). Additionally, CO2 production occurs much after bentazone removal, indicating the production of TPs in the filter (Hedegaard & Albrechtsen, Citation2014). In another microcosm experiment, investigators use AOB to co-metabolize sulfamethoxazole, which results in the formation of 4-nitro-sulfamethoxazole (Kassotaki et al., Citation2016). The researchers also found that 4-nitro-sulfamethoxazole can be transformed back to the parent compound (sulfamethoxazole) (Kassotaki et al., Citation2016). In fact, many studies show that the OMPs, especially pharmaceutical compounds, cannot be removed completely by biodegradation (Lindqvist et al., Citation2005; Ternes, Citation1998).
Therefore, RSF-based treatment technologies must go beyond merely considering removal of the original OMPs and ensure effective removal of all TPs. Firstly, TPs and end products of mineralization should be monitored by specific techniques during OMPs biodegradation. For example, the pathways of and TPs formation during bentazone biodegradation have been elucidated by an isotopic tracer method in RSF (Hedegaard & Albrechtsen, Citation2014). The result showed that bentazone was initially cleaved in the removal process. One metabolite containing the carbonyl group is removed rapidly from the water phase and slowly mineralized after 24 h, while another one containing the benzene-ring is still present in the water phase and biodegraded only after seven days (Hedegaard & Albrechtsen, Citation2014).
Similarly, the toxicity of TPs should be further analyzed and effectively relieved during drinking water production. A variety of degradation pathways exist for one compound in RSF, such as chemical oxidation by FeOx/MnOx as well as biodegradation by different autotrophic or heterotrophic microorganisms (), resulting in a variety of potential TPs. The combination of different TPs may further increase the toxicity. Thus, TPs should be closely monitored chemically and using bioassays to measure toxicity. Cell-based bioassays covering various toxicity mechanisms, including specific and reactive modes of toxic action, induction of xenobiotic metabolism, adaptive stress responses, and receptor-mediated effects, have been applied to detect the presence of OMPs and TPs in drinking water and assess their toxicity on cellular level (Escher et al., Citation2014; Neale et al., Citation2015). When required, extra treatment methods should be applied to remove toxic TPs to ensure the safety and quality of drinking water. For example, RSF can be bioaugmented by specific heterotrophic degraders to mineralize target TPs effectively. For the compounds which are recalcitrant to biodegradation, a downstream step, such as the adsorbent filter, can be designed and combined with RSF in series to ensure their effective removal.
5.2. Application in the field with full-scale RSF
Since RSF is an existing and widely used treatment technique at DWTPs, it can be more economic to exploit and improve OMPs removal in RSF, rather than implementing a new treatment step. While there are a number of column-scale studies indicating the potential for OMPs removal in RSF, few field experiments have been performed (). To effectively apply RSF for OMP removal at DWTPs, the following challenges must be examined in field investigations.
5.2.1. Stability of OMPs biodegradation
Under field condition, different environmental factors can affect long-term stability of OMPs biodegradation in RSF. Firstly, a key difference between column and field-scale experiments is the consistency in contaminant concentrations. Previous research has shown that it is challenging to maintain biodegradation activity in long-term RSF operation due to varying concentration of OMPs in influent (Baghapour et al., Citation2013; Zearley & Summers, Citation2012). In addition, diverse compositions of natural inorganic and organic contaminants in source water dictate growing environments for OMPs degraders, not the presence of trace concentrations of OMPs (Hedegaard et al., Citation2014). The abundances of ammonium, methane and AOC can influence the growth of AOB, MOB, and heterotrophic communities, both those participating in OMPs biodegradation, but also those in competition with OMP degraders. Similarly, initial concentrations of Fe(II) and Mn(II) in raw water can affect the precipitation of metal oxides on filter sand, thereby having an effect on adherence of OMPs degraders (Albers et al., Citation2015; Hedegaard et al., Citation2014).
Secondly, most laboratory experiments are performed at room temperature, which is not representative for RSF in field conditions. Previous research shows that temperature has a significant effect on OMPs biodegradation in RSF (Elhadi et al., Citation2006). In the filters fed with geosmin and MIB at 100 ng/L, a reduction in temperature from 20 °C to 8 °C resulted in 15%–25% lower OMPs removal efficiencies (Elhadi et al., Citation2006). Furthermore, Albers et al. (Citation2015) found that the degradation rate of BAM at approximately 10 °C in pilot waterworks is only half that at 20 °C in laboratory experiments. Thirdly, the operating duration of RSF can also affect the potential for OMPs biodegradation. Hedegaard et al. (Citation2014) found that the concentration of MCPP in effluent water of RSF decresed rapidly after exposure to MCPP for at least three years. This corresponds to previous observation that an adaptation period is required before the initiation of OMPs biodegradation (Janniche et al., Citation2010; Tuxen et al., Citation2000). If a RSF requires a long startup period, addition of used sand which has been exposed to relative OMPs can be considered as a promising method to shorten the adaption period. However, it is important to develop strategies to minimize the downtime of field RSF for this inoculation. Thus, field investigations are required to elucidate the influence of variable contaminants concentrations, changing temperatures, and different operation durations on RSF performance.
5.2.2. Bioaugmentation in field condition
It is clear that bioaugmentation is one of promising approach to improve biodegradation of some recalcitrant compounds in RSF, while the retention and distribution of inoculated bacteria and potential secondary pollution remain challenges that must be investigated in future field-scale studies. Previous research shows that the densities of inoculated bacteria in the top of filter bed is much higher, indicating that bacteria can be immediately adhered once they contact with the filter material (Albers et al., Citation2015). However, a higher concentration of the inoculated population in the top sand layer means that this population is more easily flushed out from RSF by backwashing flow (Albers et al., Citation2015; Hozalski & Bouwer, Citation1998). In addition, previous studies show a correlation between the amount of FeOx precipitates and the number of inoculated bacteria in backwashed water (Albers et al., Citation2015; Hozalski & Bouwer, Citation1998).The larger amount of FeOx is normally formed in upper layer (Hedegaard et al., Citation2014), where bacteria are gathered because they prefer adherence to FeOx (Albers et al., Citation2015). Thus, two filters connected in series will have more advantages for bioaugmentation. Due to large removal of iron in the primary filter, both backwashing intensity and FeOx amount can be much lower in second filter (Albers et al., Citation2015). Hence, not only a wider bacterial distribution can be obtained, but a large loss of biomass by backwashing can be avoided due to reduced backwashing requirements in the second filter. However, some nutrient will also be consumed in the first filter, thereby leaving an even more oligotrophic growing environment for inoculated bacteria in the second filter (Albers et al., Citation2015). Addition of extra AOC or nutrients may improve the condition for sustaining inoculated population (Albers et al., Citation2015). But it will require additional monitoring to avoid the secondary pollution, such as residual AOC or nutrients in the effluent. Finally, in future optimization of bioaugmented RSF, the amount of inoculated bacteria in effluent from RSF also need to be measured to ensure that the quality of drinking water will not be impaired by residual biomass.
5.2.3. Synergistic operation with other treatment technologies
Recent research has shown that many other traditional treatment processes also contribute to OMPs removal at DWTP (Benner et al., Citation2013). The growth of heterotrophic bacteria during aeration causes the biological degradation of a wide range of pharmaceuticals (Burke et al., Citation2013). As a tertiary treatment process, activated carbon filters also show OMPs removal via both adsorption and biodegradation (Li et al., Citation2012; Piai et al., Citation2019; Westerhoff et al., Citation2005). Additionally, other treatment processes can also influence OMPs biodegradation in RSF. For example, aeration normally removes the majority of methane from groundwater, which will cause the starvation of MOB which in turn will have an adverse impact on OMPs biodegradation by MOB. Thus, RSF should be seen as one step within an DWTP, whose function is dependent on the synergistic performance of the entire chain of treatment technologies. By considering the DWTP as a set of interconnected treatment steps, we can optimally tune each treatment to achieve the best performance of OMPs removal for drinking water production.
6. Conclusions
This review demonstrates that optimization of RSF is a promising step for OMPs removal at DWTPs.
Biodegradation is the main removal process for OMPs rather than adsorption and degradation by FeOx or MnOx. OMPs can be biodegraded by enzymes produced by AOB or/and MOB. Heterotrophic communities can biodegrade OMPs and their oxidized metabolites to be their primary substrate for growth. Since different native populations coexist in field-scale RSF at DWTPs, more detailed data and information need to be studied for better understanding their different contributions and interactions during OMPs removal processes.
Bioaugmentation has the potential to improve the OMPs biodegradation in RSF. To obtain effective bioaugmentation, we suggest that the bacterial strains should be enriched with the target OMPs under relevant conditions to ensure survival under oligotrophic conditions.
Critical EBCT and backwashing strategies should be determined to enhance microbial degradation of OMPs and reduce the biomass loss during RSF. We advise that the EBCT should be optimized for enhanced biodegradability of target OMPs while maintaining the basic functions of RSF. Backwashing protocols could be more mild during the filter startup and initial period of bioaugmentation, to reduce biomass loss. Furthermore, bio-carriers should be developed that immobilize microbial cells and protect enriched OMPs degraders from the rapid flow.
During future application, RSF-based treatment technologies must go beyond merely considering removal of the original OMPs and ensure complete removal of all TPs. On the other hand, we should consider the influence of field factors on stability of OMPs biodegradation, the challenges during bioaugmentation, and synergistic operation with other treatment steps in field-scale RSF.
The combination of current knowledge and potential optimization approaches described here offer a step toward enhancing OMPs removal in RSF, thereby optimally using existing infrastructure for improving the function of DWTPs.
Abbreviations | ||
2,6-DCBA | = | 2,6-dichlorobenzoic acid |
AOB | = | ammonia oxidizing bacteria |
AOC | = | assimilable organic carbon |
BAM | = | 2,6-dichlorobenzamide |
DMAA | = | demethylaminophenazone |
DWTP | = | drinking water treatment plant |
EBCT | = | empty bed contact time |
FeOx | = | Fe oxides |
MCPP | = | mecoprop |
MIB | = | 2-methylisoborneol |
MnOB | = | manganese oxidizing bacteria |
MnOx | = | Mn oxides |
MOB | = | methane oxidizing bacteria |
OMP | = | organic micropollutant |
RSF | = | rapid sand filtration |
TP | = | transformation product |
WWTP | = | wastewater treatment plant |
Supplemental Material
Download MS Word (26.6 KB)Additional information
Funding
References
- Ahmad, J., Naeem, S., Ahmad, M., Usman, A. R. A., & Al-Wabel, M. I. (2019). A critical review on organic micropollutants contamination in wastewater and removal through carbon nanotubes. Journal of Environmental Management, 246, 214–228. https://doi.org/10.1016/j.jenvman.2019.05.152
- Albers, C. N., Feld, L., Ellegaard-Jensen, L., & Aamand, J. (2015). Degradation of trace concentrations of the persistent groundwater pollutant 2,6-dichlorobenzamide (BAM) in bioaugmented rapid sand filters. Water Research, 83, 61–70. https://doi.org/10.1016/j.watres.2015.06.023
- Arciero, D., Vannelli, T., Logan, M., & Hooper, A. B. (1989). Degradation of trichloroethylene by the ammonia-oxidizing bacterium Nitrosomonas europaea. Biochemical and Biophysical Research Communications, 159(2), 640–643. https://doi.org/10.1016/0006-291X(89)90042-9
- Baghapour, M. A., Nasseri, S., & Derakhshan, Z. (2013). Atrazine removal from aqueous solutions using submerged biological aerated filter. Journal of Environmental Health Science & Engineering, 11(1), 6. https://doi.org/10.1186/2052-336X-11-6
- Bai, Y., Chang, Y., Liang, J., Chen, C., & Qu, J. (2016). Treatment of groundwater containing Mn(II), Fe(II), As(III) and Sb(III) by bioaugmented quartz-sand filters. Water Research, 106, 126–134. https://doi.org/10.1016/j.watres.2016.09.040
- Bar-Zeev, E., Belkin, N., Liberman, B., Berman, T., & Berman-Frank, I. (2012). Rapid sand filtration pretreatment for SWRO: Microbial maturation dynamics and filtration efficiency of organic matter. Desalination, 286, 120–130. https://doi.org/10.1016/j.desal.2011.11.010
- Batt, A. L., Kim, S., & Aga, D. S. (2006). Enhanced biodegradation of iopromide and trimethoprim in nitrifying activated sludge. Environmental Science & Technology, 40(23), 7367–7373. https://doi.org/10.1021/es060835v
- Bellusci, M., La Barbera, A., Padella, F., Mancuso, M., Pasquo, A., Grollino, M. G., Leter, G., Nardi, E., Cremisini, C., Giardullo, P., & Pacchierotti, F. (2014). Biodistribution and acute toxicity of a nanofluid containing manganese iron oxide nanoparticles produced by a mechanochemical process. International Journal of Nanomedicine, 9(1), 1919–1929. https://doi.org/10.2147/IJN.S56394
- Benner, J., De Smet, D., Ho, A., Kerckhof, F. M., Vanhaecke, L., Heylen, K., & Boon, N. (2015). Exploring methane-oxidizing communities for the co-metabolic degradation of organic micropollutants. Applied Microbiology and Biotechnology, 99(8), 3609–3618. https://doi.org/10.1007/s00253-014-6226-1
- Benner, J., Helbling, D. E., Kohler, H.-P E., Wittebol, J., Kaiser, E., Prasse, C., Ternes, T. A., Albers, C. N., Aamand, J., Horemans, B., Springael, D., Walravens, E., & Boon, N. (2013). Is biological treatment a viable alternative for micropollutant removal in drinking water treatment processes? Water Research, 47(16), 5955–5976. https://doi.org/10.1016/j.watres.2013.07.015
- Benner, J., & Ternes, T. A. (2009). Ozonation of metoprolol: Elucidation of oxidation pathways and major oxidation products. Environmental Science & Technology, 43(14), 5472–5480. https://doi.org/10.1021/es900280e
- Bernard, S., Chazal, P., & Mazet, M. (1997). Removal of organic compounds by adsorption on pyrolusite (β-MnO2). Water Research, 31(5), 1216–1222. https://doi.org/10.1016/S0043-1354(96)00149-2
- Bruins, J. H., Petrusevski, B., Slokar, Y. M., Huysman, K., Joris, K., Kruithof, J. C., & Kennedy, M. D. (2015). Biological and physico-chemical formation of Birnessite during the ripening of manganese removal filters. Water Research, 69, 154–161. https://doi.org/10.1016/j.watres.2014.11.019
- Bruins, J. H., Petrusevski, B., Slokar, Y. M., Kruithof, J. C., & Kennedy, M. D. (2014). Manganese removal from groundwater: Characterization of filter media coating. Desalination & Water Treatment, 55(7), 1–13.
- Brusseau, G. A., Tsien, H.-C., Hanson, R. S., & Wackett, L. P. (1990). Optimization of trichloroethylene oxidation by methanotrophs and the use of a colorimetric assay to detect soluble methane monooxygenase activity. Biodegradation, 1(1), 19–29. https://doi.org/10.1007/BF00117048
- Burke, V., Duennbier, U., & Massmann, G. (2013). The effect of aeration on the removal of wastewater-derived pharmaceutical residues from groundwater – A laboratory study. Water Science and Technology, 67(3), 658–666. https://doi.org/10.2166/wst.2012.613
- Cakmakci, M., Koyuncu, I., & Kinaci, C. (2008). Effects of iron concentrations, filter hydraulic loading rates, and porosities on iron removal by rapid sand filtration. Environmental Engineering Science, 25(5), 669–676. https://doi.org/10.1089/ees.2007.0060
- Cartinella, J. L., Cath, T. Y., Flynn, M. T., Miller, G. C., Hunter, K. W., & Childress, A. E. (2006). Removal of natural steroid hormones from wastewater using membrane contactor processes. Environmental Science & Technology, 40(23), 7381–7386. https://doi.org/10.1021/es060550i
- Colby, J., Stirling, D. I., & Dalton, H. (1977). The soluble methane mono-oxygenase of Methylococcus capsulatus (Bath). Its ability to oxygenate n-alkanes, n-alkenes, ethers, and alicyclic, aromatic and heterocyclic compounds. The Biochemical Journal, 165(2), 395–402. https://doi.org/10.1042/bj1650395
- Cooper, J. E., Kendig, E. L., & Belcher, S. M. (2011). Assessment of bisphenol A released from reusable plastic, aluminium and stainless steel water bottles. Chemosphere, 85(6), 943–947. https://doi.org/10.1016/j.chemosphere.2011.06.060
- Costerton, J. W., Lewandowski, Z., Caldwell, D. E., Korber, D. R., & Lappin-Scott, H. M. (1995). Microbial biofilms. Annual Review of Microbiology, 49(1), 711–745. https://doi.org/10.1146/annurev.mi.49.100195.003431
- Craft, T. F. (1966). Review of rapid sand filtration theory. Journal of American Water Works Association, 58(4), 428–439. https://doi.org/10.1002/j.1551-8833.1966.tb01600.x
- Dahlbäck, B., Hermansson, M., Kjelleberg, S., & Norkrans, B. (1981). The hydrophobicity of bacteria – An important factor in their initial adhesion at the air-water interface. Archives of Microbiology, 128(3), 267–270. https://doi.org/10.1007/BF00422527
- de Jongh, C. M., Kooij, P. J. F., de Voogt, P., & ter Laak, T. L. (2012). Screening and human health risk assessment of pharmaceuticals and their transformation products in Dutch surface waters and drinking water. The Science of the Total Environment, 427-428, 70–77. https://doi.org/10.1016/j.scitotenv.2012.04.010
- de Moel, P. J., Verberk, J. Q. J. C., & van Dijk, J. C. (2006). Drinking water principles and practices. World Scientific Publishing Co. Pte. Ltd.
- de Vet, W. W. J. M., Kleerebezem, R., van der Wielen, P. W. J. J., Rietveld, L. C., & van Loosdrecht, M. C. M. (2011). Assessment of nitrification in groundwater filters for drinking water production by qPCR and activity measurement. Water Research, 45(13), 4008–4018. https://doi.org/10.1016/j.watres.2011.05.005
- Ding, C., Xin, Y., Wei, L., Chang, Y., & Shang, C. (2010). Removal of natural organic matter using surfactant-modified iron oxide-coated sand. Journal of Hazardous Materials, 174(1–3), 567–572. https://doi.org/10.1016/j.jhazmat.2009.09.089
- Dodd, M. C., Rentsch, D., Singer, H. P., Kohler, H. P. E., & von Gunten, U. (2010). Transformation of β-lactam antibacterial agents during aqueous ozonation: Reaction pathways and quantitative bioassay of biologically-active oxidation products. Environmental Science & Technology, 44(15), 5940–5948. https://doi.org/10.1021/es101061w
- Donlan, R. M., & Costerton, J. W. (2002). Biofilms: Survival mechanisms of clinically relevant microorganisms. Clinical Microbiology Reviews, 15(2), 167–193. https://doi.org/10.1128/CMR.15.2.167-193.2002
- Elhadi, S. L. N., Huck, P. M., & Slawson, R. M. (2006). Factors affecting the removal of geosmin and MIB in drinking water biofilters. Journal of American Water Works Association, 98(8), 108–119. https://doi.org/10.1002/j.1551-8833.2006.tb07738.x
- Ellegaard-Jensen, L., Albers, C. N., & Aamand, J. (2016). Protozoa graze on the 2,6-dichlorobenzamide (BAM)-degrading bacterium Aminobacter sp. MSH1 introduced into waterworks sand filters. Applied Microbiology and Biotechnology, 100(20), 8965–8973. https://doi.org/10.1007/s00253-016-7710-6
- Escher, B. I., Allinson, M., Altenburger, R., Bain, P. A., Balaguer, P., Busch, W., Crago, J., Denslow, N. D., Dopp, E., Hilscherova, K., Humpage, A. R., Kumar, A., Grimaldi, M., Jayasinghe, B. S., Jarosova, B., Jia, A., Makarov, S., Maruya, K. A., Medvedev, A., … Leusch, F. D. L. (2014). Benchmarking organic micropollutants in wastewater, recycled water and drinking water with in vitro bioassays. Environmental Science & Technology, 48(3), 1940–1956. https://doi.org/10.1021/es403899t
- Fatta-Kassinos, D., Vasquez, M. I., & Kümmerer, K. (2011). Transformation products of pharmaceuticals in surface waters and wastewater formed during photolysis and advanced oxidation processes – Degradation, elucidation of byproducts and assessment of their biological potency. Chemosphere, 85(5), 693–709. https://doi.org/10.1016/j.chemosphere.2011.06.082
- Feakin, S. J., Gubbins, B., McGhee, I., Shaw, L. J., & Burns, R. G. (1995). Inoculation of granular activated carbon with s-triazine-degrading bacteria for water treatment at pilot-scale. Water Research, 29(7), 1681–1688. https://doi.org/10.1016/0043-1354(94)00322-X
- Feitosa-Felizzola, J., Hanna, K., & Chiron, S. (2009). Adsorption and transformation of selected human-used macrolide antibacterial agents with iron(III) and manganese(IV) oxides. Environmental Pollution (Barking, Essex: 1987), 157(4), 1317–1322. https://doi.org/10.1016/j.envpol.2008.11.048
- Feld, L., Nielsen, T. K., Hansen, L. H., Aamand, J., & Albers, C. N. (2016). Establishment of bacterial herbicide degraders in a rapid sand filter for bioremediation of phenoxypropionate-polluted groundwater. Applied and Environmental Microbiology, 82(3), 878–887. https://doi.org/10.1128/AEM.02600-15
- Forrez, I., Carballa, M., Fink, G., Wick, A., Hennebel, T., Vanhaecke, L., Ternes, T., Boon, N., & Verstraete, W. (2011). Biogenic metals for the oxidative and reductive removal of pharmaceuticals, biocides and iodinated contrast media in a polishing membrane bioreactor. Water Research, 45(4), 1763–1773. https://doi.org/10.1016/j.watres.2010.11.031
- Fulthorpe, R. R., Rhodes, A. N., & Tiedje, J. M. (1996). Pristine soils mineralize 3-chlorobenzoate and 2,4-dichlorophenoxyacetate via different microbial populations. Applied and Environmental Microbiology, 62(4), 1159–1166. https://doi.org/10.1128/AEM.62.4.1159-1166.1996
- Furgal, K. M., Meyer, R. L., & Kai, B. (2015). Removing selected steroid hormones, biocides and pharmaceuticals from water by means of biogenic manganese oxide nanoparticles in situ at ppb levels. Chemosphere, 136, 321–326. https://doi.org/10.1016/j.chemosphere.2014.11.059
- Gözdereliler, E., Boon, N., Aamand, J., De Roy, K., Granitsiotis, M. S., Albrechtsen, H.-J., & Sørensen, S. R. (2013). Comparing metabolic functionalities, community structures, and dynamics of herbicide-degrading communities cultivated with different substrate concentrations. Applied and Environmental Microbiology, 79(1), 367–375. https://doi.org/10.1128/AEM.02536-12
- Gude, J. C. J., Rietveld, L. C., & van Halem, D. (2016). Fate of low arsenic concentrations during full-scale aeration and rapid filtration. Water Research, 88, 566–574. https://doi.org/10.1016/j.watres.2015.10.034
- Gude, J. C. J., Rietveld, L. C., & van Halem, D. (2018). Biological As(III) oxidation in rapid sand filters. Journal of Water Process Engineering, 21, 107–115. https://doi.org/10.1016/j.jwpe.2017.12.003
- Hedegaard, M. J., & Albrechtsen, H. J. (2014). Microbial pesticide removal in rapid sand filters for drinking water treatment – Potential and kinetics. Water Research, 48(1), 71–81. https://doi.org/10.1016/j.watres.2013.09.024
- Hedegaard, M. J., Arvin, E., Corfitzen, C. B., & Albrechtsen, H. J. (2014). Mecoprop (MCPP) removal in full-scale rapid sand filters at a groundwater-based waterworks. Science of the Total Environment, 499, 257–264. https://doi.org/10.1016/j.scitotenv.2014.08.052
- Hedegaard, M. J., Deliniere, H., Prasse, C., Dechesne, A., Smets, B. F., & Albrechtsen, H.-J. (2018). Evidence of co-metabolic bentazone transformation by methanotrophic enrichment from a groundwater-fed rapid sand filter. Water Research, 129, 105–114. https://doi.org/10.1016/j.watres.2017.10.073
- Hedegaard, M. J., Prasse, C., & Albrechtsen, H.-J. (2019). Microbial degradation pathways of the herbicide bentazone in filter sand used for drinking water treatment. Environmental Science: Water Research & Technology, 5(3), 521–532. https://doi.org/10.1039/C8EW00790J
- Ho, L., Meyn, T., Keegan, A., Hoefel, D., Brookes, J., Saint, C. P., & Newcombe, G. (2006). Bacterial degradation of microcystin toxins within a biologically active sand filter. Water Research, 40(4), 768–774. https://doi.org/10.1016/j.watres.2005.12.009
- Horemans, B., Raes, B., Vandermaesen, J., Simanjuntak, Y., Brocatus, H., T'Syen, J., Degryse, J., Boonen, J., Wittebol, J., Lapanje, A., Sørensen, S. R., & Springael, D. (2017). Biocarriers improve bioaugmentation efficiency of a rapid sand filter for the treatment of 2,6-dichlorobenzamide-contaminated drinking water. Environmental Science & Technology, 51(3), 1616–1625. https://doi.org/10.1021/acs.est.6b05027
- Howdeshell, K. L., Hotchkiss, A. K., Thayer, K. A., Vandenbergh, J. G., & Vom Saal, F. S. (1999). Exposure to bisphenol A advances puberty. Nature, 401(6755), 763–764. https://doi.org/10.1038/44517
- Hozalski, R. M., & Bouwer, E. J. (1998). Deposition and retention of bacteria in backwashed filters. Journal of American Water Works Association, 90(1), 71–85. https://doi.org/10.1002/j.1551-8833.1998.tb08362.x
- Hu, W., Liang, J., Ju, F., Wang, Q., Liu, R., Bai, Y., Liu, H., & Qu, J. (2020). Metagenomics unravels differential microbiome composition and metabolic potential in rapid sand filters purifying surface water versus groundwater. Environmental Science & Technology, 54(8), 5197–5206. https://doi.org/10.1021/acs.est.9b07143
- Hyman, M. R., Murton, I. B., & Arp, D. J. (1988). Interaction of ammonia monooxygenase from Nitrosomonas europaea with alkanes, alkenes, and alkynes. Applied and Environmental Microbiology, 54(12), 3187–3190. https://doi.org/10.1128/AEM.54.12.3187-3190.1988
- Im, J., & Semrau, J. D. (2011). Pollutant degradation by a Methylocystis strain SB2 grown on ethanol: Bioremediation via facultative methanotrophy. FEMS Microbiology Letters, 318(2), 137–142. https://doi.org/10.1111/j.1574-6968.2011.02249.x
- Ives, K. J. (1970). Rapid filtration. Water Research, 4(3), 201–223. https://doi.org/10.1016/0043-1354(70)90068-0
- Janniche, G. S., Lindberg, E., Mouvet, C., & Albrechtsen, H. J. (2010). Mineralization of isoproturon, mecoprop and acetochlor in a deep unsaturated limestone and sandy aquifer. Chemosphere, 81(7), 823–831. https://doi.org/10.1016/j.chemosphere.2010.08.023
- Jian, Z., Bai, Y., Chang, Y., Liang, J., & Qu, J. (2019). Removal of micropollutants and cyanobacteria from drinking water using KMnO4 pre-oxidation coupled with bioaugmentation. Chemosphere, 215, 1–7. https://doi.org/10.1016/j.chemosphere.2018.10.013
- Jochimsen, E. M., Carmichael, W. W., An, J., Cardo, D. M., Cookson, S. T., Holmes, C. E., Antunes, M. B., De Melo Filho, D. A., Lyra, T. M., Barreto, V. S. T., Azevedo, S. M., & Jarvis, W. R. (1998). Liver failure and death after exposure to microcystins at a hemodialysis center in Brazil. New England Journal of Medicine, 338(13), 873–878. https://doi.org/10.1056/NEJM199803263381304
- Jones, O. A., Lester, J. N., & Voulvoulis, N. (2005). Pharmaceuticals: A threat to drinking water? Trends in Biotechnology, 23(4), 163–167. https://doi.org/10.1016/j.tibtech.2005.02.001
- Kassotaki, E., Buttiglieri, G., Ferrando-Climent, L., Rodriguez-Roda, I., & Pijuan, M. (2016). Enhanced sulfamethoxazole degradation through ammonia oxidizing bacteria co-metabolism and fate of transformation products. Water Research, 94, 111–119. https://doi.org/10.1016/j.watres.2016.02.022
- Kim, J., & Kang, B. (2008). DBPs removal in GAC filter-adsorber. Water Research, 42(1–2), 145–152. https://doi.org/10.1016/j.watres.2007.07.040
- Kirby, C. S., Thomas, H. M., Southam, G., & Donald, R. (1999). Relative contributions of abiotic and biological factors in Fe(II) oxidation in mine drainage. Applied Geochemistry, 14(4), 511–530. https://doi.org/10.1016/S0883-2927(98)00071-7
- Kolpin, D. W., Furlong, E. T., Meyer, M. T., Thurman, E. M., Zaugg, S. D., Barber, L. B., & Buxton, H. T. (2002). Pharmaceuticals, hormones, and other organic wastewater contaminants in U.S. streams, 1999–2000: A national reconnaissance. Environmental Science & Technology, 36(6), 1202–1211. https://doi.org/10.1021/es011055j
- Kors, L. J., Moorman, J. H. N., Wind, A. P. M., & van der Hoek, J. P. (1998). Nitrification and low temperature in a raw water reservoir and rapid sand filters. Water Science and Technology, 37(2), 169–176. https://doi.org/10.2166/wst.1998.0131
- Košutić, K., Furač, L., Sipos, L., & Kunst, B. (2005). Removal of arsenic and pesticides from drinking water by nanofiltration membranes. Separation and Purification Technology, 42(2), 137–144. https://doi.org/10.1016/j.seppur.2004.07.003
- Larsen, T. A., Lienert, J., Joss, A., & Siegrist, H. (2004). How to avoid pharmaceuticals in the aquatic environment. Journal of Biotechnology, 113(1–3), 295–304. https://doi.org/10.1016/j.jbiotec.2004.03.033
- Lee, C. O., Boe-Hansen, R., Musovic, S., Smets, B., Albrechtsen, H. J., & Binning, P. (2014). Effects of dynamic operating conditions on nitrification in biological rapid sand filters for drinking water treatment. Water Research, 64(7), 226–236. https://doi.org/10.1016/j.watres.2014.07.001
- Lee, Y., & Gunten, U. V. (2010). Oxidative transformation of micropollutants during municipal wastewater treatment: Comparison of kinetic aspects of selective (chlorine, chlorine dioxide, ferrate VI, and ozone) and non-selective oxidants (hydroxyl radical) ). Water Research, 44(2), 555–566. https://doi.org/10.1016/j.watres.2009.11.045
- Li, Z., Dvorak, B., & Li, X. (2012). Removing 17beta-estradiol from drinking water in a biologically active carbon (BAC) reactor modified from a granular activated carbon (GAC) reactor. Water Research, 46(9), 2828–2836. https://doi.org/10.1016/j.watres.2012.03.033
- Lindqvist, N., Tuhkanen, T., & Kronberg, L. (2005). Occurrence of acidic pharmaceuticals in raw and treated sewages and in receiving waters. Water Research, 39(11), 2219–2228. https://doi.org/10.1016/j.watres.2005.04.003
- Liu, R., Sun, L., Qu, J., & Li, G. (2009). Arsenic removal through adsorption, sand filtration and ultrafiltration: In situ precipitated ferric and manganese binary oxides as adsorbents. Desalination, 249(3), 1233–1237.
- Liu, W., Sutton, N. B., Rijnaarts, H. H. M., & Langenhoff, A. A. M. (2016). Pharmaceutical removal from water with iron- or manganese-based technologies: A review. Critical Reviews in Environmental Science and Technology, 46(19–20), 1584–1621. https://doi.org/10.1080/10643389.2016.1251236
- Lopato, L., Röttgers, N., Binning, P. J., & Arvin, E. (2013). Heterogeneous nitrification in a full-scale rapid sand filter treating groundwater. Journal of Environmental Engineering, 139(3), 375–384. https://doi.org/10.1061/(ASCE)EE.1943-7870.0000653
- Luo, Y., Guo, W., Ngo, H. H., Nghiem, L. D., Hai, F. I., Zhang, J., Liang, S., & Wang, X. C. (2014). A review on the occurrence of micropollutants in the aquatic environment and their fate and removal during wastewater treatment. The Science of the Total Environment, 473–474(3), 619–641. https://doi.org/10.1016/j.scitotenv.2013.12.065
- Manoli, K., Nakhla, G., Ray, A. K., & Sharma, V. K. (2017). Enhanced oxidative transformation of organic contaminants by activation of ferrate(VI): Possible involvement of FeV/FeIV species. Chemical Engineering Journal, 307, 513–517. https://doi.org/10.1016/j.cej.2016.08.109
- Mcdowell, D. C., Huber, M. M., Wagner, M., von Gunten, U., & Ternes, T. A. (2005). Ozonation of carbamazepine in drinking water: Identification and kinetic study of major oxidation products. Environmental Science & Technology, 39(20), 8014–8022. https://doi.org/10.1021/es050043l
- Meerburg, F., Hennebel, T., Vanhaecke, L., Verstraete, W., & Boon, N. (2012). Diclofenac and 2‐anilinophenylacetate degradation by combined activity of biogenic manganese oxides and silver. Microbial Biotechnology, 5(3), 388–395. https://doi.org/10.1111/j.1751-7915.2011.00323.x
- Men, Y., Han, P., Helbling, D. E., Jehmlich, N., Herbold, C., Gulde, R., Onnis-Hayden, A., Gu, A. Z., Johnson, D. R., Wagner, M., & Fenner, K. (2016). Biotransformation of two pharmaceuticals by the ammonia-oxidizing archaeon Nitrososphaera gargensis. Environmental Science & Technology, 50(9), 4682–4692. https://doi.org/10.1021/acs.est.5b06016
- Meng, Y. T., Zheng, Y. M., Zhang, L. M., & He, J. Z. (2009). Biogenic Mn oxides for effective adsorption of Cd from aquatic environment. Environmental Pollution, 157(8–9), 2577–2583. https://doi.org/10.1016/j.envpol.2009.02.035
- Michalakos, G. D., Nieva, J. M., Vayenas, D. V., & Lyberatos, G. (1997). Removal of iron from potable water using a trickling filter. Water Research, 31(5), 991–996. https://doi.org/10.1016/S0043-1354(96)00343-0
- Müller, A., Weiss, S. C., Beisswenger, J., Leukhardt, H. G., Schulz, W., Seitz, W., Ruck, W. K. L., & Weber, W. H. (2012). Identification of ozonation by-products of 4- and 5-methyl-1H-benzotriazole during the treatment of surface water to drinking water. Water Research, 46(3), 679–690. https://doi.org/10.1016/j.watres.2011.11.033
- Neale, P. A., Ait-Aissa, S., Brack, W., Creusot, N., Denison, M. S., Deutschmann, B., Hilscherová, K., Hollert, H., Krauss, M., Novák, J., Schulze, T., Seiler, T.-B., Serra, H., Shao, Y., & Escher, B. I. (2015). Linking in vitro effects and detected organic micropollutants in surface water using mixture-toxicity modeling. Environmental Science & Technology, 49(24), 14614–14624. https://doi.org/10.1021/acs.est.5b04083
- Nicolaisen, B. (2003). Developments in membrane technology for water treatment. Desalination, 153(1–3), 355–360. https://doi.org/10.1016/S0011-9164(02)01127-X
- Niu, J., Kasuga, I., Kurisu, F., Furumai, H., & Shigeeda, T. (2013). Evaluation of autotrophic growth of ammonia-oxidizers associated with granular activated carbon used for drinking water purification by DNA-stable isotope probing . Water Research, 47(19), 7053–7065. https://doi.org/10.1016/j.watres.2013.07.056
- O'Melia, C. R., & Crapps, D. K. (1964). Some chemical aspects of rapid sand filtration. Journal American Water Works Association, 56(10), 1326–1344. https://doi.org/10.1002/j.1551-8833.1964.tb01340.x
- Onesios, K. M., Yu, J. T., & Bouwer, E. J. (2009). Biodegradation and removal of pharmaceuticals and personal care products in treatment systems: A review. Biodegradation, 20(4), 441–466. https://doi.org/10.1007/s10532-008-9237-8
- Ormad, M. P., Miguel, N., Claver, A., Matesanz, J. M., & Ovelleiro, J. L. (2008). Pesticides removal in the process of drinking water production. Chemosphere, 71(1), 97–106. https://doi.org/10.1016/j.chemosphere.2007.10.006
- Papadopoulou, A., Hedegaard, M. J., Dechesne, A., Albrechtsen, H.-J., Musovic, S., & Smets, B. F. (2019). Methanotrophic contribution to biodegradation of phenoxy acids in cultures enriched from a groundwater-fed rapid sand filter. Applied Microbiology and Biotechnology, 103(2), 1007–1019. https://doi.org/10.1007/s00253-018-9501-8
- Paredes, L., Fernandez-Fontaina, E., Lema, J. M., Omil, F., & Carballa, M. (2016). Understanding the fate of organic micropollutants in sand and granular activated carbon biofiltration systems. Science of the Total Environment, 551-552, 640–648. https://doi.org/10.1016/j.scitotenv.2016.02.008
- Pereira, R. O., de Alda, M. L., Joglar, J., Daniel, L. A., & Barceló, D. (2011). Identification of new ozonation disinfection byproducts of 17β-estradiol and estrone in water. Chemosphere, 84(11), 1535–1541. https://doi.org/10.1016/j.chemosphere.2011.05.058
- Piai, L., Dykstra, J. E., Adishakti, M. G., Blokland, M., Langenhoff, A. A. M., & van der Wal, A. (2019). Diffusion of hydrophilic organic micropollutants in granular activated carbon with different pore sizes. Water Research, 162, 518–527. https://doi.org/10.1016/j.watres.2019.06.012
- Rattier, M., Reungoat, J., Keller, J., & Gernjak, W. (2014). Removal of micropollutants during tertiary wastewater treatment by biofiltration: Role of nitrifiers and removal mechanisms. Water Research, 54, 89–99. https://doi.org/10.1016/j.watres.2014.01.030
- Remucal, C. K., & Ginder-Vogel, M. (2014). A critical review of the reactivity of manganese oxides with organic contaminants. Environmental Science. Processes & Impacts, 16(6), 1247–1266. https://doi.org/10.1039/c3em00703k
- Rentz, J. A., Charoenkwan, K., Luther, G. W., & David, E. (2007). Control of ferrous iron oxidation within circumneutral microbial iron mats by cellular activity and autocatalysis. Environmental Science & Technology, 41(17), 6084–6089. https://doi.org/10.1021/es062203e
- Richter, D., Massmann, G., & Dünnbier, U. (2008). Behaviour and biodegradation of sulfonamides (p-TSA, o-TSA, BSA) during drinking water treatment. Chemosphere, 71(8), 1574–1581. https://doi.org/10.1016/j.chemosphere.2007.11.026
- Roh, H., Subramanya, N., Zhao, F., Yu, C.-P., Sandt, J., & Chu, K.-H. (2009). Biodegradation potential of wastewater micropollutants by ammonia-oxidizing bacteria. Chemosphere, 77(8), 1084–1089. https://doi.org/10.1016/j.chemosphere.2009.08.049
- Rosenberg, M., & Kjelleberg, S. (1986). Hydrophobic interactions: Role in bacterial adhesion. In K. C. Marshall (Ed.), Advances in microbial ecology (pp. 353–393). Springer US.
- Samuelsen, E. D., Badawi, N., Nybroe, O., Sorensen, S. R., & Aamand, J. (2017). Adhesion to sand and ability to mineralise low pesticide concentrations are required for efficient bioaugmentation of flow-through sand filters. Applied Microbiology and Biotechnology, 101(1), 411–421. https://doi.org/10.1007/s00253-016-7909-6
- Sarkar, B., Venkateswralu, N., Rao, R. N., Bhattacharjee, C., & Kale, V. (2007). Treatment of pesticide contaminated surface water for production of potable water by a coagulation-adsorption-nanofiltration approach. Desalination, 212(1–3), 129–140. https://doi.org/10.1016/j.desal.2006.09.021
- Schulman, L. J., Sargent, E. V., Naumann, B. D., Faria, E. C., Dolan, D. G., & Wargo, J. P. (2002). A human health risk assessment of pharmaceuticals in the aquatic environment. Human and Ecological Risk Assessment, 8(4), 657–680. https://doi.org/10.1080/20028091057141
- Semrany, S., Favier, L., Djelal, H., Taha, S., & Amrane, A. (2012). Bioaugmentation: Possible solution in the treatment of bio-refractory organic compounds (Bio-ROCs). Biochemical Engineering Journal, 69, 75–86. https://doi.org/10.1016/j.bej.2012.08.017
- Shi, J., Fujisawa, S., Nakai, S., & Hosomi, M. (2004). Biodegradation of natural and synthetic estrogens by nitrifying activated sludge and ammonia-oxidizing bacterium Nitrosomonas europaea. Water Research, 38(9), 2323–2330. https://doi.org/10.1016/j.watres.2004.02.022
- Sørensen, S. R., Holtze, M. S., Simonsen, A., & Aamand, J. (2007). Degradation and mineralization of nanomolar concentrations of the herbicide dichlobenil and its persistent metabolite 2,6-dichlorobenzamide by Aminobacter spp. isolated from dichlobenil-treated soils. Applied and Environmental Microbiology, 73(2), 399–406. https://doi.org/10.1128/AEM.01498-06
- Sullivan, J. P., Dickinson, D., & Chase, H. A. (1998). Methanotrophs, Methylosinus trichosporium OB3b, sMMO, and their application to bioremediation. Critical Reviews in Microbiology, 24(4), 335–373. https://doi.org/10.1080/10408419891294217
- Sun, Q., Li, Y., Chou, P.-H., Peng, P.-Y., & Yu, C.-P. (2012). Transformation of bisphenol A and alkylphenols by ammonia-oxidizing bacteria through nitration. Environmental Science & Technology, 46(8), 4442–4448. https://doi.org/10.1021/es204424t
- Swartjes, F. A., & van der Aa, M. (2020). Measures to reduce pesticides leaching into groundwater-based drinking water resources: An appeal to national and local governments, water boards and farmers. The Science of the Total Environment, 699, 134186https://doi.org/10.1016/j.scitotenv.2019.134186
- Tatari, K., Smets, B. F., & Albrechtsen, H. J. (2013). A novel bench-scale column assay to investigate site-specific nitrification biokinetics in biological rapid sand filters. Water Research, 47(16), 6380–6387. https://doi.org/10.1016/j.watres.2013.08.005
- Tebo, B. M., Bargar, J. R., Clement, B. G., Dick, G. J., Murray, K. J., Parker, D., Verity, R., & Webb, S. M. (2004). Biogenic manganese oxides: Properties and mechanisms of formation. Annual Review of Earth and Planetary Sciences, 32(1), 287–328. https://doi.org/10.1146/annurev.earth.32.101802.120213
- Tekerlekopoulou, A. G., Pavlou, S., & Vayenas, D. V. (2013). Removal of ammonium, iron and manganese from potable water in biofiltration units: A review. Journal of Chemical Technology & Biotechnology, 88(5), 751–773. https://doi.org/10.1002/jctb.4031
- Ternes, T. A. (1998). Occurrence of drugs in German sewage treatment plants and rivers. Water Research, 32(11), 3245–3260. https://doi.org/10.1016/S0043-1354(98)00099-2
- Ternes, T. A., Meisenheimer, M., McDowell, D., Sacher, F., Brauch, H.-J., Haist-Gulde, B., Preuss, G., Wilme, U., & Zulei-Seibert, N. (2002). Removal of pharmaceuticals during drinking water treatment. Environmental Science & Technology, 36(17), 3855–3863. https://doi.org/10.1021/es015757k
- Tom, H., Bart, D. G., Nico, B., & Willy, V. (2009). Biogenic metals in advanced water treatment. Trends in Biotechnology, 27(2), 90–98.
- Tran, N. H., Urase, T., Ngo, H. H., Hu, J., & Ong, S. L. (2013). Insight into metabolic and cometabolic activities of autotrophic and heterotrophic microorganisms in the biodegradation of emerging trace organic contaminants. Bioresource Technology, 146, 721–731. https://doi.org/10.1016/j.biortech.2013.07.083
- Tu, J., Yang, Z., Hu, C., & Qu, J. (2014). Characterization and reactivity of biogenic manganese oxides for ciprofloxacin oxidation. Journal of Environmental Sciences, 26(5), 1154–1161. https://doi.org/10.1016/S1001-0742(13)60505-7
- Tuxen, N., Tüchsen, P. L., Rügge, K., Albrechtsen, H. J., & Bjerg, P. B. (2000). Fate of seven pesticides in an aerobic aquifer studied in column experiment. Chemosphere, 41(9), 1485–1494. https://doi.org/10.1016/S0045-6535(99)00533-0
- van Beek, C. G. E. M., Hiemstra, T., Hofs, B., Nederlof, M. M., van Paassen, J. A. M., & Reijnen, G. K. (2012). Homogeneous, heterogeneous and biological oxidation of iron(II) in rapid sand filtration. Journal of Water Supply: Research and Technology-Aqua, 61(1), 1–13. https://doi.org/10.2166/aqua.2012.033
- van der Aa, L. T. J., Kors, L. J., Wind, A. P. M., Hofman, J. A. M. H., & Rietveld, L. C. (2002). Nitrification in rapid sand filter: Phosphate limitation at low temperatures. Water Supply, 2(1), 37–46. https://doi.org/10.2166/ws.2002.0005
- Vandermaesen, J., Horemans, B., Degryse, J., Boonen, J., Walravens, E., & Springael, D. (2016). Mineralization of the common groundwater pollutant 2,6-dichlorobenzamide (BAM) and its metabolite 2,6-dichlorobenzoic acid (2,6-DCBA) in sand filter units of drinking water treatment plants. Environmental Science & Technology, 50(18), 10114–10122. https://doi.org/10.1021/acs.est.6b01352
- Vandermaesen, J., Horemans, B., Degryse, J., Boonen, J., Walravens, E., & Springael, D. (2019). The pesticide mineralization capacity in sand filter units of drinking water treatment plants (DWTP): Consistency in time and relationship with intake water and sand filter characteristics. Chemosphere, 228, 427–436. https://doi.org/10.1016/j.chemosphere.2019.04.033
- Verhagen, P., De Gelder, L., Hoefman, S., De Vos, P., & Boon, N. (2011). Planktonic versus biofilm catabolic communities: Importance of the biofilm for species selection and pesticide degradation. Applied and Environmental Microbiology, 77(14), 4728–4735. https://doi.org/10.1128/AEM.05188-11
- Vries, D., Bertelkamp, C., Schoonenberg Kegel, F., Hofs, B., Dusseldorp, J., Bruins, J. H., de Vet, W., & van den Akker, B. (2017). Iron and manganese removal: Recent advances in modelling treatment efficiency by rapid sand filtration. Water Research, 109, 35–45. https://doi.org/10.1016/j.watres.2016.11.032
- Walker, S. L., Hill, J. E., Redman, J. A., & Elimelech, M. (2005). Influence of growth phase on adhesion kinetics of Escherichia coli D21g. Applied and Environmental Microbiology, 71(6), 3093–3099. https://doi.org/10.1128/AEM.71.6.3093-3099.2005
- Westerhoff, P., Yoon, Y., Snyder, S., & Wert, E. (2005). Fate of endocrine-disruptor, pharmaceutical, and personal care product chemicals during simulated drinking water treatment processes. Environmental Science & Technology, 39(17), 6649–6663. https://doi.org/10.1021/es0484799
- WHO. (1997). Report of the working group meeting on chemical substances in drinking water. World Health Organisation.
- Wright, D. A., Killham, K., Glover, L. A., & Prosser, J. I. (1995). Role of pore size location in determining bacterial activity during predation by protozoa in soil. Applied and Environmental Microbiology, 61(10), 3537–3543. https://doi.org/10.1128/AEM.61.10.3537-3543.1995
- Zearley, T. L., & Summers, R. S. (2012). Removal of trace organic micropollutants by drinking water biological filters. Environmental Science & Technology, 46(17), 9412–9419. https://doi.org/10.1021/es301428e
- Zhang, Y., Zhu, H., Szewzyk, U., & Geissen, S. U. (2015). Removal of pharmaceuticals in aerated biofilters with manganese feeding. Water Research, 72, 218–226. https://doi.org/10.1016/j.watres.2015.01.009
- Zuehlke, S., Duennbier, U., & Heberer, T. (2007). Investigation of the behavior and metabolism of pharmaceutical residues during purification of contaminated ground water used for drinking water supply. Chemosphere, 69(11), 1673–1680. https://doi.org/10.1016/j.chemosphere.2007.06.020