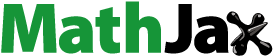
ABSTRACT
A bird’s nest-like structure of Nb2O5 (Nb2O5/PVP) was produced using a hydrothermal method that involved the introduction of PVP. This unique structure provides a micro-reactor for the photocatalytic reaction, which improves the reaction efficiency. The test results demonstrated that the surface properties of Nb2O5 were altered by the introduction of PVP. This change caused an enhancement of the water adsorption capacity and ∙OH production yield. Another aspect, the energy band structure was altered by the introduction of PVP. Thus, the photogenerated charge recombination rate was reduced, the photogenerated charge mobility was improved, the reduction of photogenerated electrons was enhanced, and the ∙O2- production yield was increased. The PVP introduction broadened the light absorption range of Nb2O5 from the UV to the visible light region, increasing the light utilization efficiency. The degradation experiments demonstrated that Nb2O5/PVP had significantly better catalytic activity than pristine Nb2O5. Meanwhile, the photocatalytic degradation efficiency of TC using Nb2O5/PVP reached 90.4% in 25 min.
Introduction
Semiconductor photocatalytic technology is a highly effective method for converting solar energy into chemical energy, making it an ideal choice for individuals and organizations committed to environmental sustainability and energy conservation [Citation1,Citation2]. By utilizing this technology, we can make significant strides towards a more sustainable future. This technology has been widely recognized for its potential applications in wastewater treatment, carbon dioxide conversion, organic compound synthesis, photocatalytic hydrogen production, and bacterial and virus elimination [Citation3–9]. The study of semiconductor photocatalytic technology is of utmost importance.
Photocatalytic reactions involve several key steps, including light absorption, photogenerated charge generation, photogenerated charge complexation, photogenerated charge migration, and active species generation [Citation7,Citation10]. The efficiency of visible light utilization is ensured by a suitable forbidden band width, which results in the production of strong reduction of photogenerated electrons or strong oxidation of photogenerated holes [Citation11]. These processes are well-established and have been extensively studied, leading to a high level of confidence in their effectiveness. Additionally, it is important to acknowledge the potential for further optimization and improvement in this field, and to approach these challenges with a diplomatic and collaborative mindset. The efficiency of the photocatalytic reaction is determined by the activity of the photogenerated charges, the degree of photogenerated charge migration and complexation, and the surface structure of the photocatalytic material [Citation11,Citation12]. These processes are interrelated and critically dependent on the internal (electronic) structure of the photocatalytic semiconductor material, which strongly influences the degree of photogenerated charge migration and complexation, and consequently the yield of active species. The photocatalytic reaction efficiency is determined by the activity of the photogenerated charges, the degree of photogenerated charge migration and complexation, and the surface structure of the photocatalytic material [Citation13,Citation14]. Studying any of these processes in semiconductor photocatalytic materials is highly significant.
It is also very interesting to modify the existing semiconductor photocatalytic materials, especially to prepare photocatalytic materials with different morphologies and unexpectedly find that different morphologies determine different physicochemical properties. Nb2O5 is an n-type wide bandgap semiconductor similar to TiO2 [Citation15,Citation16]. Similarly, Nb2O5 is regarded as a potential photocatalytic material because of its benefits such as low toxicity, excellent thermodynamic stability, and relatively high photocatalytic activity [Citation16,Citation17]. However, the photocatalytic activity of pristine Nb2O5 has been severely limited by its shortcomings, including a low light absorption intensity, particularly in the visible light regime, a poor crystallinity, and an extremely fast recombination rate of photoinduced carriers. So far, various schemes such as noble metal loading [Citation18,Citation19], metal ion doping [Citation17], and heterojunction assembly [Citation15,Citation20] have been investigated to modify Nb2O5 resulting in a significant boost in photocatalytic performance. The study about shape-shifting to optimize the visible light response of Nb2O5 and to improve its properties has been little explored. As a result, the systematic study of shape-shifting to expand the absorption range and improve the photocatalytic ability of Nb2O5 is an appealing work.
In this work, a bird’s nest-like structure of Nb2O5 (Nb2O5/PVP) was produced using a hydrothermal method that involved the introduction of PVP into the preparation process. The resultants were characterized using FTIR, XRD, SEM, and TEM to confirm the phase composition, elemental composition, and bird’s nest morphology. This unique structure provides a micro-reactor for the photocatalytic reaction, which improves the reaction efficiency. The FTIR, XPS, and EPR tests demonstrated that the surface properties of Nb2O5 were altered by the introduction of PVP. This change caused an enhancement of the water adsorption capacity and the photocatalytic hydroxyl radical production yield. The results of the UV-DRS, PL, and EIS tests indicate that the energy band structure was altered by the PVP introduction. Additionally, the photogenerated charge fusion rate was reduced, the photogenerated charge mobility was improved, the reduction of photogenerated electrons was enhanced, and the photocatalytic yield of superoxide radicals was increased. The PVP introduction o extended the light absorption range of Nb2O5 from UV to visible light region, increasing light utilization efficiency. Photocatalytic performance was explored using methyl orange (MO), rhodamine 6B (RhB), and tetracycline (TC) as targets. Degradation experiments demonstrated that Nb2O5/PVP had significantly better catalytic activity than pristine Nb2O5.
Experimental procedure
Preparation of Nest-like Nb2O5
To prepare a homogeneous solution (referred to as solution A), 1 g of niobium oxalate was dissolved in 25 mL of distilled water and stirred at 60°C for 10 min. Then, another homogeneous solution (marked as solution B) was formed by adding 0.9 g of polyvinylpyrrolidone (PVP) to 10 mL of distilled water and stirring for 5 min. The B solution was pipetted drop-by-drop into the A solution while stirring to form a mixed solution. After stirring for 10 min, the mixed solution was confidently poured into a 50 mL hydrothermal reactor. The hydrothermal reactor was transferred to a baking oven and the reaction was conducted at 180°C for 12 h. The solution was confidently filtered after being cooled to room temperature. The final product was gained by drying the filter residue at 80°C for 24 h and grinding it.
Characterization method
The samples’ phase and surface chemical bonds were determined using X-ray diffractometry (TD-3500, Dandong Tongda, China) and Fourier transform infrared spectroscopy (Perkin Elmer L160000A, Massachusetts, U.S.A.). The samples’ morphology was observed using scanning electron microscopy (SEM) and transmission electron microscopy (TEM). SEM and TEM were performed with state-of-the-art equipment, including a FEI Gemini300 microscope from ZEISS in Oberkochen, Germany, and a FEI Tecnai G2 F20 microscope in Hillsboro, OR, U.S.A., respectively. The chemical compositions of the samples were measured using an INVIA Raman microprobe (Renishaw Instruments, Wotton-under-Edge, UK) and X-ray photoelectron spectra (XPS, ESCALAB 250Xi, TFS, MA, U.S.A.) with an excitation energy of 1486.6 eV of Al Kα. An ultraviolet-visible diffuse reflectance spectrophotometer (UV-Vis DRS, TU1901, Beijing, China) was utilized to investigate the optical properties of the samples. The samples’ specific surface area and pore structure were analysed using physisorption apparatus (BET, ASAP2460, Micromeritics, U.S.A.) after degassing at 200°C for 12 h. The photoluminescence (PL) spectra were recorded using the Full-functional Steady/Transient Fluorescence Spectrometer (FLS980, Edinburgh, UK). The JES FA200 (JEOL, Japan) spectrometer was used to detect the Electron Spin Resonance (ESR) signals of radical species captured by 5,5-dimethyl-1-pyrroline N-oxide (DMPO). Electrochemical Impedance Spectroscopy (EIS) tests were performed using a three-electrode system and a PP211 electrochemical workstation (Zahner). The working electrode was made of catalyst-modified glassy carbon (3 mm in diameter), the counter electrode was platinum foil, and the reference electrode was Ag/AgCl (saturated KCl). Electrochemical impedance spectroscopy (EIS) measurements were confidently taken at open circuit potential using an AC voltage with an amplitude of 5 mV over a frequency range of 10−1 to 105 Hz. The chosen frequency range was carefully selected to ensure accurate and reliable results.
Photocatalytic application
The photocatalytic activity of the samples was evaluated by degrading macromolecular organic matter (methyl orange (MO), rhodamine 6B (RhB), tetracycline (TC)) under simulated ultraviolet light irradiation (300 W Xenon lamp with visible cut-off filter) or simulated visible-light irradiation (300 W Xenon lamp with ultraviolet cut-off filter). The optical power density was measured at the beginning of the experiment using a light metre (900 mW·cm−2 for simulated ultraviolet light and 1000 mW·cm−2 for simulated visible-light). The liquid level to light source distance was maintained at a constant 20 cm throughout the test procedures. Initially, 100 mg of photocatalyst was added to a 100 mL solution containing 10 mg⸳L−1 RhB (MO, TC). The mixture was then fully stirred in the dark for 30 min to achieve adsorption equilibrium. The UV-Vis spectrophotometer (UV-Vis DRS, TU1901, Beijing, China) was used to measure the absorption of a series of reaction solutions (MO 463 nm, RhB 552 nm, and TC 356 nm) at specific irradiation time intervals. The photodegradation rate was calculated as C/C0, where C0 is the initial concentration of the reaction solution and C is the concentration of the reaction solution after at different reaction times.
Results and discussion
Phase and structure
As shown in , there are Nb-O bond and Nb-O-Nb bond stretching vibration peaks at 520 cm−1 to 820 cm−1 with coupling [Citation16], while the characteristic absorption peaks between 1500 cm−1 and 2500 cm−1 correspond to the bending vibration of adsorbed H2O [Citation21,Citation22], and the characteristic absorption peaks between 3400 cm−1 and 3500 cm−1 belong to the hydroxyl group of the sample adsorbed H2O (−OH) stretching vibration [Citation21,Citation23]. These phenomena were observed in both samples. On the one hand, the presence of Nb2O5 is confirmed; on the other hand, the addition of PVP did not change the basic chemical bonding of Nb2O5 during the preparation. The peak intensity of adsorbed water is larger for Nb2O5/PVP than for pristine Nb2O5, indicating that Nb2O5/PVP adsorbs water more easily, which is favourable for the photocatalytic reaction.
Figure 1. FTIR spectra (a) and XRD patterns (b) of samples. The high-resolution XPS of Nb and O elements in Nb2O5/PVP sample.
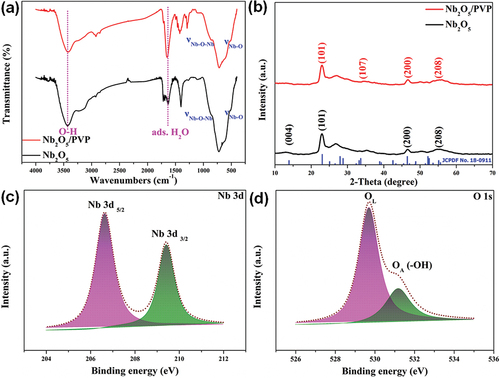
The diffraction peaks of Nb2O5 and Nb2O5/PVP were observed at 2Ɵ = 23.022°, 33.626°, 46.483°, and 55.114°, corresponding to crystal faces (101), (107), (200), and (208) [Citation16,Citation20] (). The peak intensity and sharpness of the characteristic peaks were similar, which confirms that the introduction of PVP did not affect the crystallinity or introduce any new impurities.
In , the high-resolution XPS spectra of Nb and O elements are displayed. These results demonstrate a clear understanding of the chemical composition and properties of the sample. The binding energies of Nb 3d5/2 and Nb 3d3/2 were measured at 206.64 eV and 209.41 eV, respectively [Citation15,Citation24]. Additionally, the binding energy of lattice oxygen and adsorbed oxygen were observed at 529.69 eV and 531.18 eV, respectively [Citation25–27].
In summary, it can be confidently stated that the FTIR, XRD, and XPS tests indicate the successful acquisition of high-purity niobium oxide.
The SEM image of Nb2O5 in () displays irregular morphology and severe agglomeration. However, Nb2O5/PVP exhibits a regular birdcage-like structure with uniformly distributed internal pores, as shown in (. These observations are confirmed by the TEM images in (. The birdcage-like structure facilitates an increase in the specific surface area of the sample. The lattice spacing was determined through Fourier and inverse Fourier transforms on the HRTEM. The lattice spacing of 0.3863 nm is confidently attributed to the (101) crystal plane of Nb2O5 [Citation15,Citation24], providing evidence that the preparation of Nb2O5 was successful. Furthermore, it can be diplomatically stated that the introduction of PVP did not alter its intrinsic crystal structure.
Figure 2. SEM images of Nb2O5 (a) and Nb2O5/PVP (b, c). TEM (d, e) and HRTEM (f) images of Nb2O5/PVP.
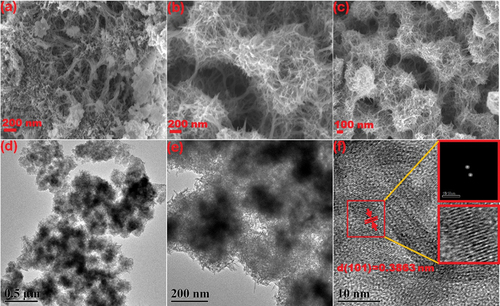
The specific surface area of pristine Nb2O5 and Nb2O5/PVP was 194.1 m2/g and 200.2 m2/g, respectively (as shown in ). The average pore size distribution of pristine Nb2O5 and Nb2O5/PVP was 8.9847 nm and 6.4221 nm, respectively (as shown in ). These results confirm our initial hypothesis. Furthermore, both pure Nb2O5 and Nb2O5/PVP samples can be classified as mesoporous materials due to their average pore size distribution falling between 2 nm and 50 nm [Citation28,Citation29]. The relationship between p/p0 and the thickness of the adsorption layer is illustrated in . The thickness of the adsorbed layer increases as p/p0 increases, indicating multilayer adsorption in numerous mesopores within the samples [Citation27,Citation28]. This finding is consistent with the deduction made from the pore size distribution. The PVP introduction did not alter the surface structure of Nb2O5. This is evidenced by the same multilayer adsorption phenomenon and the Type-II isothermal adsorption curve (as shown in ).
Multifunctional photocatalytic application
The photocatalytic activity of the as-prepared samples under visible light was evaluated using simulated dye wastewater solutions of MO and RhB. It is worth noting that neither MO nor RhB underwent self-degradation during the degradation process. As shwon in , under visible light irradiation, Nb2O5 and Nb2O5/PVP demonstrated degradation efficiencies of 33.6% and 75.0% for MO, respectively. The corresponding reaction kinetics were 0.0797 min−1 and 0.05811 min−1. The rate constant for MO degradation by Nb2O5/PVP was found to be 5.94 times higher than that of conventional Nb2O5, indicating a faster and more efficient degradation process. This could potentially result in cost savings for future applications. These results demonstrate the superior performance of Nb2O5/PVP and its potential for practical use in various applications. Additionally, under visible light irradiation, RhB degradation efficiency was observed to be 94.5% for Nb2O5 and 100.0% for Nb2O5/PVP after only 10 min, with corresponding reaction kinetics of 0.20435 min−1 and 0.30812 min−1. The rate constant for RhB degradation by Nb2O5/PVP was 1.51 times higher than that of conventional Nb2O5, indicating faster and more efficient reaction. This suggests that the use of Nb2O5/PVP could potentially result in cost savings in application.
Figure 4. The photodegradation reaction of MO/RhB using Nb2O5 and Nb2O5/PVP and the associated reaction kinetics.
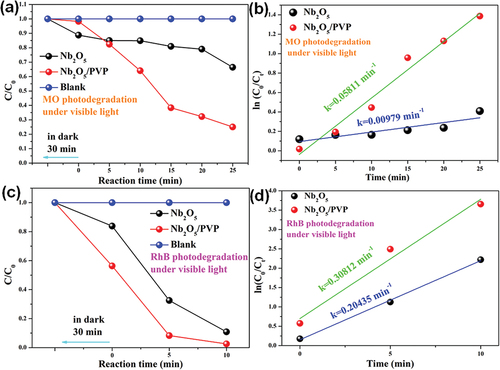
The photocatalytic properties of Nb2O5/PVP were validated through the photodegradation of TC antibiotic solutions. As shown in , the degradation efficiency of TC over Nb2O5/PVP was found to be 90.4% after a 25 min reaction, with a rate constant of 0.0824 min−1. These results demonstrate the high effectiveness of Nb2O5/PVP as a photocatalyst for the TC degradation. Among the reported photocatalytic materials for the TC degradation under visible light, Nb2O5/PVP exhibits the highest degradation efficiency and fastest reaction rate, as demonstrated in . Meanwhile, the literatures have shown that TiO2 is time consuming and has low reaction efficiency in the degradation of organic matter [Citation41–43]. This confirms that the catalytic performance of Nb2O5/PVP is superior to TiO2. These findings suggest that Nb2O5/PVP holds great potential as a catalytic material for environmental treatment.
Table 1. Comparison of visible-light-driven photocatalysis for TC degradation.
The study evaluated the reuse performance of Nb2O5/PVP in degrading RhB solution under visible light irradiation. The results revealed that Nb2O5/PVP has high stability and reusability, as the degradation efficiency of RhB remained almost unchanged after three reuses (). Additionally, complete degradation of RhB was achieved. These findings demonstrate the prepared photocatalytic material exhibits high catalytic stability and good reusability.
Enhancement mechanism of photocatalysis
The UV-Vis DRS spectra are shown in . The absorption range of Nb2O5 is restricted to the UV range because of electron transitions from the valence band, primarily composed of O (2s orbits) and Nb (4s and 4p orbits), to the conduct band, primarily composed of O (2p orbits) and Nb (4d and 5s orbits). Moreover, it is worth noting that Nb2O5/PVP also exhibits visible light absorption. The statement suggests that the introduction of PVP broadens the absorption range of Nb2O5 from the UV light regime to the visible light regime. The band gap energy (Eg) values of Nb2O5 and Nb2O5/PVP were calculated to be 2.98 eV and 2.62 eV, respectively, which is consistent with previous deductions. Therefore, the addition of PVP decreases the forbidden bandwidth value and enhances the absorption intensity of Nb2O5 in the visible light regime.
Figure 7. The UV-vis DRS spectra of Nb2O5 (a) and Nb2O5/PVP (b), (Inset: The related band gap energy); PL spectra (c) and EIS spectra (d) of Nb2O5 and Nb2O5/PVP.
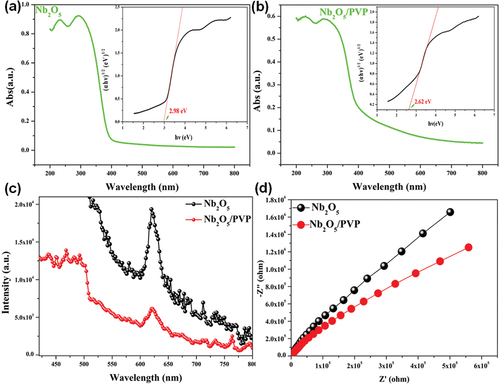
It is widely accepted that photoluminescence (PL) signals arise from the recombination of photogenerated carriers in semiconductor upon exposure to light. The intensity of the PL signals is directly proportional to the recombination rate of the carriers [Citation44]. The recombination rate of photogenerated electrons and holes is directly proportional to PL signals. Therefore, higher PL signals indicate a higher recombination rate, while lower PL signals indicate a lower recombination rate [Citation23,Citation45]. The photoluminescence (PL) signals were generated through a photoexcitation process. Electrons from the valence band were excited to the conduction band, thereby forming excited state electrons in the conduction band and holes in the valence band [Citation26,Citation46]. Some of the unstable excitation electrons relax from the conduction band to the valence band, leading to recombination with photoinduced holes, while releasing energy in the form of fluorescence or phosphorescence. This observation suggests that Nb2O5/PVP may have a lower concentration of excited electrons and holes, which could be due to the presence of PVP in the composite material. In the visible light region (), it was observed that the PL signal intensity of Nb2O5/PVP was lower than that of pristine Nb2O5. It can be confidently stated that in the visible light region, the carrier recombination rate of Nb2O5/PVP was lower than that of Nb2O5. It is important to note that these results were obtained through careful experimentation and analysis. Furthermore, the carrier separation efficiency of Nb2O5/PVP was found to be higher than that of Nb2O5.
The catalyst’s charge transport ability is determined by analysing the semicircle in the high-frequency section of the Nyquist curve. A smaller semicircle radius indicates lower impedance and better charge transport capacity [Citation44,Citation46]. It can be observed from () that the semicircular radius of Nb2O5/PVP is smaller in the high-frequency regime than that of bare Nb2O5. This indicates that the introduction of PVP improves the charge transport ability of Nb2O5, thereby facilitating the separation of photogenerated electrons and holes
Based on the experimental results presented above, it is clear that the catalytic activity of Nb2O5/PVP is higher than that of Nb2O5.
The study confidently employed DMPO spin trapping and ESR technology to examine active species and their yields, while diplomatically investigating the mechanism underlying the enhancement of photocatalytic activity. These findings provide valuable insights into the photocatalytic process. The ESR signal intensity ratio of the 1:1:1:1:1 quartet characteristic was attributed to the DMPO-·O2− spin adduct [Citation25,Citation27,Citation45], while the intensity ratio of the 1:2:2:1 quartet characteristic ESR signal was attributed to the DMPO-·OH spin adduct [Citation25,Citation44,Citation47]. The generation of ·O2− and ·OH occurs via one-step reduction and oxidation reactions between photoinduced electrons and absorbed oxygen, and photoinduced holes and absorbed water, respectively. The ESR spectra demonstrate that all samples are capable of generating ·O2− and ·OH under ultraviolet visible light. Notably, the gsignal intensity of Nb2O5/PVP is stronger than that of Nb2O5. These results confidently suggest that Nb2O5/PVP is a promising candidate for photocatalytic applications, while Nb2O5 also exhibits significant potential. The introduction of PVP in Nb2O5/PVP significantly increased the amount of ·OH and ·O2− by 5.32 and 4.15 times, respectively, compared to Nb2O5 alone (as shown in and ). This demonstrates the clear enhancement of water adsorption on the niobium oxide surface and the narrowing of the forbidden band width by PVP, resulting in a substantial increase in the reduction of photogenerated electrons.
Figure 8. DMPO spin-trapping ESR spectra in Nb2O5 and Nb2O5/PVP in dark or under ultraviolet visible light irradiation: (a) and (b) in aqueous dispersion for DMPO-·OH, (c) and (d) in methanol dispersion for DMPO-·O2−.
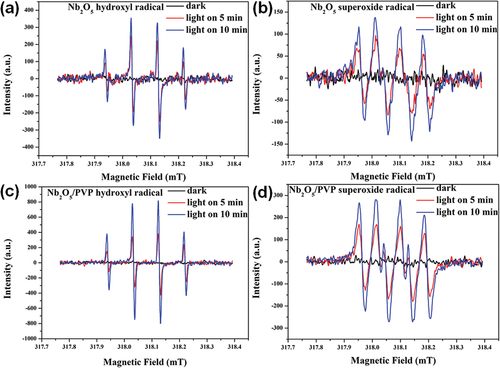
Table 2. The intensity value of free radical signal and the related square value from ESR test.
In summary, the reaction mechanism can be described as follows. When Nb2O5/PVP is exposed to light radiation, electrons in the valence bands of O (2s orbits) and Nb (4s and 4p orbits) are excited when the energy of the photons is greater than the bandgap energy, resulting in a jump to the conduction bands of O (2p orbits) and Nb (4d and 5s orbits) and the formation of photogenerated electrons with strong reducing ability (EquationEquation 1(1)
(1) ) [Citation29,Citation44,Citation46]. After the electrons are excited, they jump and form photogenerated holes in the valence band with strong oxidizing ability (EquationEquation 1
(1)
(1) ) [Citation29,Citation44,Citation46]. Photogenerated electrons and dissolved oxygen undergo a one-electron reduction reaction to produce superoxide radicals (EquationEquation 2
(2)
(2) ) [Citation21,Citation48]. Photogenerated electrons, dissolved oxygen, and adsorbed water undergo a five-electron reaction to form hydroxyl radicals (EquationEquation 3
(3)
(3) ) [Citation48]. Photogenerated holes and adsorbed water undergo an oxidation reaction via the one-hole pathway to produce hydroxyl radicals (EquationEquation 4
(4)
(4) ) [Citation21,Citation48]. The generated hydroxyl and superoxide radicals, photogenerated electrons and holes combine to attack the fragile chemical bonds of the organic matter, ultimately breaking down the large organic molecules into small, non-toxic inorganic molecules (EquationEquation 5
(5)
(5) ).
Conclusion and outlooks
A bird’s nest-like structure of Nb2O5 (Nb2O5/PVP) was synthesized using a hydrothermal method that introduced PVP during preparation. This unique structure acts as a micro-reactor for photocatalytic reactions, improving reaction efficiency. Additionally, PVP serves multiple functions, including altering the surface properties of Nb2O5, enhancing water adsorption, and increasing the yield of hydroxyl radicals in photocatalysis. It also modifies the energy band structure, reduces the rate of photogenerated charge fusion, improves photogenerated charge mobility, enhances photogenerated electron reduction, and improves the yield of superoxide radicals in photocatalysis. The addition of PVP expands the light absorption range of Nb2O5 from UV light to visible light, increasing light utilization efficiency. Nb2O5/PVP achieved photocatalytic degradation of RhB in only 10 min, and tetracycline degradation efficiency reached 90.4% in only 25 min. Therefore, we hope that researchers will widely investigate the properties of Nb2O5/PVP and its potential for environmental applications.
Acknowledgments
This work was supported by Chongqing Basic Science Advanced Technology Research Program (CSTB2022NSCQ-MSX1397), Science and Technology Research Program of Chongqing Municipal Education Commission (KJZD-M202301301), and funds for the introduction of talent from Chongqing Preschool Education College.
Disclosure statement
No potential conflict of interest was reported by the author(s).
References
- Wang S, Wang L, Huang W. Bismuth-based photocatalysts for solar energy conversion. J Mater Chem A. 2020;8(46):24307–10. doi: 10.1039/D0TA09729B
- Huang N-Y, Zheng Y-T, Chen D, et al. Reticular framework materials for photocatalytic organic reactions. Chem Soc Rev. 2023;52(22):7949–8004. doi: 10.1039/D2CS00289B
- Ravelli D, Dondi D, Fagnoni M, et al. Photocatalysis. A multi-faceted concept for green chemistry. Chem Soc Rev. 2009;38(7):1999–2011. doi: 10.1039/b714786b
- Guo Q, Zhou C, Ma Z, et al. Elementary photocatalytic chemistry on TiO2 surfaces. Chem Soc Rev. 2016;45(13):3701–3730. doi: 10.1039/C5CS00448A
- Mai H, Chen D, Tachibana Y, et al. Developing sustainable, high-performance perovskites in photocatalysis: design strategies and applications. Chem Soc Rev. 2021;50(24):13692–13729. doi: 10.1039/D1CS00684C
- Liu C, Liu H, Yu JC, et al. Strategies to engineer metal-organic frameworks for efficient photocatalysis. Chin J Catalysis. 2023;55:1–19. doi: 10.1016/S1872-2067(23)64556-5
- Zhou L, Li B, Li J, et al. Simultaneous triple-effect on inhibiting the photogenerated carriers recombination of g-C3N4 for boosting solar H2 production at atmospheric pressure. J Environ Chem Eng. 2023;11(3):109975. doi: 10.1016/j.jece.2023.109975
- Wei Z, Liu J, Shangguan W. A review on photocatalysis in antibiotic wastewater: pollutant degradation and hydrogen production. Chin J Catalysis. 2020;41(10):1440–1450. doi: 10.1016/S1872-2067(19)63448-0
- Zheng Y, Pan Z, Wang X. Advances in photocatalysis in China. Chin J Catalysis. 2013;34(3):524–535. doi: 10.1016/S1872-2067(12)60548-8
- Devi LG, Kavitha R. A review on non metal ion doped titania for the photocatalytic degradation of organic pollutants under UV/solar light: role of photogenerated charge carrier dynamics in enhancing the activity. Appl Catal B Environ. 2013;140-141:559–587. doi: 10.1016/j.apcatb.2013.04.035
- Chen P, Zhou M, Liu Y, et al. Carbon nitride in peroxide-coupled photocatalysis for aqueous organic pollutants destruction: engineered active sites and electron transfer regimes. Appl Catal B: Environ Eng. 2024;346:123767. doi: 10.1016/j.apcatb.2024.123767
- Wang H, Li X, Zhao X, et al. A review on heterogeneous photocatalysis for environmental remediation: from semiconductors to modification strategies. Chin J Catalysis. 2022;43(2):178–214. doi: 10.1016/S1872-2067(21)63910-4
- Likodimos V. Photonic crystal-assisted visible light activated TiO2 photocatalysis. Appl Catal B Environ. 2018;230:269–303. doi: 10.1016/j.apcatb.2018.02.039
- Su H, Yin H, Wang R, et al. Atomic-level coordination structures meet graphitic carbon nitride (g-C3N4) for photocatalysis: energy conversion and environmental remediation. Appl Catal B Environ. 2024:123683. doi: 10.1016/j.apcatb.2023.123683
- Liao W, Yang Z, Wang Y, et al. Novel Z-scheme Nb2O5/C3N5 photocatalyst for boosted degradation of tetracycline antibiotics by visible light-assisted activation of persulfate system. Chem Eng J. 2023;478:147346. doi: 10.1016/j.cej.2023.147346
- Zhang P, Peng C, Li H, et al. Wavelength-dependent generation of reactive species in the photodegradation process over pure and C-doped Nb2O5. Sep Purif Technol. 2022;286:120406. doi: 10.1016/j.seppur.2021.120406
- Wu T, Ma B, Bai H, et al. Ligand-induced reaction mechanism regulation on Sr/Nb2O5 for high-efficiency selective photocatalytic NO oxidation. Appl Catal B: Environ Eng. 2024;345:123688. doi: 10.1016/j.apcatb.2023.123688
- Su K, Wang Y, Zhang C, et al. Tuning the Pt species on Nb2O5 by support-induced modification in the photocatalytic transfer hydrogenation of phenylacetylene. Appl Catal B Environ. 2021;298:120554. doi: 10.1016/j.apcatb.2021.120554
- Peng C, Xie X, Xu W, et al. Engineering highly active Ag/Nb2O5@Nb2CTx (MXene) photocatalysts via steering charge kinetics strategy. Chem Eng J. 2021;421:128766. doi: 10.1016/j.cej.2021.128766
- Abdellatif HRS, Zhang G, Tang Y, et al. A highly efficient dual-phase GaN(O)/Nb2O5(N) photocatalyst prepared through nitridation and reoxidation process for NO removal. Chem Eng J. 2020;402:126199. doi: 10.1016/j.cej.2020.126199
- Zhang L, Yang J, Xie T, et al. Boosting visible-light-driven photocatalytic activity of BiPO4 via constructing Schottky junction with Ti3C2 MXene. Mater Design. 2020;192:108772. doi: 10.1016/j.matdes.2020.108772
- Yang J, Xie T, Zhu Q, et al. Boosting the photocatalytic activity of BiOX under solar light via selective crystal facet growth. J Mater Chem C. 2020;8(7):2579–2588. doi: 10.1039/C9TC05752H
- Yang J, Zhu Q, Xie Z, et al. Enhancement mechanism of photocatalytic activity for MoS2/Ti3C2 Schottky junction: experiment and DFT calculation. J Alloys Compd. 2021;887:161411. doi: 10.1016/j.jallcom.2021.161411
- Song T, Wang C, Zhang Y, et al. Visible-light-induced oxidative alkene difunctionalization to access α-sulfonyloxy ketones catalyzed by oxygen-vacancy-rich Nb2O5. Appl Catal B Environ. 2022;304:120964. doi: 10.1016/j.apcatb.2021.120964
- Yu D, He J, Xie T, et al. Boosting catalytic activity of SrCoO2.52 perovskite by Mn atom implantation for advanced peroxymonosulfate activation. J Hazard Mater. 2023;442:130085. doi: 10.1016/j.jhazmat.2022.130085
- Feng S, Yu M, Xie T, et al. MoS2/CoFe2O4 heterojunction for boosting photogenerated carrier separation and the dominant role in enhancing peroxymonosulfate activation. Chem Eng J. 2022;433:134467. doi: 10.1016/j.cej.2021.134467
- Xie T, Chen B, Mei Y, et al. Ultrafast degradation of tetracycline by PMS activation over perfect cubic configuration MnCo2O4.5: new insights into the role of metal-oxygen bonds in PMS activation. Sep Purif Technol. 2023;315:123694. doi: 10.1016/j.seppur.2023.123694
- Xie T, Xu L, Liu C, et al. Synthesis and adsorption properties of high specific surface area strontium ferrite from industrial strontium residue. Vacuum. 2013;93:71–78. doi: 10.1016/j.vacuum.2013.01.005
- Xie T, Xu L, Liu C, et al. Magnetic composite ZnFe2O4/SrFe12O19: preparation, characterization, and photocatalytic activity under visible light. Appl Surface Sci. 2013;273:684–691. doi: 10.1016/j.apsusc.2013.02.113
- Hu X, Lu P, Fu M, et al. Activating the photocatalytic activity of insulator barium silicate: a liquid-phase alkalized tetracycline photosensitizer and its self-destruction. Chem Eng J. 2023;454:140281. doi: 10.1016/j.cej.2022.140281
- Hosseini O, Zare-Shahabadi V, Ghaedi M, et al. Experimental design, RSM and ANN modeling of tetracycline photocatalytic degradation using LDH@CN. J Environ Chem Eng. 2022;10(5):108345. doi: 10.1016/j.jece.2022.108345
- Zhang Y, Zhang Q, Xing K, et al. Construction of Z-scheme heterojunction LaCoO3 modified ZnO for photocatalytic degradation of tetracycline under visible light irradiation. Catal Commun. 2024;186:106815. doi: 10.1016/j.catcom.2023.106815
- Shashikant, Dutta RK. A mechanistic study on enhanced solar photocatalytic activity of SnWO4/BiMoO6 nanocomposites for degradation of tetracycline. Inorg Chem Commun. 2023;158:111576. doi: 10.1016/j.inoche.2023.111576
- Fan J, Bai M, Zhang H, et al. Synthesis and immobilization of HC/BiVO4 catalyst particles with PTFE for photocatalytic tetracycline degradation: preparation, performance and mechanism. J Water Process Eng. 2023;56:104474. doi: 10.1016/j.jwpe.2023.104474
- Hou S, Gao Y, Song P, et al. Synthesis of Pd-modified Fe3O4 loaded on montmorillonite catalyst for photocatalytic degradation of tetracycline. Inorg Chem Commun. 2024;159:111745. doi: 10.1016/j.inoche.2023.111745
- He Y, Xia J, Ye J, et al. Design of Co-doped carbon nitride based on melem supramolecular assembly with enhanced photocatalytic activity toward tetracycline hydrochloride degradation. Mater Sci Semicond Process. 2024;171:107999. doi: 10.1016/j.mssp.2023.107999
- Dharman RK, Oh TH. Fabrication of g-C3N4@N-doped Bi2MoO6 heterostructure for enhanced visible-light-driven photocatalytic degradation of tetracycline pollutant. Chemosphere. 2023;338:139513. doi: 10.1016/j.chemosphere.2023.139513
- Jiang R, Lu G, Zhang L, et al. Insight into the effect of microplastics on photocatalytic degradation tetracycline by a dissolvable semiconductor-organic framework. J Hazard Mater. 2024;463:132887. doi: 10.1016/j.jhazmat.2023.132887
- Ni Q, Ke X, Qian W, et al. Insight into tetracycline photocatalytic degradation mechanism in a wide pH range on BiOI/BiOBr: coupling DFT/QSAR simulations with experiments. Appl Catal B Environ. 2024;340:123226. doi: 10.1016/j.apcatb.2023.123226
- Mondal S, Dilly Rajan K, Rathinam M, et al. Enhanced photocatalytic degradation of tetracycline using NiCo–BiVO4 nanocomposite under visible light irradiation: A noble-metal-free approach for water remediation. Chemosphere. 2024;350:141012. doi: 10.1016/j.chemosphere.2023.141012
- Wang J, Ye N, Yan X, et al. Sb2WO6 nanoparticles coated TiO2 nanobelts exhibiting remarkable photo-catalyst response. Mater Technol. 2018;33(7):479–487. doi: 10.1080/10667857.2018.1463686
- He Y, He Q, Liu Z, et al. Controllable preparation and improved performance of TiO2 photocatalysts with various structures. Mater Technol. 2020;35(1):1–10. doi: 10.1080/10667857.2019.1644038
- Shi Y, Li J, Yu Z, et al. Investigation of enhanced photocatalytic performance of europium doped TiO2 film. Mater Technol. 2021;36(9):552–563. doi: 10.1080/10667857.2020.1777796
- Feng S, Xie T, Wang J, et al. Photocatalytic activation of PMS over magnetic heterojunction photocatalyst SrTiO3/BaFe12O19 for tetracycline ultrafast degradation. Chem Eng J. 2023;470:143900. doi: 10.1016/j.cej.2023.143900
- He Y, Qian J, Wang P, et al. Synergized selenium-vacancy heterogeneous interface and carbon nanotubes for insight into efficient oxidation of pollutants via photocatalytic peroxymonosulfate activation. Appl Catal B Environ. 2023;330:122620. doi: 10.1016/j.apcatb.2023.122620
- Yang J, Xie T, Mei Y, et al. High-efficiency V-Mediated Bi2MoO6 photocatalyst for PMS activation: modulation of energy band structure and enhancement of surface reaction. Appl Catal B Environ. 2023;339:123149. doi: 10.1016/j.apcatb.2023.123149
- Xiao Y, He J, An J, et al. Highly efficient activation of peroxymonosulfate by ZIF-67 anchored cotton derived for ciprofloxacin degradation. Environ Res. 2024;244:117863. doi: 10.1016/j.envres.2023.117863
- Yang J, Zhu Q, Xie T, et al. Insights into interface charge extraction in a noble-metal-free doped Z-scheme NiO@BiOCl heterojunction. Catalysts. 2020;10(9):958. doi: 10.3390/catal10090958