Abstract
This study evaluated thiolated poly(acrylic acid) nanoparticles as a valuable tool to protect insulin from degradation by serinproteases of the intestine. Nanaoparticles were characterized concerning particle size, zeta potential, and drug load. Furthermore, in vitro release studies were performed. Within in vitro degradation studies with trypsin, α-chymotrypsin, and elastase it could be demonstrated that the obtained nanoparticles are capable of protecting 44.47 ± 0.89% of the initial insulin amount from tryptic degradation, 21.33 ± 5.34% from chymotryptic degradation, and 45.01 ± 1.40% from degradation by elastase compared to insulin solutions.
Introduction
Rapid development in recombinant DNA techniques and biotechnology increases the number of therapeutic protein and peptide drugs. At present, most of these drugs have to be administered via the parenteral route. This form of application is often inconvenient for patients due to pain, fear, and risk of infections. Consequently, orally administered dosage forms for peptide and protein drugs are highly on demand. However, the oral bioavailability of most peptide drugs, such as insulin, is strongly limited since several barriers like the diffusion barrier of the mucus gel layer (CitationBernkop-Schnürch & Fragner, 1996), the absorption barrier of gastrointestinal epithelia (CitationPusztai, 1989), and the enzymatic barrier (CitationWoodley, 1994) hamper the systemic uptake of these drugs. Orally administered insulin, for example, undergoes degradation by several intestinal proteases like trypsin, α-chymotrypsin (CitationSchilling & Mitra, 1991), and elastase (CitationNarayanan & Anwar, 1969) in the gastrointestinal tract (GIT). Hence, a carrier system for oral peptide administration should include inhibitory properties towards these digestive enzymes.
As a result of their prolonged residence time on mucosal membranes (CitationCoupe, Davis, & Wilding, 1991) and their capacity to reach a greater mucosal surface area leading to a comparatively higher drug uptake (CitationPonchel & Irache, 1998), nanoparticles have been widely investigated as carrier systems for oral peptide and protein delivery. Furthermore, it was reported by several work groups that a protective effect towards proteolytic enzymes could be achieved for peptides and proteins by incorporating them into nanoparticles. Nanoparticulate oral delivery systems are based on various polymers, such as poly(lactic-co-glycolic acid) (CitationCui et al., 2007), chitosan (CitationBodmeier, Chen, & Paeratakul, 1989), and poly(acrylic acid) (PAA) (CitationKriwet, Walter, & Kissel, 1998). Among these polymers, poly(acrylic acid) exhibits a remarkably strong inhibitory effect on various luminal proteolytic enzymes (CitationBai, Chang, & Guo, 1996). Additional immobilization of thiol groups to the backbone of PAA leads to improved enzyme inhibitory properties and allows cross-linking within the nanoparticles by oxidizing thiol groups to disulfide bonds. Moreover, thiolation of polyacrylates allows a further increase in oral bioavailability by improving the mucoadhesive (CitationMarschütz & Bernkop-Schnürch, 2002) and permeation enhancing (CitationPalmberger et al., 2007) capacities of PAA.
Since the enzymatic barrier has been identified to be a major obstacle for oral peptide and protein delivery, it was the aim of this study to develop a drug carrier system based on thiolated PAA in order to benefit from its enzyme inhibitory potential besides the other mentioned advantages.
Insulin-containing nanoparticles were synthesized with a simple nanoprecipitation technique which is based on the formation of interpolymer complexes between PAA and the non-ionic proton-acceptor poly(vinyl pyrrolidon) (PVP) (CitationNurkeeva et al., 2003). This technique does not rely on high temperatures which are needed for solvent evaporation techniques and could harm peptides and proteins. Non-cross-linked particles are stable at pH-values below 4.5. Therefore, intra- and intermolecular disulfide bonds were formed by means of oxidation to stabilize the nanoparticles at a broader pH-range. The obtained nanoparticles were finally incorporated into a triglyceride.
In order to investigate the protective potential of this formulation, in vitro enzyme degradation studies with trypsin, α-chymotrypsin, and elastase were performed with these solid dispersions of insulin-containing nanoparticles, insulin solutions, and insulin dispersions in the triglyceride without previous encapsulation.
Material and methods
Materials
All chemicals except Kollidon 12 PF (BASF, Ludwigshafen, Germany) and mannitol (Gatt-Koller, Absam, Austria) were purchased from Sigma-Aldrich (Steinheim, Germany).
Synthesis of thiolated poly(acrylic acid)
Thiolated PAA was synthesized according to a method previously described by our research group (CitationMarschütz & Bernkop-Schnürch, 2002). Briefly, 1 g of PAA with a molecular mass (MM) of 100,000 kDa was first hydrated in 100 ml demineralized water and the pH was adjusted to 5.8 with 5 M NaOH. One gram of 1-ethyl-3-(3-dimethylaminopropyl) carbodiimide (EDAC) was added to activate the carboxylic acid moieties of the hydrated polymers. After 15 min of incubation under magnetic stirring 1 g of L-cysteine hydrochloride was added and the pH was readjusted to 5.8. The reaction mixture was incubated for further 3 h at room temperature under magnetic stirring. Finally, the conjugate (PAA100-Cys) was dialysed twice against 0.2 mM HCl, twice against 0.2 mM HCl containing 1% NaCl, and finally twice against 0.2 mM HCl. Subsequently, the polymer was freeze-dried at −70°C and 0.01 mbar (Benchtop 2K, VirTis, NY, USA) and stored at 4°C until further use.
Characterization of PAA-Cys conjugates
The amount of thiol groups on the conjugates was determined with Ellman’s reagent [DTNB, 5,5’-dithio-bis(2-nitrobenzoic acid)] as described previously (CitationBernkop-Schnürch, Schwarz, & Steininger, 1999). Disulfide content was measured after reduction with NaBH4 and subsequent addition of DTNB (CitationHabeeb, 1973). Moreover, the content of free L-cysteine in the lyophilized product was determined with 2,4,6-trinitrobenzene-1-sulfonic acid (TNBS) in order to assess purity of the conjugate (CitationSatake et al., 1960).
Preparation of thiolated PAA nanoparticles
For the preparation of nanoparticles PAA-Cys (MM 100,000 kDa) was chosen since preliminary studies have shown that this MM delivers the most stable nanoparticles concerning agglomeration and precipitation. Fifty milligrams of PAA100-Cys were hydrated in 50 mL demineralized water, pH was adjusted to 2.0, and 4 mg of insulin were added. The amount of insulin was chosen because, at this PAA100-Cys to insulin ratio, 100% of the employed insulin could be incorporated into nanoparticles within preliminary studies and consequently it was possible to omit further purification steps. Afterwards, 750 μL of a 1% PVP-solution (Kollidon 12 PF) were added dropwise under magnetic stirring. As a result, nanoparticles were formed spontaneously. Iodine solution (0.05 M) was added to oxidize the nanoparticles for further stabilization. The volume of iodine was chosen according to the amount of thiol groups on the polymer in order to reach complete oxidation of thiol groups. The nanoparticle suspension was freeze-dried at −70°C and 0.01 mbar with 1% mannitol as a cryoprotectant. Subsequently, the product was stored at 4°C until further use.
In preliminary studies with the prepared nanoparticles burst release profiles were observed. In order to avoid such a burst release of insulin from the nanoparticles the lyophilized product was dispersed in 1.5 g of a molten triglyceride with a hydroxyl number of 45–60 mg KOH/g under vigorous magnetic stirring. The suspension was soaked up into a plastic tube with a syringe. After solidification the dispersions were cleaved into pieces of ~120 mg.
Particle characterization
Size distribution and zeta potential of particles were measured by means of photon correlation spectroscopy using a PSS NICOMP™ 380 DLS/ZLS (Santa Barbara, CA). Zeta potential had to be measured prior to the oxidation because of electrochemic reactions of iodine.
For the determination of the drug load nanoparticles without cryoprotectant were prepared. Five milligrams of the lyophilized product were re-suspended and exhaustively extracted in 10 ml 1 M HCl. After subsequent centrifugation the insulin content was determined via a previously described HPLC method (CitationMarschütz & Bernkop-Schnürch, 2000). Insulin concentrations were derived from a calibration curve which was obtained from seven different concentrations of insulin dissolved in 1 M HCl.
HPLC analysis of insulin
HPLC analysis was performed according to a method previously described by our research group. In brief, insulin was separated from its degradation products on a Nucleosil 100-5 C18 column (250 × 4 mm) at 40°C with gradient elution (1 ml/min): 0–22 min; linear gradient; from 91% A/9% B to 39% A/61% B (eluent A: 0.1% triflour-acetic acid; eluent B: acetonitrile). Detection was carried out with a diode array absorbance detector at 220 nm. The observed retention time for insulin was 16.4 min.
In vitro release studies
For the examination of the insulin release profile, 120 mg of the solid nanoparticle dispersion were placed in 1 ml of a buffer solution containing 1.36 g of Na2HPO4, 0.22 g of KH2PO4, 8.5 g of NaCl, and 5 mmol EDTA (pH 6.8) per liter. Tween 80 was added in a final concentration of 0.05%, which is above the critical micelle concentration in order to reduce surface adsorption of insulin (CitationMollmann et al., 2005), whereas EDTA was added to improve insulin solubility at the given pH. EDTA can reduce the formation of insulin hexamers due to complexation of zinc ions (CitationJames et al., 1981). After 0, 15, 30, 60, 120, 180, and 360 min the sample was centrifuged at 37°C and the aqueous phase was separated and stored at 4°C until further analysis. In order to measure the complete insulin content, 120 mg of the dispersion were extensively extracted with 1 M HCl, and the aqueous phase was obtained by centrifugation. The collected aqueous samples were then analyzed for their insulin content by the HPLC method mentioned above.
Enzymatic degradation studies
Degradation studies were performed according to a slightly modified method, as described previously by our research group (CitationWerle, Samhaber, & Bernkop-Schnürch, 2006).
Enzyme solutions containing 3.1 IU trypsin and 6.6 BTEE U α-chymotrypsin, respectively, were prepared in 250 μL in TRIS buffer (50 mM, pH 6.8). Elastase was first dissolved in 1% KCl and subsequently diluted with TRIS buffer (50 mM, pH 6.8) to obtain an activity of 0.3 (Suc Ala3NA) U elastase per mL. Insulin was dissolved in a buffer solution containing 1.36 g of Na2HPO4, 0.22 g of KH2PO4, 8.5 g of NaCl, 5 mmol EDTA per liter (pH 6.8), and 0.05% Tween 80 to obtain a final concentration of 0.1 mg/mL; 250 μL of the insulin solution was added to each enzyme solution containing intestinal proteases (250 μl). The reaction mixtures were incubated at 37°C under shaking (500 rpm). The enzymatic reaction was stopped at predetermined time points (0, 5, 15, 30, 60, 120, 180 min) due to the addition of 500 μl of 0.5% trifluor-acetic acid (TFA). For the reaction mixture containing α-chymotrypsin different time intervals were chosen due to the rapid degradation (0, 1, 2, 5, 15, 30, 60 min) observed in plain insulin solutions. The samples were stored at 4°C until they were analyzed by HPLC.
One hundred and twenty milligrams of the solid nanoparticle dispersion were placed in a mixture of 250 μl of the mentioned enzyme solutions and 250 μl of the given buffer used to dissolve insulin. At the mentioned time points, 500 μl of 0.5% TFA were added to stop the reaction. Samples were shaken for another 30 min for complete insulin dissolution under acidic conditions. For further analysis these samples were centrifuged at 37°C and the aqueous phase was separated from the lipid phase. The aqueous samples were stored at 4°C until HPLC analysis.
As a control, insulin dispersions in triglyceride with an insulin content similar to the nanoparticles suspensions were prepared; 120 mg of this control dispersion were treated in the same manner as the nanoparticles dispersions.
Statistical data analysis
Statistical data analyses were performed using the Student t-test with p < 0.05 as the minimal level of significance.
Results
Characterization of PAA100-Cys conjugate
The PAA-cysteine conjugate was synthesized according to a previously described method (CitationMarschütz & Bernkop-Schnürch, 2002). After activation of the carboxylic function of PAA with EDAC, L-cysteine was covalently attached to it via amid-formation. The obtained product was white, odourless, and showed a fibrous structure. The PAA100-Cys conjugate was investigated for its thiol and disulfide group content since the amount of thiol groups plays a crucial role for the physicochemical properties of the polymer. Moreover, the content of free cysteine in the conjugate was determined. In total, an amount of 397.22 ± 16.67 μmol of thiol and disulfide groups per gram polymer was determined, of which 312.45 ± 43.81 μmol/g were found to be free thiol groups. L-cysteine remainders of 13.25 ± 0.84 μmol/g were determined by using the TNBS-reagent.
Characterization of nanoparticles
Within this study, thiolated PAA nanoparticles were prepared via hydrogen bonding between PAA and PVP with subsequent oxidation by iodine in order to form disulfide bonds within the nanoparticles for stabilization. With respect to the stability of nanoparticles suspensions, the above mentioned percentages of PAA, insulin, and PVP delivered the best results concerning particle size and surface charge, since neither agglomeration nor precipitation could be observed within the obtained suspensions. Insulin was incorporated into nanoparticles during their formation. After centrifugation, no free insulin could be found in the supernatant. Therefore, it is assumed that the employed amount of insulin was completely incorporated into nanoparticles, which leads to a drug load of 7.0 ± 0.4% when correlated to the total mass of the obtained nanoparticles. The nanoparticles were slightly yellow and odourless. Particle size distributions after nanoparticle formation, after oxidation, and after release from the lipid matrix are depicted in . Furthermore, the zeta potential has been measured prior to oxidation. An overview of the physicochemical properties of the prepared particles is provided in .
Figure 1. Particle size distributions directly after precipitation (-□-), after oxidation (-▴-), and after release from the lipid formulation (-⧫-). Indicated values are means ± SD (n ≥ 3).
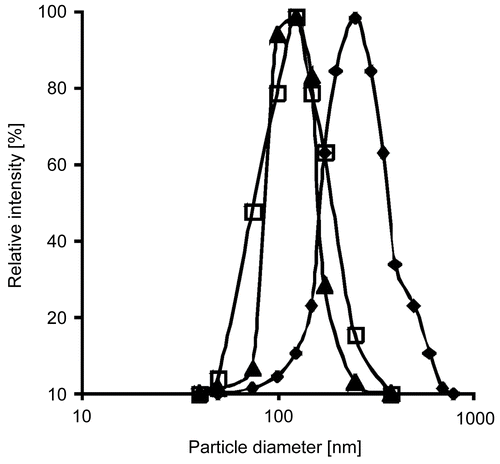
Table 1. Characterization of the utilized PAA100-Cys nanoparticles regarding mean particle diameter, zeta potential, and drug load. Indicated values are means ± SD (n ≥ 3).
In vitro release studies
Insulin release studies were performed with 120 mg of solid lipid dispersions of the nanoparticles in the above mentioned buffer solution. This mass of the dispersion equated to ~100 μg insulin. After further processing the aqueous phase was investigated for its insulin content, which was then related to the total amount of insulin. The total amount was calculated from exhaustive extraction of the solid dispersions in 1 M HCl. The insulin release profile of the dispersion is shown in .
Enzymatic degradation studies
Within these studies free insulin, insulin embedded in triglyceride, and solid dispersions of insulin-containing thiolated nanoparticles in triglyceride were incubated with solutions of digestive enzymes of the intestine. Insulin degradation by the mentioned enzymes was observed as a function of time. In the progress of insulin degradation by trypsin, α-chymotrypsin, and elastase is shown. It could be demonstrated that by using the solid nanoparticle dispersion 44.47 ± 0.89% of the initial insulin amount has been shielded from tryptic degradation within 3 h compared to insulin solutions, 21.33 ± 5.34% from α-chymotryptic degradation, and 45.07 ± 1.40% from degradation by elastase, respectively. After incubation of an insulin solution with α-chymotrypsin, no insulin could be detected after 10 min, whereas no more insulin was detectable after 120 min of incubation with elastase.
Figure 3. Degradation of insulin by trypsin in an aqueous solution (⧫), as a solid dispersion of insulin in triglyceride (▴), and as a solid dispersion of insulin-loaded PAA100-Cys nanoparticles in triglyceride (▪). Indicated values are means ± SD (n ≥ 3).
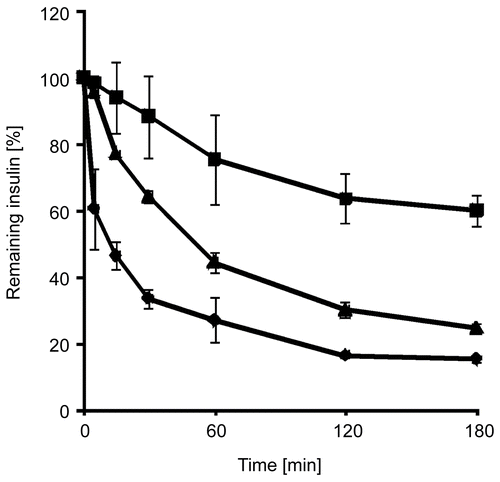
Discussion
Until the present day, oral delivery of protein and peptide drugs remains one of the major challenges in current formulation sciences. The enzymatic barrier of the GIT has been shown to be one of the major obstacles for a peptide on its way from the GIT to the systemic blood stream (CitationWoodley, 1994). For example, insulin has been shown to be intensively degraded by trypsin, chymotrypsin (CitationSchilling & Mitra, 1991), and elastase (CitationNarayanan & Anwar, 1969), besides other enzymes. Thus, the oral bioavailability of insulin is severely reduced before it can even reach the systemic blood stream. Consequently, it is one of the main aims in formulation sciences to overcome the enzymatic barrier.
In recent years different approaches have been developed in order to circumvent enzymatic degradation of peptides and proteins. Besides addition of inhibiting agents, incorporation of these drugs into micro- and nanoparticles as well as liposomes has become a major strategy to reach this aim. Due to the ability of polyacrylates to inhibit proteolytic enzymes (CitationBai et al., 1996) and further improvement of this ability by immobilization of thiol groups to the polymer backbone ( CitationBernkop-Schnürch, Walker, & Zarti, 2001), we consider it a reasonable approach to prepare thiolated PAA-nanoparticles in order to protect peptides and proteins from enzymatic degredation. In addition, thiolation of PAA allows oxidative cross-linking of particles, which is crucial to the stability of the prepared particles.
Investigations of the physical properties have shown mean diameters between 100–300 nm and zeta potentials of −14.3 ± 0.6 meV. According to (CitationLamprecht, Schafer, and Lehr, 2001), particles in the low nanometer-range show superior mucoadhesive properties. Furthermore, a much larger area of absorption can be reached by applying nano-sized drug carriers.
Within in vitro release studies it could be shown that a burst release of insulin can be circumvented by embedding of the nanoparticles in a solid lipid phase. For oral delivery of therapeutics it is crucial to exclude an immediate release, since different proteases would rapidly degrade peptides and proteins. Therefore, a release as close as possible to the area of absorption is favoured. Within the present study insulin was mainly released within the first hour. The observed release profile and the mucoadhesive properties of thiolated PAA could possibly allow an in vivo insulin release close to the intestinal mucosa, which will be additionally supported by the mentioned properties of the prepared nanoparticles. A drawback of this formulation is the incomplete release of insulin under the chosen conditions, which can be attributed to nanoparticulate remainders within the boundary layer between triglyceride and aqueous phase. Under in vivo conditions there would be a much larger dissemination area for the formulation, which will result in a larger boundary layer. In fact, this could lead to an improved in vivo release.
Concerning stability of peptides and proteins within nano-sized carriers, several indications for a significant protective potential of the carriers can be found in the literature. CitationSakuma et al. (1997) prepared calcitonin- containing polystyrene nanoparticles and investigated the stability of calcitonin towards trypsin and pepsin. It was found that polystyrene particles can protect calcitonin from degradation within 30 min of incubation. CitationAkiyoshi et al. (1998) investigated the stability of insulin within cholesterol-bearing pullulan nanoparticles towards α-chymotrypsin and could show that cleavage of insulin by α-chymotrypsin was significantly reduced within 30 min. In a more recent study, CitationJintapattanakit et al. (2007) could show that polyelectrolyte nanocomplexes of trimethyl chitosan are capable of protecting insulin. Within 60 min, ~40% of the initial insulin amount were degraded compared to ~70% in a solution of free insulin. CitationStark et al. (2008) examined liposomes concerning their protective capacities towards proteolytic enzymes. The degradation of vasoactive intestinal peptide was shown to be significantly reduced in the lung.
In order to investigate the full protective potential of the insulin-containing PAA100-Cys nanoparticle formulation it was exposed to three enzymes present in the gastrointestinal tract which are known to degrade insulin and drastically reduce its bioavailability. The utilized concentrations of trypsin, α-chymotrypsin, and elastase are found to be in the physiological range of 1 ml of pancreatic secretions. Being compared to other studies which investigated the stability of insulin incorporated into nanoparticles towards single enzymes, a protective effect towards three different enzymes which are present in the intestine could be demonstrated. The utilized formulation was capable of protecting more than 40% of the initial insulin amount from degradation by trypsin and elastase, whereas ~20% were protected towards degradation by α-chymotrypsin. Moreover, it could be demonstrated that undegraded insulin was still present within 3 h of incubation with all three enzymes, whereas insulin in solution was completely degraded by α-chymotrypsin and elastase after 10 min and 120 min, respectively. Within 3 h a systemic insulin uptake could possibly be realized, especially if the mucoadhesive and permeation enhancing properties of thiolated polymers are taken into consideration.
The achieved protective effect can be explained by the enzyme inhibitory capacities of poly(acrylic acid). This effect is attributed to surface inactivation of serinproteases when they get bound to acrylic polymers. Due to enzyme binding denaturation might occur, which consequently results in a loss of hydrolytic activity (CitationBernkop-Schnürch & Walker, 2001). Chelation of Ca2+- and Zn2+-ions can be excluded as a responsible mechanism for the inhibitory effect, since EDTA was present in all experiments. For the inhibitory effect of thiolated poly(acrylic acid) on brush border bound enzymes, chelation of metal ions will be of much higher relevance (CitationBernkop-Schnürch et al., 2001). Moreover, the nanoparticles themselves can function as a steric hindrance for enzymes on their way to the substrate.
Conclusion
In contemporary research a lot of expectations have been raised concerning nanoparticles as an oral delivery system for therapeutic proteins and peptides. Since enzymatic degradation pre-systemically decreases the oral bioavailability of insulin and other peptide drugs to a huge extent it is a logical approach to incorporate insulin into nanoparticles of a polymer with enzyme inhibiting capacities. In this study it could be shown that enzymatic degradation can be slowed down by the investigated formulation. Reduced enzymatic degradation combined with the further advantages of thiolated poly(acrylic acid) make this an interesting approach for oral peptide and protein delivery.
Acknowledgements
The Austrian Nano-Initiative co-financed this work as part of the Nano-Health project (no. 0200), the sub-project NANO-N-0204 being financed by the Austrian FWF (Fonds zur Förderung der Wissenschaftlichen Forschung) (Project no. N-0204-NAN).
Declaration of interest: The authors report no conflicts of interest. The authors alone are responsible for the content and writing of the paper.
References
- Akiyoshi, K., Kobayashi, S., Shichibe, S., Mix, D., Baudys, M., Kim, S.W., Sunamoto, J. (1998). Self-assembled hydrogel nanoparticles of cholesterol-bearing pullulan as a carrier of protein drugs: complexation and stabilization of insulin. J Contr Rel. 54, 313–20.
- Bai, J.P., Chang, L.L., Guo, J.H. (1996). Effects of polyacrylic polymers on the degradation of insulin and peptide drugs by chymotrypsin and trypsin. J Pharm Pharmacol. 48, 17–21.
- Bernkop-Schnürch, A., Fragner, R. (1996). Investigations into the diffusion behaviour of polypeptides in native intestinal mucus with regard to their peroral administration. Pharm Sci. 2, 361–3.
- Bernkop-Schnürch, A., Walker, G. (2001). Multifunctional matrices for oral peptide delivery. Crit Rev Ther Drug Carrier Syst. 18, 459–501.
- Bernkop-Schnürch, A., Schwarz, V., Steininger, S. (1999). Polymers with thiol groups: a new genereation of mucoadhesive polymers? Pharm Res. 16, 876–81.
- Bernkop-Schnürch, A., Walker, G., Zarti, H. (2001). Thiolation of polycarbophil enhances its inhibition of intestinal brush border membrane bound aminopeptidase N J Pharm Sci. 90, 1907–14.
- Bodmeier, R., Chen, H.G., Paeratakul, O. (1989). A novel approach to the oral delivery of micro- or nanoparticles. Pharm Res. 96, 413–7.
- Coupe, A.J., Davis, S.S., Wilding, I.R. (1991). Variation in gastrointestinal transit of pharmaceutical dosage forms in healthy subjects. Pharm Res. 8, 360–4.
- Cui, F.D., Tao, A.J., Cun, D.M., Zhang, L.Q., Shi, K. (2007). Preparation of insulin loaded PLGA-Hp55 nanoparticles for oral delivery. J Pharm Sci. 96, 421–7.
- Habeeb, A.F. (1973). A sensitive method for localization of disulfide containing peptides in column effluents. Anal Biochem. 56, 60–5.
- James, D.E., Jenkins, A.B., Kaegen, E.W., Chisholm, D.J. (1981). Insulin precipitation in artificial infusion devices. Diabetologia. 21, 554–7.
- Jintapattanakit, A., Junyaprasert, V.B., Mao, S., Sitterberg, J., Bakowsky, U., Kissel, T. (2007). Peroral delivery of insulin using chitosan derivatives: a comparative study of polyelectrolyte nanocomplexes and nanoparticles. Int J Pharm. 342, 240–249.
- Kriwet, B., Walter, E., Kissel, T. (1998). Synthesis of bioadhesive poly(acrylic acid) nano- and microparticles using an inverse emulsion polymerization method for the entrapment of hydrophilic drug candidates. J Contr Rel. 56, 149–58.
- Lamprecht, A., Schafer, U., Lehr, C.M. (2001). Size-dependent bioadhesion of micro- and nanoparticulate carriers to the inflamed colonic mucosa. Pharm Res. 18, 788–93.
- Marschütz, M.K., Bernkop-Schnürch, A. (2000). Oral peptide drug delivery: polymer-inhibitor conjugates protecting insulin from enzymatic degradation in vitro. Biomaterials. 21, 1499–507.
- Marschütz, M.K., Bernkop-Schnürch, A. (2002). Thiolated polymers: self-crosslinking properties of thiolated 450 kDa poly(acrylic acid) and their influence on mucoadhesion. Eur J Pharm Sci. 15, 387–94.
- Mollmann, S.H., Elofsson, U., Bukrinsky, J.T., Frokjaer, S. (2005). Displacement of adsorbed insulin by Tween 80 monitored using internal reflection fluorescence and ellipsometry. Pharm Res. 22, 1931–41.
- Narayanan, A.S., Anwar, R.A. (1969). The specifity of purified porcine pancreatic elastase. Biochem J. 114, 11–17.
- Nurkeeva, Z.S., Mun, G.A., Khutoryanskiy, V.V., Bitekenova, A.B., Dubolazov, A.V., Esirkegenova, S.Z. (2003). pH effects in the formation of interpolymer complexes between poly(N- vinylpyrrolidone) and poly(acrylic acid) in aqueous solutions. Eur Phys J E Soft Mater. 10, 65–8.
- Palmberger, T.F., Albrecht, K., Loretz, B., Bernkop-Schnürch, A. (2007). Thiolated polymers: evaluation of the influence of the amount of covalently attached L-cysteine to poly(acrylic acid). Eur J Pharm Biopharm. 66, 405–412.
- Ponchel, G., Irache, J. (1998). Specific and non-specific bioadhesive particulate systems for oral delivery to the gastrointestinal tract. Adv Drug Deliv Rev. 34, 191–219.
- Pusztai, A. (1989). Transport of proteins through the membranes of the adult gastro-intestinal tract—a potential for drug delivery? Adv Drug Deliv Rev. 3, 215–28.
- Sakuma, S., Ishida, Y., Sudo, R., Suzuki, N., Kikuchi, H., Hiwatari, K., Kishida, A., Akashi, M., Hayashi, M. (1997). Stabilization of salmon calcitonin by polystyrene nanoparticles having surface hydrophobic polymeric chains, against enzymatic degradation. Int J Pharm. 159, 181–9.
- Satake, K., Okuyama, T., Ohashi, M., Shinoda, T. (1960). The spectrophotometric determination of amines, amino acids and peptide with 2,4,6-trinitrobenzene-1-sulfonic acid. J Biochem. 47, 654.
- Schilling, R.J., Mitra, A.K. (1991). Degradation of insulin by trypsin and alpha-chymotrypsin. Pharm Res. 8, 360–4.
- Stark, B., Andreae, F., Mosgoeller, W., Edetsberger, M., Gaubitzer, E., Koehler, G., Prassl, R. (2008). Liposomal vasoactive intestinal peptide for lung application: protection from proteolytic degradation. Eur J Pharm Biopharm. 70, 153–64.
- Werle, M., Samhaber, A., Bernkop-Schnürch, A. (2006). Degradation of teriparatide by gastro-intestinal proteolytic enzymes. J Drug Target. 14, 109–15.
- Woodley, J.F. (1994). Enzymatic barriers for GI peptide and protein delivery. Crit Rev Ther Drug Carrier Syst. 11, 61–95.