Abstract
Acute liver injury is a common clinical disease, which easily leads to liver failure and endangers life, seriously threatening human health. Naringenin is a natural flavonoid that holds therapeutic potential against various liver injuries; however it has poor water solubility and bioavailability. In this study, we aimed to develop naringenin-loaded bovine serum albumin nanoparticles (NGNPs) and to evaluate their hepatoprotective effect and underlying mechanisms against acetaminophen overdose toxicity. In vitro data indicated that NGNPs significantly increased the drug solubility and also more effectively protected the hepatocyte cells from oxidative damage during hydrogen peroxide exposure or lipopolysaccharide (LPS) stimulation. In vivo results confirmed that NGNPs showed an enhanced accumulation in the liver tissue. In the murine model of acetaminophen-induced hepatotoxicity, NGNPs could effectively alleviate the progression of acute liver injury by reducing drug overdose-induced levels of oxidative stress, inflammation and apoptosis in hepatocytes. In conclusion, NGNPs has strong hepatoprotective effects against acetaminophen induced acute liver injury.
1. Introduction
Acute liver injury (ALI) is a life-threatening clinical syndrome characterized by rapid loss and abnormality of liver cell function in patients with or without the prior liver disease (Kim et al., Citation2019). ALI can be caused by a viral infection, alcohol abuse, drug overdose, or toxin exposure and other exogenous substances, which can easily develop to liver failure and endanger life, seriously threatening human health (Peng et al., Citation2019). So far, ALI has no effective clinical treatment strategy and drug therapy. Therefore, it is urgent to develop a new drug to treat ALI. The pathogenesis of ALI is mainly caused by an inflammatory response and redox imbalance, resulting in liver cells death and liver dysfunction (Zhong et al., Citation2016). During the progression of ALI, many reactive oxygen species (ROS) are generated in liver cells, which can enhance the expression of proinflammatory genes by stimulating intracellular signal cascades. Also, inflammatory cells can produce more ROS, leading to excessive oxidative stress at the inflammatory site. Thus, oxidative stress and inflammation together formed a vicious cycle, which promote the development of liver injury (Li et al., Citation2016). Therefore, simultaneous inhibition of oxidative stress and inflammatory response could be a desired drug therapy against acute liver injury.
Naringenin, one main component of citrus fruits, is a natural flavonoid with many potential therapeutic applications. The effective pharmacological activities of this bioactive compound include anti-tumor, neuroprotective, anti-oxidative, anti-adipogenesis, anti-inflammatory and anti-injurious effects. Naringenin has been explored in the treatment of many inflammatory diseases due to its impressive anti-oxidative and anti-inflammatory effects (Gratieri et al., Citation2020). The antioxidant effect of naringenin is mainly through reducing ROS and enhancing antioxidant activity, which has been tested in the treatment of cardiovascular, neurodegenerative, diabetes, kidney disease and other oxidative stress-induced diseases (Zaidun et al., Citation2018). In addition, literature has proved that naringenin has a protective effect on inflammatory diseases such as encephalomyelitis, arthritis, and airway inflammation (Niu et al., Citation2021). Although naringenin has the potential to treat many diseases as a therapeutic agent, its application in the treatment of acute liver injury has several obvious limitations, including poor water solubility, low bioavailability, and insufficient retention in the liver (Brodersen, Citation1974; Gratieri et al., Citation2020). Therefore, a method to increase the solubility, bioavailability and liver targeting of naringenin is urgently needed.
The rapid development of nanotechnology provides an effective method to increase the water-solubility, improve bioavailability and achieve liver targeting of insoluble drugs. What should be mentioned is that the mononuclear phagocyte system (MPS, such as liver and spleen) will engulf the nanoparticles in the blood, allowing nanoparticles to easily accumulate in the liver through passive targeting effect (Ni et al., Citation2019). Therefore, nanotechnology is an effective strategy to solve the limitations of naringenin. Recently, scientists have developed various naringenin nanomedicine to increase water solubility and optimize the pharmacokinetic profiles (Yen et al., Citation2009; Khan et al., Citation2015; Zobeiri et al., Citation2018; Budel et al., Citation2020). Many studies have found that naringenin nanoparticles could reduce the levels of inflammatory mediators to a greater extent than free naringenin as well as protect cells from oxidative stress (Kumar & Abraham, Citation2017; Ahmad et al., Citation2019; Wang et al., Citation2020; Yang et al., Citation2020; Goncalves et al., Citation2021). For example, Kumar et al. synthesized naringenin nanoparticles coated with chitosan by the ionic gelation method, and nanoparticle’s in vitro antioxidant activity was superior to free naringenin (Kumar et al., Citation2015). In addition, Shadab MD et al. recently developed a naringenin-soy protein complex, which was then incorporated into an aqueous-based gel to deliver naringenin specifically to treat colon cancer (Md et al., Citation2022). The final preparation significantly increased the solubility and strengthened anticancer effect of naringenin. This study indicated that naringenin-protein complex could be a suitable option for naringenin formulation design. It’s worth noting that bovine serum albumin (BSA) has been widely used as a nanocarrier due to its good biocompatibility, biodegradability, easy surface functionalization and high water solubility (Wang & Zhang, Citation2018; Tan et al., Citation2021). Therefore, we developed BSA-based and naringenin-encapsulated nanoparticles (NGNPs) using a desolvation method. The prepared nanoparticles could evenly disperse naringenin in an aqueous solution, thus solving the limitations of naringenin.
In this study, naringenin albumin nanoparticles (NGNPs) were prepared to address the poor water solubility of naringenin and achieve liver targeting, and their particle size, zeta potential and biocompatibility were characterized. Also, in vitro studies were conducted to study the antioxidant and anti-inflammatory activities of NGNPs. In addition, the liver targeting efficiency and therapeutic effect were further evaluated in a mouse model of acute acetaminophen (APAP) overdose induced liver injury.
2. Materials and methods
2.1. Materials, cell lines, and experimental animals
Naringenin was bought from Chengdu Pufei De Biotech Co., Ltd. (Chengdu, China). Acetaminophen (APAP) was obtained from Aladdin Reagent Co., Ltd. (Shanghai, China). Bovine serum albumin, superoxide dismutase (SOD) activity kit and reactive oxygen species (ROS) assay kit were from Beyotime Biotechnology (Nanjing, China). 1,1-dioctadecyl-3,3,3,3-tetramethylindotricarbocyaine iodide (DiR) was provided by Yeasen Biotechnology (Shanghai) Co., Ltd. (Shanghai China). Hydrogen peroxide (H2O2), penicillin-streptomycin-neomycin solution, and 3-(4,5-dimethylthiazol-2-yl) −2,5-Diphenyltetrazolium bromide (MTT) were purchased from Sigma-Aldrich (MO, USA). Fetal bovine serum (FBS), Dulbecco’s Modified Eagle’s Medium (DMEM) and DMEM/Ham’s F-12 Medium were provided by Gibco (Carlsbad, USA). Malondialdehyde (MDA) assay kit was provided by Solarbio (Beijing, China). Lactate dehydrogenase (LDH) assay kit and alkaline phosphatase (AKP) assay kit were provided by Nanjing Jiancheng Bioengineering Institute. Anti-Bax (50599-2-lg) was provided by Proteintech (Chicago, USA). Anti-tumor necrosis factor-α (TNF-α) (AF7014) and Anti-interleukin-6 (IL-6) (DF6087) were purchased from Affinity Biosciences (Cincinnati, USA). Anti-Bcl-2 (ab7973) and Anti-IL-1β (ab9722) were purchased from Abcam (MA, USA). TNF-α and IL-6 enzyme-linked immunosorbent assay (ELISA) kits were purchased from Shanghai Jianglai Biological Technology Co., Ltd. (Shanghai, China).
AML12 cells, RAW264.7 cells, HepG2 cells, HUVEC cells and 3T3 cells were purchased from the cell bank of type culture collection of Chinese Academy of Sciences (Shanghai, China). AML12 cells were grown in DMEM/F-12 medium containing 10% FBS and 1% penicillin/streptomycin. Other cells were cultured in DMEM medium containing 10% FBS and 1% penicillin/streptomycin. Cells were cultured at 37 °C in a humidified incubator with 5% CO2.
Male ICR mice aged 8 weeks (25-35 g) were obtained from Shanghai Laboratory Animal Center. All animal experiments were approved by Institutional Animal Care and Use Committee of Wenzhou Medical University (Ethical approval number: xmsq2022-0295).
2.2. Preparation and characterization of naringenin nanoparticles (NGNPs)
BSA was used as a nanoparticulate delivery carrier for naringenin. NGNPs were prepared with a modified desolvation technique (Huang et al., Citation2018). Briefly, 100 mg BSA was firstly dissolved into 4 mL pH 7.4 buffer. Then 6 mL ethanol containing 6 mg naringenin was added dropwise to BSA solution at the rate of 1 mL/min under magnetic stirring of 500 rpm. After 6 h continuous stirring at room temperature, the formed nanoparticle solution was crosslinked with 25 µL 8% glutaraldehyde solution. After 1 h additional stirring, the resulting nanoparticle solution was purified by repeated centrifugation at 12,000 rpm for 30 minutes. Finally, the NGNPs nanoparticles were collected by re-dispersion in double-distilled water and freeze-drying. To visualize NGNPs after intravenous injection, DiR was used to track NGNPs, and DiR-labelled NGNPs were prepared by adding DiR into naringenin ethanol solution during the nanoparticle preparation.
The freeze-dried nanoparticles were dispersed in double-distilled water and placed in a specific chamber. The particle size and zeta potential of the nanoparticles were measured using a particle analyzer (Litesizer™ 500, Anton-Paar, Ltd., Graz, Austria). The nanoparticle morphology was observed by a transmission electron microscope (TEM, JEM 1200EX, JEOL Ltd., Japan). Briefly, nanoparticle solution was dropped onto a carbon coated copper grid, and the excess water was carefully removed by the filter paper. Then, 0.2% phosphotungstic acid was droped onto the sample side for counterstain. After removing excess solution, the sample was dried at room temperature and then tested under TEM. The freeze-dried nanoparticles were analyzed using KBr disk method by Fourier transform infrared (FTIR) spectroscopy (Nicolet IS10, Thermo Nicolet Corporation, USA). For each measurement, 64 scans were co-added with a resolution of 4 cm−1 and the wave number ranging from 400 to 4000 cm−1. In addition, the lyophilized samples were also studied by X-ray diffraction (XRD, BRUCKER D8 ADVANCE, BRUCKNER, German). For each measurement, the scanning angle range was 5 to 90 degrees, and the scanning rate was 6 degree/min.
We also evaluated the solubility enhancement of NGNPs by comparing the relative solubility of free naringenin and NGNPs in physiological pH. To be specific, 1 mg naringenin or equivalent NGNPs were added into 1 mL phosphate buffer saline (PBS) solution (pH 7.4). After stirring at 37 °C for 12 h, the samples in each group were centrifuged at 3000 rpm for 2 min and photographed. The supernatant was collected, and an equal volume of 10% NaOH solution was added to make a mixture. After 40 min, the absorbance at 309 nm was determined by UV spectrophotometry, and the naringenin concentration was calculated using the standard curve method.
2.3. In vitro release and stability study
The cumulative drug release profile was studied using a classic dialysis method (Chen et al., Citation2022; Kou et al., Citation2022). Firstly, pH 7.4 PBS solution using the commercial product (P1010-2L, Solarbio Beijing, China) by directly dissolving the one bag of powder into 2 L of double distilled water; then, 5% of Tween-80 was added into the prepared PBS to ensure the sink condition. Naringenin solution (1 mg/mL) and equivalent NGNPs were added into a dialysis bag (MWCO, 3500 Da), and immersed into 20 mL the prepared release medium (pH 7.4 PBS containing 5% Tween 80), which placed in a water bath shaking at 100 rpm (37 °C). At predetermined time points, 1 mL medium was collected to measure the released naringenin concentration. 1 mL of fresh medium was added to maintain the release medium at the same volume.
The colloidal stability of NGNPs was measured by monitoring the particle size change after incubating in a medium containing 0%, 5%, 10%, and 50% fetal bovine serum (FBS) at 37 °C.
2.4. In vitro hemocompatibility test and biocompatibility studies
A hemocompatibility test was used to study the potential adverse effects of NGNPs on blood or blood components. The purified water, 0.9% NaCl or NGNPs (10 μg/mL, 20 μg/mL, 50 μg/mL, 100 μg/mL) were incubated with a 2% suspension of red blood cells at 37 °C. After 2 h incubation, the samples were centrifuged at 1000 rpm for 5 min. The supernatant was collected, and the absorbance at 525 nm was determined by ultraviolet spectrophotometry. The relative hemolysis rate was calculated as the following equation (Yao et al., Citation2020): Hemolysis rate (%) = [(Asample - Anegative control)/(Apositive control - Anegative control)] × 100%. The A indicates the absorbance of specific sample at 525 nm.
In vitro biocompatibility of NGNPs was evaluated using MTT assay. AML12 cells, HepG2 cells, 3T3 cells or HUVEC cells were seeded in 96-well plates with a density of 5000 cells/well. After 12 h growth, the cells were treated with different formulations for 24 h. After treatment, the cells were incubated with a fresh medium containing 0.5 mg/mL of MTT for 3 h. Finally, the medium was removed and replaced with DMSO to dissolve the formazan crystals. The absorbance was measured at a wavelength of 490 nm and 570 nm using a microplate spectrophotometer (SPECTRA MAX 190, Molecular Devices, CA, USA).
2.5. In vitro antioxidant and anti-inflammatory properties
To evaluate the antioxidative effect of NGNPs, AML12 cells were seeded in 6-well plates with a density of 1 × 106 cells/well. After 12 h growth, the cells were firstly incubated with naringenin or NGNPs for 24 h and then treated with H2O2 for 2 h. The LDH and MDA levels, and SOD activities were determined with the appropriate detection kits. The intracellular ROS levels were measured using a ROS assay kit and observed with an inverted fluorescence microscope (TS2, NIKON, Tokyo, Japan). The fluorescent images were recorded and semi-quantified using the Image J software. In addition, AML12 cells were also seeded in 96-well plates with a density of 5000 cells/well and treated in the same way as above. The cell viability was determined by MTT assay.
Also, the expression level of proinflammatory cytokines were measured as previously reported (Yao et al., Citation2020; Kou et al., Citation2022). Briefly, RAW 264.7 cells were first stimulated with 3 μg/mL lipopolysaccharide (LPS) for 6 h, and then incubated with naringenin or NGNPs for another 24 h. Following that, the cell supernatants were harvested to measure TNF-α and IL-6 levels by ELISA kits. Also, the relative expression levels of IL-6 and TNF-α in treated macrophages were also observed by immunofluorescence staining.
2.6. APAP-induced ALI model and in vivo drug treatment
The Male ICR mice (25-35 g) were fasted for 12 h, then received gavage administration of APAP (800 mg/kg) to develop an APAP-induced ALI mice model. Then, the ALI mice were randomly assigned into three groups: i. ALI + saline; ii. ALI + naringenin (25 mg/kg, i.v.); iii. ALI + NGNPs (25 mg/kg of naringenin, i.v.). The drug therapy was given at 2 h after the ALI model was established. Untreated healthy mice were used as control. After 24 h, high-resolution ultrasound imaging (RESOA 8 PRO, Mindray, Shenzhen, China) was performed to evaluate the liver conditions. Then, the serum samples were collected from mice to determine the levels of alkaline phosphatase (AKP), alanine aminotransferase (ALT), aspartate aminotransferase (AST) using AU480 Chemistry Analyzer (Beckman Coulter, CA, USA). After that, the mice were all sacrificed with the liver tissue collected for further evaluation. The liver index was calculated as the ratio of liver weight/body weight. Then we also determined the weight of liver tissue samples before and after drying (60 °C, 12 h), called Liver Wet-Dry ratio, representing the hepatic edema. Additionally, the liver tissue was fixed, paraffin embedded, and sectioned for further histological analysis.
2.7. In vivo imaging and biodistribution assay
DiR was used as a probe to monitor the biodistribution of intravenous injected NGNPs in the established ALI mice model. ALI mice were injected with DiR solution or DiR-labeled NGNPs (DiR, 0.05 mg/kg). The fluorescence images were recorded at the predetermined time points use a whole-body imaging system (Exc = 735 nm, Emi = 800 nm). After 24 h, the mice were sacrificed with their main organs collected to visualize the tissue distribution under the imaging system.
2.8. Histological analysis
Tissue samples were fixed, paraffin embedded, and sliced. Then the sections were deparaffinized, rehydrated, and stained with hematoxylin and eosin (H&E) to observe hepatic pathology. The H&E staining images were blindly analyzed to grade liver injury as previously reported (Tong et al., Citation2019). Briefly, liver injuries were assessed by following parameters, including cytoplasmic discoloration, vacuolation formation, nuclear pyknosis, nuclear fragmentation, nuclear discoloration, and red cell stasis. The liver injury severity is measured on a scale of 0-4 with higher score indicating more severe injury.
In addition, paraffinized liver sections were also studied with immunohistochemistry (IHC) staining. After blocking with 10% goat serum, the sections were incubated with the primary antibodies against TNF-α (AF7014, 1:200, Affinity), IL-6 (DF6087, 1:200, Affinity), IL-1β (ab9722, 1:500, Abcam), Bcl-2 (ab7973, 1:100, Abcam), or Bax (50599-2-lg, 1:500, Proteintech) overnight at 4 °C. Following that, the secondary antibody incubation and DAB color rendering were performed, and the images were visualized under a microscope. In addition, quantitative analysis was conducted using Image J software.
2.9. In vivo safety assessment
In vivo toxicity experiment was conducted to further evaluate the safeness of NGNPs (Ma et al., Citation2019). ICR mice (25-35 g) were assigned into 2 groups: Saline and NGNPs (100 mg/kg, i.v.). Any signs of toxicity in mice were monitored for 14 days after administration. After 14 days, the mice were sacrificed with blood and major tissues collected for further examination.
2.10. Statistical analysis
The data was subjected to statistical analysis by using GraphPad Prism 7.0 (GraphPad Software, San Diego, CA). All the results were presented as mean ± SD. Significance testing in comparisons is based on analysis of variance (ANOVA) and student t-test. A p value < 0.05 was considered as statistically significant.
3. Results and discussion
3.1. Preparation and characterization of NGNPs
The naringenin nanoparticles were prepared by a modified desolvation method as reported (Huang et al., Citation2018). As shown in and , we characterized the obtained nanoparticle samples, including BSANPs (blank BSA nanoparticles) and NGNPs with particle size, zeta potential, TEM, FTIR, XRD, stability, release behavior and relative solubility. shows that the hydrodynamic diameter distribution is narrow (PDI < 0.2), and the average hydrodynamic diameters of BSANPs and NGNPs were 170.53 ± 1.06 nm and 238.19 ± 3.24 nm, respectively. The increased particle size of NGNPs might be due to naringenin loading. But BSANPs and NGNPs showed no difference in zeta potential, which is ranging from −25 mV to −26 mV (). TEM data () presented the morphological characteristics of BSANPs and NGNPs, and both were spherical discrete and well-distributed. These data confirmed the successful preparation of NGNPs with appropriate particle size and uniform dispersion.
Figure 1. Characterization of naringenin nanoparticles (NGNPs). Hydrodynamic size distributions of (A) BSANPs and (B) NGNPs. (C) Zeta potential of BSANPs and NGNPs. (D) TEM images of BSANPs and NGNPs. (E) FTIR spectra and (F) XRD spectra of naringenin, BSANPs and NGNPs.
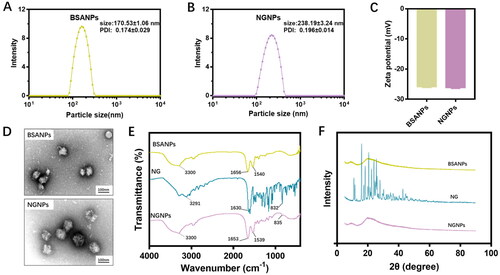
Figure 2. The stability, in vitro release profiles, and solubilizing effect of NGNPs. (A) The change of particle size of NGNPs in PBS buffer containing 5%, 10% and 50% FBS. (B) The change of particle size of NGNPs during 36 h storage. (C) The cumulative release of naringenin from naringenin solution and NGNPs. (D) The photos of naringenin or equivalent NGNPs in pH 7.4 PBS after centrifugation. (E) Relative solubility of naringenin in the collected supernatant.
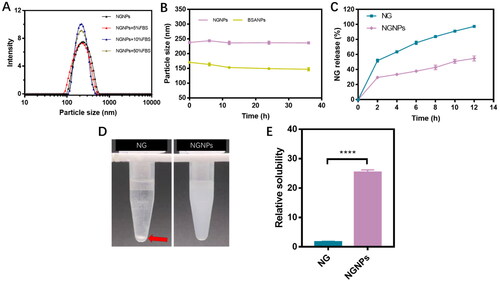
The physical interaction of naringenin and BSANPs within nanoparticles was also studied with FTIR measurements (). As indicated, FTIR spectrum of BSA had characteristic absorbance peaks, like 3300 cm−1 (N-H stretching vibration), and 1656 cm−1 (peptide carbonyl stretching vibration). Additionally, FITR spectrum of naringenin had three distinct peaks at 3291 cm−1, 1630 cm−1 and 832 cm−1, which belonged to the O-H stretching vibration, C = O stretching vibration and C-H bonding vibration. The spectrum of NGNPs exhibited most characteristic peaks in naringenin and BSA, confirming the successful naringenin loading in the BSA nanoparticulate carrier. Notably, the peaks at 1630 cm−1 and 832 cm−1, which were characteristic peaks of naringenin, had a distinct blue shift in the spectrum of NGNPs. It might be explained by that the binding of naringenin to a hydrophobic pocket in BSA (Xiao et al., Citation2007). Moreover, XRD spectra of naringenin, BSANPs, and NGNPs also provide evidence of the successful preparation. The XRD spectrum of BSANPs and NGNPs showed characteristic peaks at 20.1°, and multiple peaks of naringenin could be observed in the 10-30° range (). The characteristic peak of naringenin disappeared in NGNPs spectrum, indicating that the crystal structure of naringenin was destroyed during the preparation of nanoparticles.
Nanoparticle stability is an important factor for nanomedicine development and application. As we know, the nanoparticulate structure must be maintained stable in vivo long enough before the drug can be delivered to the targeted tissue. But the interaction of nanoparticles with blood components might transfer or disrupt the nanoparticles. Thus, we first monitored the nanoparticle stability in the presence of fetal bovine serum (FBS). The NGNPs were incubated in PBS containing 5%, 10%, and 50% FBS for 24 h with the particle size measured. As show in , NGNPs exhibited excellent stability by remaining uniform particle size. Then we also monitored the particle size during storage (25 °C, 36 h) to evaluate the colloidal stability (). Negligible change in the particle size was observed in both BSANPs and NGNPs, representing good storage stability. Additionally, naringenin release curves of NGNPs were also studied. As shown in , NGNPs showed slow and sustained release compared to rapid release of naringenin solution over a short period, suggesting that NGNPs can control naringenin release and ensure continued effect during treatment.
The water solubility of free naringenin and NGNPs was studied (). As shown in , large amount of white sediment was presented in the bottom of the test tube, indicating the low aqueous solubility of naringenin (Chen et al., Citation2021). In contrast, almost no sediments were observed in NGNP groups. The concentration of naringenin detected in the supernatant confirmed that the solubility of the nanoparticles group was 15 times higher than that of naringenin group in PBS at pH 7.4 (). Thus, the nanoparticle encapsulation significantly enhanced the naringenin solubility in PBS, and make it suitable for intravenous use.
3.2. In vitro safety and biocompatibility of NGNPs
To investigate the safety of intravenous administration of NGNPs, a hemolytic experiment was performed. As shown in , purified water caused erythrocyte rupture and hemoglobin leaked from the cell, resulting in a transparent red colored solution. But 0.9% NaCl solution stayed colorless, indicating erythrocyte maintained intact and no hemolysis occurred. The NGNPs incubated samples mostly remained colorless or light pink. Also, we measured the absorbance of supernatants collected from each group and calculated the hemolytic rate. The hemolytic rates of 10 μg/mL, 20 μg/mL, 50 μg/mL, 100 μg/mL NGNPs were all below the threshold (5%) (), indicating the biosafety of NGNPs when they were intravenously injected.
Figure 3. In vitro hemolysis test and biocompatibility of NGNPs. (A) Hemolysis test of NGNPs (a: purified water; b: 0.9% NaCl solution; c: 10 μg/mL NGNPs; d: 20 μg/mL NGNPs; e: 50 μg/mL NGNPs; f: 100 μg/mL NGNPs). (B) Hemolysis ratio of NGNPs. The in vitro biocompatibility of NGNPs were tested in (C) AML12 cells, (D) HepG2 cells, (E) 3T3 cells and (F) HUVEC cells.
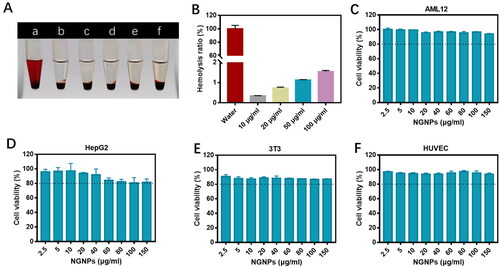
The in vitro biocompatibility of NGNPs was determined in AML12 cells, HepG2 cells, 3T3 cells, and HUVEC cells using MTT assay. All tested cells maintained a high cell viability after incubated with different concentrations of NGNPs (2.5-150 μg/mL). Even when the concentration of NGNPs reached up to 150 μg/mL, the cell viability was over 90% (). These results indicated that NGNPs had high biosafety and good cellular biocompatibility, allowing for further in vivo application.
3.3. In vitro antioxidative and anti-inflammatory effects of NGNPs
Oxidative stress is one of the key factors promoting the progression of liver injury. Excessive ROS can degrade proteins, lipids and DNA, leading to apoptosis of hepatic cells (Li et al., Citation2016). Previous studies shown that naringenin has a strong antioxidant effect and can effectively remove ROS, providing cell protection against oxidative stress (Hua et al., Citation2021). In order to investigate if NGNPs could protect hepatic cells by relieving oxidative stress, AML12 cells were first incubated with naringenin or NGNPs for 24 h and then injured with H2O2. DCFH-DA was used as a fluorescent probe to detect the ROS levels in each group (). H2O2-stimulated AML12 cells produced large amounts of ROS compared to normal cells. Pre-incubation of free naringenin or NGNPs reduced ROS production in H2O2-stimulated AML12 cells. Semi-quantitative results () showed that NGNPs had a stronger ability to inhibit ROS production compared with free naringenin. The protective effect of NGNPs on H2O2-damaged cell viability were also evaluated. AML12 cells lost 54% after H2O2-treatment (). The activity of cells pre-incubated with free naringenin or NGNPs was retained. Meanwhile, we measured the LDH release in each group and found that the H2O2 stimulated group released a large amount of LDH, representing a large degree of cell damage (). NGNPs significantly reduced LDH release, even back to normal level, further demonstrating its strong protection effect.
Figure 4. In vitro antioxidant effects of NGNPs. (A) Intracellular ROS levels of H2O2-Stimulated AML12 cells after treatment with NG and NGNPs was observed by fluorescent microscopy. (B) Semi-quantitative results of fluorescence intensity of ROS. (C) Cell viability of H2O2-stimulated AML12 cells after treatment with NG and NGNPs. The level of (D) LDH, (E) MDA and (F) SOD in H2O2-stimulated AML12 cells after treatment with NG and NGNPs. Scale bar = 100 μm. Data are presented as means ± SEM. n.s., no statistical significance. *P < 0.05, **P < 0.01, ***P < 0.001, ****P < 0.0001.
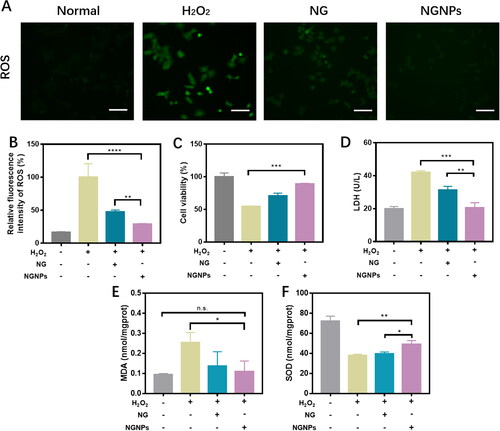
Studies have shown that antioxidant enzymes can maintain the cell redox homeostasis, while excessive ROS will consume these enzymes, leading to oxidative damage of cells (Huang et al., Citation2021). Therefore, we also detected MDA and SOD levels in each group of cells. As shown in , MDA levels in cells significantly increased after H2O2 injury. Compared with the H2O2 stimulation group, MDA level in both naringenin and NGNPs groups were decreased, and NGNPs group showed the greatest reduction. Meanwhile, SOD activity of cells was greatly inhibited after H2O2 injury (). Both NGNPs and naringenin can enhance SOD activity and antioxidative capacity. Similarly, NGNPs had the most significant effect on increasing the SOD activity of H2O2-stimulated cells. NGNPs increased the stability of naringenin and continued to release the drug to protect cells from oxidative stress damage. It is worth mentioning that compared with free naringenin, NGNPs had a stronger protective effect on liver cells, with higher SOD activity and lower LDH release and MDA content.
The brilliant protectional property of NGNPs must be attributed to the loaded naringenin. As a flavonoid mainly from citrus fruits, naringenin’s structure is responsible for antioxidant activity. It has been reported that naringenin could directly remove reactive oxygen species to protect cells from oxidative damage (Yang et al., Citation2011; Ge et al., Citation2021; Veiko et al., Citation2021). In addition, naringenin could also active nuclear factor E2-related factor 2 (Nrf2) and elevate its downstream target genes (including heme oxygenase 1 (HO-1) and NAD(P)H dehydrogenase 1 (NQO1)) to reduce oxidative stress (Wang et al., Citation2017; Pan et al., Citation2022). It was suggested that naringenin could suppress oxidative stress not only by directly scavenging ROS but also by activating Nrf2 and upregulating the downstream antioxidative enzymes. Notably, free naringenin and NGNPs can reduce oxidative stress injury of cells, while NGNPs have better antioxidant activity. This may be because naringenin is susceptible to degradation due to oxidation and light, while NGNPs can stabilize the drug for sustained release, therefore exerting better antioxidant activity compared to free naringenin (Cordenonsi et al., Citation2016).
Inflammation response often occurs during acute liver injury, which can provoke further liver injury and inhibit regeneration through the damaging effects of proinflammatory cytokines. Naringenin possesses potential in the treatment of a variety of inflammation-related diseases and can inhibit the production of proinflammatory factors and weaken inflammatory responses (Zeng et al., Citation2018). Mononuclear macrophages play an important part in the host defense system, and the proinflammatory response of macrophages is involved in the pathophysiological process of acute liver injury. Excessive release of inflammatory cytokines by macrophages has been widely reported as an important contributor to the progression of liver injury (Peng et al., Citation2019). Therefore, we used RAW264.7 macrophages to test the anti-inflammatory effects of naringenin and NGNPs. Immunofluorescence staining was used to detect the expression of representative inflammatory cytokines, including TNF-α and IL-6 (). The fluorescence intensity in the LPS group was defined as 100%. Fluorescence semi-quantitative results showed that the expression of inflammatory factors was significantly reduced in NGNPs-pretreated RAW264.7 cells (). We then used ELISA kits to detect TNF-α and IL-6 levels in cell culture medium. In the same dose of naringenin or NGNPs pretreatment, both TNF-α and IL-6 levels in LPS-stimulated macrophages were reduced (). NGNPs were more effective at reducing inflammatory cytokine secretion than free naringenin. This is attributed to the high uptake rate of BSA based nanoparticles in RAW264.7 cells, in addition to NGNPs increasing the stability of naringenin (Zhang et al., Citation2016; da Silva et al., Citation2018). Therefore, NGNPs can reduce oxidative stress and protect liver cells by inhibiting the production of ROS and proinflammatory cytokines.
Figure 5. In vitro anti-inflammatory effects of NGNPs. RAW264.7 cells were stimulated with LPS (3 μg/mL) for 6 h and the fluorescence images of (A) TNF-α and (B) IL-6 in each group were obtained. Scale bar = 50 μm. Fluorescence semi-quantitative statistics of (C) TNF-α and (D) IL-6 in RAW264.7 cells of each group. The secreted amount of (E) TNF-α and (F) IL-6. **P < 0.01, ***P < 0.001, ****P < 0.0001.
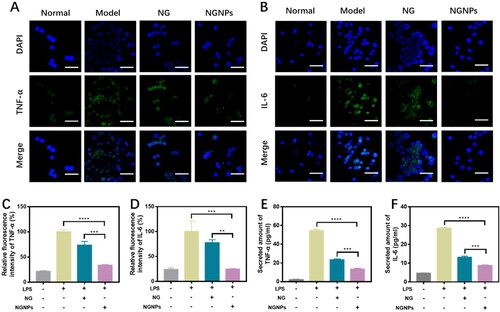
3.4. In vivo biodistribution of NGNPs
We then studied the in vivo biodistribution of NGNPs in an acute liver injury model. Free DiR or DiR-NGNPs (0.05 mg/kg of DiR) were injected intravenously, and fluorescence images were collected at different time points (0.5 h, 2 h, 4 h, 6 h, 12 h, and 24 h) after administration. As shown in , DiR-NGNPs are enriched in the liver and stay there for a prolonged time, while free DiR was eliminated soon after reaching the liver, indicating that DiR-NGNPs is liver-targeting and not easily eliminated in vivo. Fluorescence images of the excised organs further confirmed that significant fluorescence signals of the excised liver were still observed in the fluorescence images at 24 h after treatment with DiR-NGNPs, while the fluorescence signals were much weaker in the free DiR group (). Semi-quantitative statistical plot of fluorescence showed a much higher accumulation of DiR-NGNPs than free DiR in liver (). These results suggest that DiR-NGNPs could increase the loaded drug accumulation and retention in the liver. As shown in , the maximum accumulation of nanoparticles in the liver was occurred at 12 h after injection. This may be due to the limited number of Kupffer cells and saturation of phagocytosis when a large number of nanoparticles were injected into the mice (Ouyang et al., Citation2020). The remaining portion of the nanoparticles that are not phagocytosed will reenter the systemic circulation and return to the liver later in the process. Therefore, the liver nano-deposit reached its maximum value at 12 h and was then slowly eliminated (Tsoi et al., Citation2016).
Figure 6. In vivo biodistribution of NGNPs. (A) In vivo whole-body fluorescence images at different time points and ex vivo images of major organs of mice intravenous injected with DiR solution or DiR-NGNPs. Semi-quantitative statistical plot of (B) fluorescence in vivo abdomen and (C) fluorescence of major organs. ***P < 0.001.
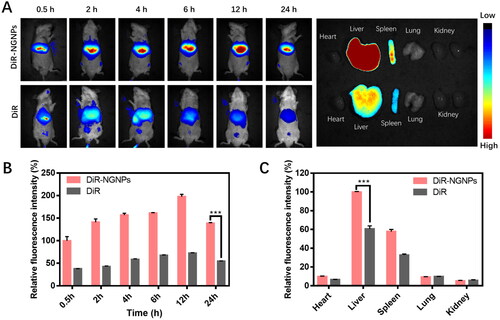
3.5. In vivo therapeutic effect of NGNPs in ALI mouse model
Based on above results, we further studied the therapeutic effect of intravenous NGNPs against APAP overdose induced liver injury to explore the potential of NGNPs as a treatment for ALI. APAP-induced ALI mice model was established in this study (). The main manifestations of ALI are liver hypertrophy, histopathological damage, and elevated levels of liver injury indicators (AKP, AST and ALT) (Shimada et al., Citation2015; Su et al., Citation2019). In addition, studies have shown that ALI can slow down hepatic blood flow rate, thereby affecting hepatic blood perfusion and further developing liver injury (Wang et al., Citation2020). Firstly, liver volume and blood flow rate were measured by color Doppler imaging (), and the results showed that liver volume increased and blood flow rate decreased in ALI mice (). After liver tissue collection, we found that ALI mice had severe liver enlargement and tissue necrosis. Liver index and liver Wet-Dry ratio were also calculated, further demonstrating severe hepatic edema in ALI mice (). H&E staining showed obvious pathological changes in the liver tissue of ALI mice, including vacuolization and cord degeneration of liver cells, focal necrosis in or around the central vein, accompanied by inflammatory cell infiltration (). The serum levels of AKP, AST and ALT in ALI mice were abnormally elevated (), suggesting impaired liver function. These results indicated that APAP-induced ALI mice model was successfully established.
Figure 7. In vivo therapeutic effect of NGNPs in ALI mouse model. (A) Experimental scheme diagram. (B) Two-dimensional ultrasonic images of Normal, Model, NG and NGNPs group. (C) Representative images of liver and the H&E staining images of liver tissue in each group (black dashed line and red arrow indicate the necrotic liver tissue). Scale bar = 100 μm. (D) The liver volume, (E) liver venous flow velocity, (F) liver index, (G) liver wet-dry ratio and (H) injury score of each group. The level of (I) AKP, (J) AST and (K) ALT in serum of each group. *P < 0.05, **P < 0.01, ***P < 0.001, ****P < 0.0001.
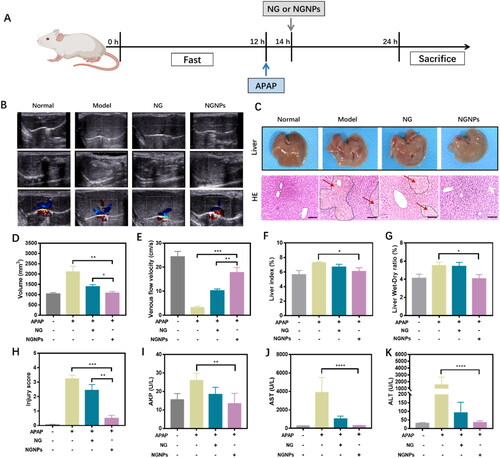
After modeling, ALI mice were randomly divided into three groups and intravenously injected with normal saline, naringenin or NGNPs (25 mg/kg). As shown in , NGNPs treatment significantly reduced liver volume and levels of liver injury indicators (AKP, AST and ALT), even comparable to that of the normal group, suggesting that NGNPs can significantly reduce the ALI severity. Subsequently, H&E staining also confirmed the therapeutic effect of NGNPs on ALI. As shown in , the liver tissue structure of ALI mice was destroyed, and liver cells were necrotic, and inflammatory cells were infiltrated. After NGNPs treatment, histopathological changes can be reversed. As shown in , the liver pathological score in the NGNPs group was significantly reduced. In addition, the hepatic blood flow rate increased significantly after NGNPs treatment. It is noteworthy that free naringenin treatment can partially reduce the severity of the liver injury but not enough to reverse the histopathological changes, and the efficacy is much lower than that of NGNPs. This may be due to the insufficient distribution of free naringenin in the liver after intravenous administration. Overall, these results suggest that NGNPs have a good therapeutic potential for ALI.
3.6. In vivo anti-apoptotic, anti-inflammatory, and anti-oxidation effects of NGNPs
Two key regulators of apoptosis, Bax and Bcl-2 proteins, were also studied with immunohistochemistry staining (). As shown in , upon NGNPs treatment, pro-apoptotic Bax was reduced, and anti-apoptotic Bcl-2 was enhanced. Further, TUNEL staining, as a robust technique, was also applied to detect apoptotic cells. As shown in , the liver tissues from ALI mice showed increased number of TUNEL positive cells, indicating an increased rate of apoptosis. Whereas, NGNPs treatment significantly reduced the cell apoptosis, indicating a stronger therapeutic effect of NGNPs compared to free drug. These results proved that NGNPs played protection role on liver cells by inhibiting cell apoptosis and kept liver healthy from acute injury.
Figure 8. Anti-inflammatory, anti-apoptotic and anti-oxidative effects of NGNPs in vivo. (A) The Bax, Bcl-2, IL-6 and IL-1β immunohistochemical staining of liver tissue in each group mice. The (B) Bax, (C) Bcl-2 positive field portion in each group mice. (D) The TUNEL fluorescence staining and (E) corresponding semi-quantitative statistics of Tunel levels. The positive field portion of (F) IL-6 and (G) IL-1β in liver tissue of mice in each group. The levels of (H) SOD, (I) MDA and (J) LDH of liver in each group mice. Scale bar = 100 μm. *P < 0.05, **P < 0.01, ***P < 0.001, ****P < 0.0001.
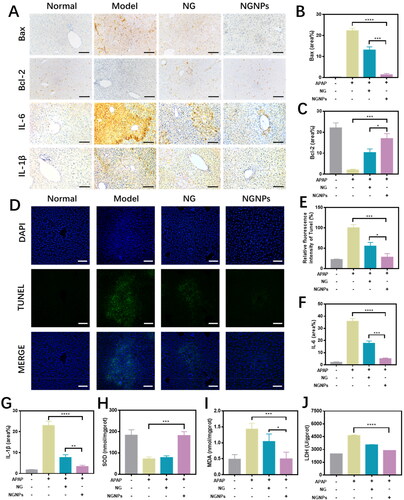
Proinflammatory cytokines were defined as critical mediators in the inflammatory process, which were released as the host defense. But abnormal release of proinflammatory cytokines will cause the activation of immune system and further release of cytokines. A sudden release of these cytokines could upregulate the inflammatory process, inducing “cytokine storm”. In in vitro studies, we have demonstrated that NGNPs could reduce the proinflammatory cytokines release from LPS-stimulated macrophages (). To evaluate the anti-inflammatory effect of NGNPs in treating ALI mice, we used immunohistochemical staining to assess the expression levels of proinflammatory factors, including IL-6 and IL-1β, in liver tissues. As shown in , the levels of proinflammatory factors (IL-6 and IL-1β) were much higher in the model group as compared to those in normal group. Treatment with naringenin and NGNPs can inhibit the production and release of these cytokines. Semi-quantitative analysis data () also confirmed that NGNPs had higher anti-inflammatory activity.
Free oxygen radicals play an important role in the pathogenesis of ALI. MDA is one of the final products of oxygen radical chain reaction and lipid peroxidation reaction. Increased free radicals often caused MDA overproduction. Thus, MDA levels is commonly known as a marker of oxidative stress (Ji et al., Citation2021; Ma et al., Citation2021). SOD is the first detoxification enzyme and play a major role in fighting free radical damage and lipid peroxidation. Also, LDH is a stable cytoplasmic enzyme, which would be rapidly released when injury damages the cells. Therefore, the high levels of LDH in the extracellular fluid or blood indicated the tissue damage during ALI progress (Gao et al., Citation2018). Therefore, oxidative stress levels in liver tissues can be measured by SOD activity, MDA and LDH levels. In vitro data () has suggested that NGNPs treatment could decrease the LDH release and intracellular MDA levels and increase the anti-oxidative SOD activity in liver cells upon H2O2 injury. As shown in , after APAP overdose, SOD activity was inhibited, while MDA and LDH production was promoted. After naringenin or NGNPs treatment, SOD activity was increased while MDA and LDH levels were decreased. Notably, NGNPs showed stronger antioxidant and liver protective effects. This may be due to the higher concentration of NGNPs in liver compared with free naringenin, which can achieve the optimal therapeutic concentration.
3.7. In vivo safety assessment of NGNPs
Finally, we investigated whether NGNPs is toxic to other organs after intravenous administration. Mice injected intravenously with high doses (100 mg/kg) of NGNPs had a stable body weight during the observation period () and did not exhibit any abnormal behavior. After NGNPs administration, the contents of AKP (), ALT () and AST () in serum remained within the normal range, indicating normal liver function. Hearts, livers, spleens, lungs, and kidneys were collected and stained with H&E ().These organs in NGNPs group showed normal tissue structure with no significant difference compared to the health control, further confirming the excellent biocompatibility and safety of NGNPs.
Figure 9. In vivo safety assessment of NGNPs. (A) Weight change in normal mice and mice injected with high dose NGNPs for 14 days. The level of (B) AKP, (C) ALT and (D) AST of in serum of each group. (E) H&E staining of heart, liver, spleen, lung and kidney of mice in each group. n.s., no statistical significance.
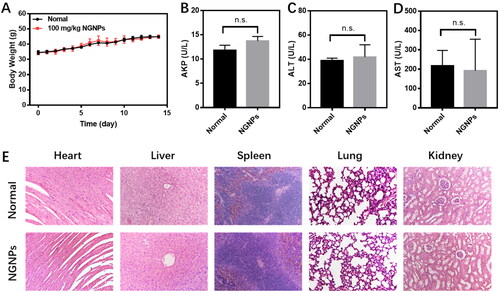
4. Conclusion
Naringenin possesses powerful anti-inflammatory and antioxidant properties and has therapeutic potential in both inflammatory related diseases and oxidative stress-induced diseases. However, naringenin has limited its application in the medical field due to its poor water solubility and low bioavailability. Therefore, in this study, we designed bovine serum albumin-based naringenin nanoparticles (NGNPs), which could increase liver accumulation by the mononuclear phagocyte system and continuously reach effective concentrations. The constructed NGNPs significantly enhanced the resistance of liver cells to excessive oxidative stress and proinflammatory stimulation. Meanwhile, NGNPs significantly alleviated the disease progression of ALI and inhibited liver cell apoptosis in ALI mouse models. In conclusion, naringenin nanoparticles with a simple preparation method have great potential in treating ALI.
Disclosure statement
No potential conflict of interest was reported by the authors.
Additional information
Funding
References
- Ahmad A, Fauzia E, Kumar M, et al. (2019). Gelatin-coated polycaprolactone nanoparticle-mediated naringenin delivery rescue human mesenchymal stem cells from oxygen glucose deprivation-induced inflammatory stress. ACS Biomater Sci Eng 5:683–95.
- Brodersen R. (1974). Competitive binding of bilirubin and drugs to human serum albumin studied by enzymatic oxidation. J Clin Invest 54:1353–64.
- Budel RG, daSilva DA, Moreira MP, et al. (2020). Toxicological evaluation of naringin-loaded nanocapsules in vitro and in vivo. Colloids Surf B Biointerfaces 188:110754.
- Chen M, Li R, Gao Y, et al. (2021). Encapsulation of hydrophobic and low-soluble polyphenols into nanoliposomes by pH-driven method: Naringenin and naringin as model compounds. Foods 10:963.
- Chen R, Zhai Y-Y, Sun L, et al. (2022). Alantolactone-loaded chitosan/hyaluronic acid nanoparticles suppress psoriasis by deactivating STAT3 pathway and restricting immune cell recruitment. Asian J Pharm Sci 17:268–83.
- Cordenonsi LM, Bromberger NG, Raffin RP, Scherman EE. (2016). Simultaneous separation and sensitive detection of naringin and naringenin in nanoparticles by chromatographic method indicating stability and photodegradation kinetics. Biomed Chromatogr 30:155–62.
- da Silva NIO, Salvador EA, Rodrigues Franco I, et al. (2018). Bovine serum albumin nanoparticles induce histopathological changes and inflammatory cell recruitment in the skin of treated mice. Biomed Pharmacother 107:1311–7.
- Gao Y, Liu W, Wang W, et al. (2018). Polyguluronate sulfate (PGS) attenuates immunological liver injury in vitro and in vivo. Int J Biol Macromol 114:592–8.
- Ge Y, Chen H, Wang J, et al. (2021). Naringenin prolongs lifespan and delays aging mediated by IIS and MAPK in Caenorhabditis elegans. Food Funct 12:12127–41.
- Goncalves C, Ramalho MJ, Silva R, et al. (2021). Lipid nanoparticles containing mixtures of antioxidants to improve skin care and cancer prevention. Pharmaceutics 13:2042.
- Gratieri T, Pinho LAG, Oliveira MA, et al. (2020). Hydroxypropyl-beta-cyclodextrin-complexed naringenin by solvent change precipitation for improving anti-inflammatory effect in vivo. Carbohydr Polym 231:115769.
- Hua YQ, Zeng Y, Xu J, Xu XL. (2021). Naringenin alleviates nonalcoholic steatohepatitis in middle-aged Apoe(-/-)mice: role of SIRT1. Phytomedicine 81:153412.
- Huang Y, Hu L, Huang S, et al. (2018). Curcumin-loaded galactosylated BSA nanoparticles as targeted drug delivery carriers inhibit hepatocellular carcinoma cell proliferation and migration. Int J Nanomedicine 13:8309–23.
- Huang ZW, Shi Y, Zhai YY, et al. (2021). Hyaluronic acid coated bilirubin nanoparticles attenuate ischemia reperfusion-induced acute kidney injury. J Control Release 334:275–89.
- Ji C-l, Dai S, Liu H, et al. (2021). Polyphenols from Securidaca inappendiculata alleviated acute lung injury in rats by inhibiting oxidative stress sensitive pathways. Chin Herb Med 13:381–8.
- Khan AW, Kotta S, Ansari SH, et al. (2015). Self-nanoemulsifying drug delivery system (SNEDDS) of the poorly water-soluble grapefruit flavonoid Naringenin: design, characterization, in vitro and in vivo evaluation. Drug Deliv 22:552–61.
- Kim JW, Yang D, Jeong H, et al. (2019). Dietary zerumbone, a sesquiterpene, ameliorates hepatotoxin-mediated acute and chronic liver injury in mice. Phytother Res 33:1538–50.
- Kou L, Huang H, Tang Y, et al. (2022). Opsonized nanoparticles target and regulate macrophage polarization for osteoarthritis therapy: A trapping strategy. J Control Release 347:237–55.
- Kou L, Jiang X, Tang Y, et al. (2022). Resetting amino acid metabolism of cancer cells by ATB0,+-targeted nanoparticles for enhanced anticancer therapy. Bioact Mater 9:15–28.
- Kumar RP, Abraham A. (2017). Inhibition of LPS induced pro-inflammatory responses in RAW 264.7 macrophage cells by PVP-coated naringenin nanoparticle via down regulation of NF-kappaB/P38MAPK mediated stress signaling. Pharmacol Rep 69:908–15.
- Kumar SP, Birundha K, Kaveri K, Devi KT. (2015). Antioxidant studies of chitosan nanoparticles containing naringenin and their cytotoxicity effects in lung cancer cells. Int J Biol Macromol 78:87–95.
- Li S, Hong M, Tan HY, et al. (2016). Insights into the role and interdependence of oxidative stress and inflammation in liver diseases. Oxid Med Cell Longev 2016:4234061.
- Ma H, Yang B, Yu L, et al. (2021). Sevoflurane protects the liver from ischemia-reperfusion injury by regulating Nrf2/HO-1 pathway. Eur J Pharmacol 898:173932.
- Ma X, Ren X, Guo X, et al. (2019). Multifunctional iron-based Metal-Organic framework as biodegradable nanozyme for microwave enhancing dynamic therapy. Biomaterials 214:119223.
- Md S, Abdullah S, Awan ZA, Alhakamy NA. (2022). Smart Oral pH-responsive dual layer nano-hydrogel for dissolution enhancement and targeted delivery of naringenin using protein-polysaccharides complexation against colorectal cancer. J Pharm Sci 111:3155–64.
- Ni D, Wei H, Chen W, et al. (2019). Ceria nanoparticles meet hepatic ischemia-reperfusion injury: The perfect imperfection. Adv Mater 31:e1902956.
- Niu X, Sang H, Wang J. (2021). Naringenin attenuates experimental autoimmune encephalomyelitis by protecting the intact of blood-brain barrier and controlling inflammatory cell migration. J Nutr Biochem 89:108560.
- Ouyang B, Poon W, Zhang YN, et al. (2020). The dose threshold for nanoparticle tumour delivery. Nat Mater 19:1362–71.
- Pan Z, He Q, Zeng J, et al. (2022). Naringenin protects against iron overload-induced osteoarthritis by suppressing oxidative stress. Phytomed 105:154330.
- Peng J, Li J, Huang J, et al. (2019). p300/CBP inhibitor A-485 alleviates acute liver injury by regulating macrophage activation and polarization. Theranostics 9:8344–61.
- Shimada Y, Tomita M, Yoshida T, et al. (2015). Inhibition of lipopolysaccharide-induced liver injury in rats treated with a hepatic drug-metabolizing enzyme inducer p,p’-DDT. Exp Toxicol Pathol 67:245–51.
- Su L, Mao J, Hao M, et al. (2019). Integrated plasma and bile metabolomics based on an UHPLC-Q/TOF-MS and network pharmacology approach to explore the potential mechanism of Schisandra chinensis-protection from acute alcoholic liver injury. Front Pharmacol 10:1543.
- Tan T, Yang Q, Chen D, et al. (2021). Chondroitin sulfate-mediated albumin corona nanoparticles for the treatment of breast cancer. Asian J Pharm Sci 16:508–18.
- Tong F, Tang X, Liu D. (2019). Phycocyanin/PEG-b-(PG-g-PEI) attenuated hepatic ischemia/reperfusion-induced pancreatic islet injury and enlarged islet functionality. Int J Nanomedicine 14:339–51.
- Tsoi KM, MacParland SA, Ma XZ, et al. (2016). Mechanism of hard-nanomaterial clearance by the liver. Nat Mater 15:1212–21.
- Veiko AG, Lapshina EA, Zavodnik IB. (2021). Comparative analysis of molecular properties and reactions with oxidants for quercetin, catechin, and naringenin. Mol Cell Biochem 476:4287–99.
- Wang H, Burke LJ, Patel J, et al. (2020). Imaging-based vascular-related biomarkers for early detection of acetaminophen-induced liver injury. Theranostics 10:6715–27.
- Wang H, He Y, Hou Y, et al. (2020). Novel self-nanomicellizing formulation based on Rebaudioside A: A potential nanoplatform for oral delivery of naringenin. Mater Sci Eng C Mater Biol Appl 112:110926.
- Wang J, Zhang B. (2018). Bovine serum albumin as a versatile platform for cancer imaging and therapy. Curr Med Chem 25:2938–53.
- Wang K, Chen Z, Huang L, et al. (2017). Naringenin reduces oxidative stress and improves mitochondrial dysfunction via activation of the Nrf2/ARE signaling pathway in neurons. Int J Mol Med 40:1582–90.
- Xiao J, Shi J, Cao H, et al. (2007). Analysis of binding interaction between puerarin and bovine serum albumin by multi-spectroscopic method. J Pharm Biomed Anal 45:609–15.
- Yang F, Hu S, Sheng X, Liu Y. (2020). Naringenin loaded multifunctional nanoparticles to enhance the chemotherapeutic efficacy in hepatic fibrosis. Biomed Microdevices 22:68.
- Yang J, Li Q, Zhou XD, et al. (2011). Naringenin attenuates mucous hypersecretion by modulating reactive oxygen species production and inhibiting NF-κB activity via EGFR-PI3K-Akt/ERK MAPKinase signaling in human airway epithelial cells. Mol Cell Biochem 351:29–40.
- Yao Q, Jiang X, Zhai YY, et al. (2020). Protective effects and mechanisms of bilirubin nanomedicine against acute pancreatitis. J Control Release 322:312–25.
- Yao Q, Lan QH, Jiang X, et al. (2020). Bioinspired biliverdin/silk fibroin hydrogel for antiglioma photothermal therapy and wound healing. Theranostics 10:11719–36.
- Yen FL, Wu TH, Lin LT, et al. (2009). Naringenin-loaded nanoparticles improve the physicochemical properties and the hepatoprotective effects of naringenin in orally-administered rats with CCl(4)-induced acute liver failure. Pharm Res 26:893–902.
- Zaidun NH, Thent ZC, Latiff AA. (2018). Combating oxidative stress disorders with citrus flavonoid: Naringenin. Life Sci 208:111–22.
- Zeng W, Jin L, Zhang F, et al. (2018). Naringenin as a potential immunomodulator in therapeutics. Pharmacol Res 135:122–6.
- Zhang W, Jiang P, Chen Y, et al. (2016). Suppressing the cytotoxicity of CuO nanoparticles by uptake of curcumin/BSA particles. Nanoscale 8:9572–82.
- Zhong W, Qian K, Xiong J, et al. (2016). Curcumin alleviates lipopolysaccharide induced sepsis and liver failure by suppression of oxidative stress-related inflammation via PI3K/AKT and NF-kappaB related signaling. Biomed Pharmacother 83:302–13.
- Zobeiri M, Belwal T, Parvizi F, et al. (2018). Naringenin and its nano-formulations for fatty liver: Cellular modes of action and clinical perspective. Curr Pharm Biotechnol 19:196–205.