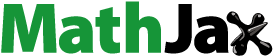
Abstract
Glaucoma is the leading cause of blindness worldwide. However, its surgical treatment, in particular via trabeculectomy, can be complicated by fibrosis. In current clinical practice, application of the drug, Mitomycin C, prevents or delays fibrosis, but can lead to additional side effects, such as bleb leakage and hypotony. Previous in silico drug screening and in vitro testing has identified the known antibiotic, josamycin, as a possible alternative antifibrotic medication with potentially fewer side effects. However, a suitable ocular delivery mechanism for the hydrophobic drug to the surgical site does not yet exist. Therefore, the focus of this paper is the development of an implantable drug delivery system for sustained delivery of josamycin after glaucoma surgery based on crosslinked γ-cyclodextrin. γ-Cyclodextrin is a commonly used solubilizer which was shown to complex with josamycin, drastically increasing the drug’s solubility in aqueous solutions. A simple γ-cyclodextrin crosslinking method produced biocompatible hydrogels well-suited for implantation. The crosslinked γ − cyclodextrin retained the ability to form complexes with josamycin, resulting in a 4-fold higher drug loading efficiency when compared to linear dextran hydrogels, and prolonged drug release over 4 days.
1. Introduction
Glaucoma is one of the main causes of irreversible visual impairment. The most common type of glaucoma, primary open angle glaucoma, has an estimated global prevalence of 2% (Kapetanakis et al., Citation2016). Common surgical interventions to reduce the high intraocular pressure associated with glaucoma include trabeculectomy or stent placement (Bell et al., Citation2021). However, local fibrosis and scarring processes in response to the surgery can impair the draining function of these treatments. To prevent fibrosis, it is standard practice during and after surgery to treat the patients with locally applied cytostatic or cytotoxic drugs.
5-Fluorouracil (5-FU) and mitomycin C (MMC), in combination with trabeculectomy, have been shown to achieve a long-term reduction in intraocular pressure (Reibaldi et al., Citation2008). MMC, a cytotoxic antibiotic, can be applied in a single, intraoperative dose and is currently considered the gold standard agent for use during trabeculectomy (Mallone et al., Citation2021; Vinod et al., Citation2017). However, MMC acts nonspecifically by irreversibly intercalating between the DNA double strands of cells, ultimately leading to cell death (Wolters et al., Citation2021). Therefore, despite contributing to significant improvements in the treatment of glaucoma, the use of MMC is associated with treatment-limiting side effects, including bleb leakage, hypotony and endophthalmitis (Bell et al., Citation2021; Yoon & Singh, Citation2004). Given these side effects, there is a high medical need for alternative, less cytotoxic drugs capable of more targeted suppression of fibrosis.
In our preliminary work, in silico drug screening based on gene expression datasets from a fibrosis model employing human Tenon fibroblasts was used to identify new antifibrotic drug candidates that could downregulate the processes in fibroblasts known to contribute to fibrosis (Stahnke et al., Citation2020). The primary candidate to emerge from this analysis was josamycin (JM), which is a 16-membered ring macrolide antibiotic that has been used clinically since 1970. However, our work demonstrated that JM also has antifibrotic effects, suppressing proliferation and fibrosis in human Tenon fibroblasts in vitro (Stahnke et al., Citation2020; Stahnke et al., Citation2012). These results position JM as a promising new agent for specific prevention of fibrosis after surgical glaucoma treatment.
While MMC is administered in a single application during surgery, most strategies involving alternative antifibrotic medications employ repetitive applications for days or weeks following surgery (Lama & Fechtner, Citation2003; Seibold et al., Citation2012). However, frequent visits to the doctor for subconjunctival injections are not time or cost effective and medicated eye drops face issues with efficient drug delivery and patient compliance (Jumelle et al., Citation2020; Schwartz & Quigley, Citation2008). For these reasons, it was our goal to develop a drug delivery system (DDS) that could be implanted at the eye at the time of glaucoma filtration surgery and provide sustained, local delivery of JM. The subconjunctival space is commonly used for drug delivery after ocular surgery and can accommodate injected solutions and solid implants (Kopp et al., Citation2017; S Khouri et al., Citation2017). Subconjunctival implants have been shown to be a convenient and efficient method for sustained ocular drug delivery and a few subconjuctival hydrogel-based implants are already commercially available (Rafiei et al., Citation2020; Reitsamer et al., Citation2019; Zelefsky et al., Citation2008).
One hurdle in designing a DDS is that JM is poorly soluble in water, limiting its incorporation into hydrogel systems. However, γ-cyclodextrin (γCD) has been shown to increase the solubility of JM in aqueous solutions (El Harti et al., Citation2012). The naturally occurring cyclic oligosaccharides, α-cyclodextrin (αCD), β-cyclodextrin (βCD) and γCD, are comprised of 6, 7, or 8 glucose subunits, respectively. The cyclodextrins form a truncated cone structure with a hydrophobic core which can form complexes with hydrophobic molecules, increasing their solubility in aqueous solutions. γCD is the most soluble and largest of the naturally occurring cyclodextrin molecules. Additionally, of the three naturally occurring, unmodified cyclodextrins, γCD is the only one that can be degraded by the α-amylase enzyme (Marshall & Miwa, Citation1981; Loftsson & Stefánsson, Citation2017; Lumholdt et al., Citation2012) and has been found to be the most biocompatible (Antlsperger & Schmid, Citation1996; Leroy-Lechat et al., Citation1994; Saarinen-Savolainen et al., Citation1998). γCD is considered GRAS (generally recognized as safe) by the FDA and is used in many food and cosmetic products as well as drug formulations (Saokham & Loftsson, Citation2017).
The unique structure and properties of cyclodextrins have been utilized in the fabrication of hydrogels with the goals of increasing drug loading, specifically of poorly water soluble drugs, and prolonging drug release (Rohner et al., Citation2020). γCD can be directly crosslinked utilizing its many available hydroxyl groups without losing the molecule’s ability to form complexes (Haley et al., Citation2020; Machín et al., Citation2013; Mennini et al., Citation2016; Wintgens & Amiel, Citation2010). Additionally, γCD nanoparticles have been investigated for drug delivery in the eye in the form of eye drops, which have proved to be well tolerated in clinical tests (Fang et al., Citation2022; Gudmundsdottir et al., Citation2014; Loftsson & Stefánsson, Citation2017). For these reasons, we have chosen γCD as the ideal matrix for developing a novel DDS for JM. It is hypothesized that γCD-based hydrogels will have an improved loading capacity due to complex formation between γCD and JM. Additionally, it is expected that this complexation will slow the release of JM and extend the drug release profile.
Here we utilize a simple, one-pot crosslinking method to develop a γCD hydrogel based on epichlorohydrin crosslinking for the release of JM (see Graphical Abstract). γCD maintained the ability to complex with JM after crosslinking, resulting in a highly swellable hydrogel with a high JM loading efficiency despite the drug’s low water solubility. Additionally, the γCD gel significantly extends the release of JM in vitro, demonstrating drug delivery over several days. The γCD hydrogel does not support direct cell growth, but was shown to be biocompatible in our study. The rate of enzymatic degradation of the γCD gel was investigated and shown to be insufficient for the intended application so future work will address increasing degradability. The characterization completed in this work demonstrated the potential of γCD hydrogels for extended JM delivery. The simple and flexible fabrication method will allow for easy modification for further development and application. Critically, the developed γCD-based DDS will facilitate future in vivo evaluation of the efficacy of JM as a novel antifibrotic by allowing for the continuous, prolonged release of the drug after glaucoma surgery. This, in turn, will enable a better definition of the therapeutic targets for the drug’s delivery and allow for further refinement of the γCD hydrogel. The characterization done here is a crucial first step in the development of a new treatment for post-surgical ocular fibrosis.
2. Methods
2.1. Materials
γ-Cyclodextrin (γCD) was manufactured by Wacker Chemie AG (MW: 1297.12 g/mol, 98%, Munich, Germany). Josamycin (JM, MW: 827.99 g/mol) was purchased from Chemodex (St. Gallen, Switzerland). Sodium hydroxide, calcium chloride, epichlorohydrin (EPI), Dulbecco’s phosphate-buffered saline (PBS), and α-amylase enzyme (Type VI-B from porcine pancreas) were all purchased from Sigma-Aldrich (Darmstadt, Germany). Additionally, dextran (MW: ∼70,000 g/mol), HEPES and sodium chloride were purchased from Carl Roth (Karlsruhe, Germany). The SYLGARD 184 Silicone Elastomer Kit from Dow (Midland, USA) was used as per the manufacturer’s instructions. Dulbecco’s Modified Eagle Medium (DMEM), fetal calf serum and penicillin-streptomycin were purchased from PAN BIOTECH (Aidenbach, Germany) and the CellQuanti-Blue Cell Viability Assay Kit was purchased from BioAssay Systems (Biotrend Chemicals GmbH, Cologne, Germany) BMG LABTECH (Ortenberg, Germany).
2.2. Ultra high performance liquid chromatography (UHPLC) method for measuring JM concentration
JM concentration was measured using a UHPLC LC30 (Shimadzu, Kyoto, Japan) consisting of two Nexera X2 LC30AD pump modules, a DGU-20A3R Degassing Unit, a Nexera X2 SIL30AC autosampler, a prominence SPD-M20A digital array detector, and a prominence CTO-20AC column oven. A 4 min, isocratic method was used with 47% acetonitrile in deionized (DI) water with 0.05% formic acid as the mobile phase and a flow rate of 0.3 mL/min through a Kinetex 2.6 µm, C18 100 Å, 150 × 2.1 mm column (Phenomenex, Aschaffenburg, Germany) heated to 40 °C. Samples injections of 1 or 10 µl volumes were used. JM was detected at a retention time of Rt = 2.5 min at a detection wavelength of λ = 232 nm. Calibration was done with 5 samples in a concentration range between 0.1 and 0.0125 mg/mL.
2.3. Measuring phase solubility of JM with various cyclodextrins
Solutions between 12.5-150 mg/mL of γCD were made in DI water. An excess amount of JM was added to 1 mL of each tested cyclodextrin solution and each concentration was tested in triplicate. Additionally, excess JM in pure DI water was tested under the same conditions to determine the intrinsic solubility. All samples were sonicated for 5 min and mixed at room temperature for 3 days. Then samples were sonicated again for 5 min and mixed for an additional 10 min before filtering through a 0.45 µm syringe filter. Samples were then diluted in ethanol, mixed for 30 min and centrifuged for 5 min at 5000 rpm to remove precipitated γCD and the JM concentration of the supernatant was measured.
The phase-solubility profile for JM was analyzed based on the general method outlined in Loftsson et al. to determine the stability constant (K1:1), complex efficiency (CE), and cyclodextrin molar ratio (Loftsson et al., Citation2005). K1:1 can be used to quantify the affinity of JM for γCD and is defined by EquationEquation 1(1)
(1) :
(1)
(1)
Where S0 is the intrinsic solubility of the drug in the solution. Slope is based on the linear regression of γCD molar concentration versus the corresponding total molar concentration of JM. The CE was calculated based on EquationEquation 2(2)
(2) :
(2)
(2)
Where [drug/CD] is the concentration of dissolved complex, and [CD] is the concentration of dissolved free cyclodextrin. The CE fundamentally represents the ratio of complexed CD molecules to free unbound CD molecules so this value was also used to calculate that molar ratio of complexed to free CDs.
2.4. JM aqueous stability measurements
JM stability in aqueous solution was tested with and without γCD. 1 mg/mL JM was dissolved in PBS by mixing at room temperature for 24 h, sonicating for 5 min, and subsequently filtered with a 0.45 µm syringe filter. A 10 mg/mL JM solution was prepared similarly with 100 mg/mL γCD in PBS. Both solutions were separated into aliquots in glass containers and stored in the dark at 4, 25 or 37 °C (n = 3). Before sampling at 7, 13, 14, 21 and 35 days, the aliquots were allowed to come to room temperature and were sonicated for 5 min. The JM concentrations were measured and compared to the initial concentration of the solutions.
2.5. Hydrogel fabrication
γCD hydrogels were prepared by first dissolving γCD in a 30% w/v NaOH to obtain a final solution that was 50% w/w γCD. Under basic conditions, EPI reacts with the hydroxyl groups on the γCD, resulting in the formation of glyceryl bridges between CD molecules (Renard et al., Citation1997). EPI was added to the γCD solution to reach a 1:10 molar ratio of γCD:EPI. The mixture was stirred for 80 min at room temperature and was then pipetted into custom-made silicone molds made with a SYLGARD 184 kit, which had cylindrical (9 mm diameter x 1 mm height) wells. 70 µl of γCD hydrogel solution was pipetted into each well and the mixture was allowed to finish crosslinking, covered, overnight at room temperature. The solidified γCD hydrogels were then washed at 37 °C in DI water twice for 1 hour and once overnight before being dried overnight at room temperature on a flat piece of silicone.
For comparison, dextran hydrogels were fabricated by first dissolving dextran in 30% w/v NaOH to obtain a final solution that was 30% w/w dextran and then adding EPI to reach a 1:446 ratio of dextran:EPI and mixing for 30 min. Then the mixture was molded in the same way as the γCD gels and allowed to set at room temperature for 3 h. Dextran gels were washed and dried in the same manner as the γCD gels.
To view interior morphology, hydrated γCD gels were frozen with liquid nitrogen and then lyophilized before being imaged with a QUANTA FEG 250 system (FEI Company, Frankfurt, Germany) scanning electron microscope (SEM). Samples were gold sputtered prior to SEM imaging under high vacuum and 10 kV with a working distance of 10 mm.
2.6. Hydrogel swelling and mechanical characterization
The swelling ratio of the γCD and dextran gels was measured gravimetrically at room temperature. Individual gels were weighed dry and then placed in water on a shaker for 24 h. Then the gels were blotted on tissue paper and weighed again to determine the hydrated weight. For γCD gels, 30 samples from 5 different batches were measured. For dextran gels, 3 samples from one batch were tested. The swelling ratio was calculated with the EquationEquation 3(3)
(3) :
(3)
(3)
where Ww is the weight of wet, completely hydrated gels and Wd is the weight of the dry hydrogel. The effect of multiple cycles of swelling and drying on the γCD gels was tested by fully hydrating and drying the same gels for 5 cycles, weighing the mass at each step (n = 9).
Compression testing of γCD hydrogels was performed under ambient conditions in air using a single-column ZwickiLine Z 2.5 testing machine (Zwick/Roell, Ulm, Germany) equipped with a 1 kN load cell and two compression plates at a test speed of 2 mm/min (n = 8). The disk shaped gels were dried for storage and rehydrated prior to testing using 1 mL deionized water, resulting in specimens with 11 mm diameter and 1 mm height when fully hydrated. The compressive modulus was determined by performing a linear regression between 0 and 10% deformation in the resulting stress-deformation curves (Worthington et al., Citation2014). Percent deformation at first breaking point of the samples was determined and averaged.
2.7. Josamycin loading of hydrogels
Hydrogels were loaded with drug by rehydrating in a saturated JM solution. The loading solution was prepared by mixing excess JM in DI water, sonicating for 5 min and mixing at room temperature for 24 h. Then the loading solution was sonicated again for 5 min and filtered with a 0.45 µm syringe filter before use, resulting in a JM concentration of 868 ± 23 µg/mL. Each dry hydrogel was placed in 1 mL of JM loading solution and left overnight on a shaker at room temperature. The final JM concentration and remaining mass of the loading solution after loading was used to calculate the amount of JM loaded into each gel. 30 samples from 5 batches of γCD gels were tested and 6 samples from one batch of dextran gel were tested. Loaded gels were dried completely and stored at room temperature. To measure the change in loading solution concentration over time, the same JM loading procedure was followed as described above and 10 µl samples were taken 1, 3, 6, 21, 30 and 48 h after loading (n = 3).
Dried, γCD loaded gels were stored in the dark at room temperature to test long-term stability of loaded γCD gels. At each time point, dried gels were placed in 3 mL of 50% ethanol on a shaker overnight at room temperature. The ethanol release solution was collected and replaced for a second incubation overnight. The mass of the release solution and the JM concentration were used to calculate the total amount of JM released from the samples and compared to the original amount loaded (n = 5).
2.8. In vitro JM release from hydrogels
In vitro release of JM was measured by placing dried, JM-loaded gels in 2 mL of PBS at 37 °C with shaking. JM release was measured at 0.5, 2, 6, 24, 48, 72, 96, 168 and 192 h. At each time point, the release medium was completely collected and replaced. The JM concentration, measured by HPLC, and mass of the release solution was used to calculate the total JM released at each time point (n = 15 for γCD gels and n = 3 for dextran gels).
2.9. Biocompatibility γCD gels
Commercially available mouse L929 fibroblasts (ATCC®, CCL-1, Manassas, USA) were used for biocompatibility tests. Cells were cultured in DMEM (with 4.5 g/l glucose and 3.7 g/l NaHCO3) supplemented with 10% fetal calf serum and 1% penicillin-streptomycin and incubated at 37 °C (5% CO2). Culture medium was changed every 2 to 3 days.
To test direct contact biocompatibility, pieces of γCD hydrogel, which were not loaded with JM, were cut to fit in a 96 well plate. Then these gels were sterilized by 5 min incubation in 70% ethanol, followed by rinsing twice with sterile PBS. The gels were then soaked overnight in the cell culture medium, before placing the gels in a 96 well plate and seeding with 5000 cells per well. Cells were incubated for 48 h before imaging.
The cytotoxicity of γCD gels was further tested based on the eluent medium test protocol outlined in the ISO 10993-5 (International Organization for Standardization, Citation2009). Eluent medium was prepared by incubating 0.1 g/mL of dried, unloaded γCD hydrogel in complete medium for 48 h at 37 °C according to ISO 10993-12:2021 (International Organization for Standardization, Citation2021). 1x104 cells were added to each well (n = 4 wells) in a standard 96 well plate and grown under standard conditions for 24 h before replacing the normal medium with eluent medium. Cell culture polystyrene was used as a negative control and a cytotoxic eluate made from polyurethane film loaded with 0.1% zinc diethyldithiocarbamate (Hatano Research Institute, Ochiai, Japan) was used as a positive control as outlined in the ISO 10993-5. Measurements from high-density polyethylene (Hatano Research Institute, Ochiai, Japan) as a negative control were used as a reference for calculating cell viability. After incubation in eluent medium for 48 h, cell viability was measured using the CellQuanti-Blue Cell Viability Assay Kit according to the manufacturer’s instructions. Briefly, 10% CellQuanti-Blue supplement was added to each well and incubated for 2 h before measuring the fluorescence (excitation 544 nm, emission 590 nm) with a Fluostar optima (BMG LABTECH, Ortenberg, Germany). Results from 4 replicate wells were tested for normal distribution and with the Nalimov test for outliers before calculating mean values.
2.10. Enzymatic degradation of γCD gels
To measure enzymatic degradation, γCD gels were incubated in α-amylase solution. The enzyme degradation solution was based on previous studies of the degradation of soluble γCD with α-amylase, but with a higher concentration of enzyme to accelerate degradation (Sripetch et al., Citation2020). An enzyme solution of 10 U/mL α-amylase (Type VI-B from porcine pancreas) in degradation buffer (20 mM HEPES buffer containing 140 mM NaCl and 2 mM CaCl2, pH 7.4) was used. Samples were incubated at 37 °C in 3 mL of enzyme solution (n = 6). Control γCD gels (n = 6) were incubated in degradation buffer without enzyme added. The degradation buffer was changed every 2 to 4 weeks. Every 4 weeks, the samples were washed 3 times with ∼10 mL of DI water then left at room temperature to dry completely. The bottoms of the vials used for the degradation experiment had been coated with a layer of silicone (SYLGARD 184) which allowed for drying the gels directly in the vials, minimizing damage from handling. Then the dry masses of the samples were recorded before replacing the degradation buffer and returning the samples to 37 °C.
2.11. Statistics
In all cases, data were reported as the mean ± standard deviation. Comparison of groups in drug loading and long-term storage data was done with a Student’s t-test. The cyclic swelling data was analyzed with a 1-way ANOVA. All other data were analyzed by 2-way ANOVA and, when significant results were indicated, post hoc comparisons of experimental groups at each time point were performed with Bonferroni correction for multiple comparisons. P-values of <0.05 were considered significant. R and Rstudio were used for all statistical analysis and graphic representation (R Core Team, Citation2022; RStudio Team, Citation2020).
3. Results and discussion
3.1. Josamycin-cyclodextrin complex characterization
JM is a poorly water soluble drug with an estimated LogP value of 2.9 (Cheng et al., Citation2007). γCD is commonly used to increase the concentration of poorly water-soluble drugs by forming inclusion complexes. The relationship between γCD concentration and the resulting maximum JM concentration is shown in the phase solubility curve in . This relationship was linear (R2 > 0.99), which indicates a predominately 1:1 binding ratio (Loftsson et al., Citation2005). Based on the slope of the linear fit of this curve, the stability constant (K1:1 =149.7 M−1) and complexation efficiency (CE = 0.19) were calculated according to EquationEquations 1(1)
(1) and Equation2
(2)
(2) based on the measured intrinsic solubility of JM (1.29 ± 0.18 mM). From the CE, the molar ratio of complexed γCD to free γCD was calculated (Molar ratio = 1:5). Over the range tested, γCD resulted in a more than 13-fold increase of JM concentration compared to the intrinsic solubility of the drug in water (from 1.29 to 19.9 mM).
Figure 1. Characterization of γCD-JM complex formation and JM stability in aqueous solutions. (A) Phase solubility curve of josamycin in aqueous solution with γCD. (B) JM stability in aqueous solutions at 4 °C (blue points), 25 °C (black points) and 37 °C (red points) was measured in γCD (solid lines) and PBS only (dashed lines) solutions.
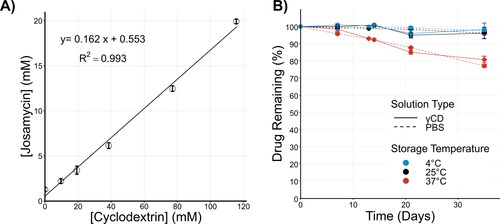
To confirm that complex formation between γCD and JM has no effect on JM stability in aqueous solution, JM was stored in PBS or γCD solutions at various temperatures. UHPLC measurement of the JM concentration out to 35 days showed that JM degrades at the same rate in PBS and γCD solutions (). In general, the JM concentration was stable during the experimental period at 4 and 25 °C, but a reduction in measured concentration indicated degradation over time when stored at 37 °C. After 35 days, the final JM concentrations at 37 °C as a percent of the initial concentration were 77.95 ± 0.8% and 80.69 ± 1.9% for PBS and γCD solutions, respectively. JM showed the same pattern of degradation with or without γCD, demonstrating that γCD has no negative effect on JM stability, but also that it was not protective.
3.2. Physical characterization of γCD hydrogels
γCD could be easily crosslinked with EPI to form a hydrogel using a one-pot method. The polycondensation reaction between the EPI and the hydroxyl groups on the γCD results in glyceryl bridges between γCD molecules (Renard et al., Citation1997). At the high concentration of NaOH used, the crosslinking reaction is expected to occur more often between secondary hydroxyl groups (Yang et al., Citation2013). The gels were initially molded in a disk form with a diameter of 9 mm and height of 1 mm. After washing, the resulting hydrogel was transparent in dry and hydrated states (). The handling properties of the gel are compatible with the intended implantation application. When dry, the gels are strong enough to handle with tweezers without breaking. When hydrated, the gels become soft enough to bend and conform to their surroundings. SEM imaging of the interior of a freeze-dried hydrogel shows a consistent porous structure (). The γCD gels had high swelling ratios with an average swelling ratio of 4.33 ± 0.63 (). Each point on the graph of hydrogel swelling represents one replicate (one hydrogel disk) and the points are color coded by batch. It is clear that there is more variability between batches than within. This was likely due to slight variations during the fabrication process, which resulted in slight changes in the degree of crosslinking. The effect of cyclic swelling and drying of γCD gels was also tested, and there was no significant change in swelling ratio of γCD gels after 5 cycles (Figure S1).
Figure 2. Physical characterization of crosslinked γCD hydrogel. (A) Macroscopic images of γCD gels show that the gel is transparent in both the dry and hydrated state. Significant swelling of the hydrogel is evident in the size change after hydration. (B) The SEM image of the interior of the freeze-dried γCD gel shows a consistent porous structure. C) The γ-CD gels were highly swellable with an average swelling ratio of 4.33 ± 0.63. Overlaid points represent individual replicates and are color coded by hydrogel batch
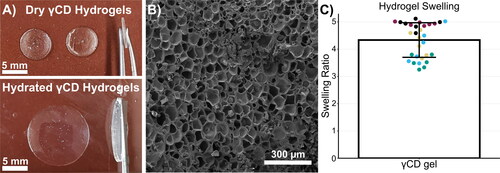
For comparison, hydrogels were made from crosslinked dextran, which is chemically identical to cyclodextrin, except it is composed of glucose monomers arranged in long branched, linear chains instead of rings and is thus not capable of forming inclusion complexes. Dextran hydrogels resulted in very soft, fragile gels with an average swelling ratio of 27.18 ± 2.83, mainly attributed to the higher molecular weight and longer chain length of the dextran compared to γCD.
To characterize the mechanical characteristics of the γCD gels, the compressive modulus of hydrated gels was measured (Figure S2). The average modulus of the γCD gels was measured to be 2.04 ± 1.03 kPa, making this a relatively soft material comparable to collagen and gelatin-based hydrogels (Luo et al., Citation2022). Compression testing of ocular samples places ocular tissue compressive moduli generally also in the kPa range (Worthington et al., Citation2014). While the low stiffness of the γCD gels may be well-suited for implantation into the similarly soft tissue of the eye, many types of materials, including metals, silicones and gelatin, have been investigated for ocular implantation suggesting that a range of mechanical properties are potentially compatible with this application (Qin et al., Citation2023).
Hydrogel materials are well suited to subconjunctival implantation because the swelling of the hydrogel can act to secure its position after implantation and the material’s flexibility allows the implant to conform to the shape or curvature of the surrounding tissue (Reitsamer et al., Citation2019). Several types of hydrogel products for implantation in the subconjuctival space for ocular pressure management, fibrosis prevention or drug delivery have been investigated (Chatzara et al., Citation2019; Helmers et al., Citation2022; Imai et al., Citation2015; Reitsamer et al., Citation2019; Zelefsky et al., Citation2008). In all cases, the hydrogel implants were well tolerated in in vivo or clinical trials.
3.3. Improved drug loading efficiency in γCD gels
To load the drug, dried gels were hydrated in a saturated solution of JM. For γCD gels, this resulted in an average of 436 ± 25 µg of total JM loaded into each hydrogel disk (53% of the available drug in the loading solution) (). The batch-to-batch variability was less pronounced in the total JM loading data than the hydrogel swelling data, suggesting that small changes in swelling do not have a significant effect on drug loading. This supports the idea that the drug loading ability stems from the material itself, and not just the amount of loading solution absorbed by the gel. The γCD gels had approximately twice the amount of total JM loaded into one dextran gel under the same conditions (222 ± 10 µg).
Figure 3. Josamycin loading improved in cyclodextrin hydrogels. (A) The total mass of JM loaded into one 9 mm γCD gel disk was significantly higher than the total loaded drug in one dextran gel disk. Overlaid points represent individual replicates and are color coded by hydrogel batch. (B) Periodic measurement of the JM concentration in the loading solution during drug loading shows a significant drop in JM concentration for the γCD, but not for the dextran gels. This drop in concentration indicates that JM is interacting with the γCDs in the gel, pulling the JM out of solution and explains the higher total loading. (C) To account for differences in swelling when comparing drug loading, the local concentration of JM was calculated by normalizing the total loaded drug to the final volume of the hydrated gel. This demonstrates that the dextran gel had a local concentration close to the aqueous solubility of JM (intrinsic solubility of JM indicated by dotted line), while the γCD gel had a final concentration 4 times higher. This additionally indicates that JM was forming complexes with the crosslinked γCD and therefore the gel can support JM concentrations much higher than the drug’s intrinsic solubility. * indicates a significant difference with p < 0.05.
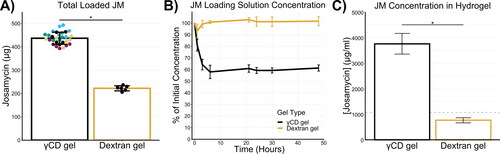
Further differences in drug loading behavior between γCD and dextran gels could be seen in the change in the loading solution concentration over the course of the drug loading procedure (). After only 1 hour of exposure of the γCD gel to the JM loading solution, the concentration of the solution dropped below 80% of the initial concentration and after 48 h the concentration was ∼60%, indicating an influx of drug into the gel. In contrast, the loading solution of the dextran gel samples did not deviate from the initial concentration during the experiment. The loading solutions of both groups were significantly different from the 3 h time point and onward. Additionally, shows that drug loading reaches equilibrium well before 24 hours and a longer loading time does not increase drug loading.
As a final evaluation of drug loading into the γCD gels, the concentration of JM within a hydrated gel was calculated (). This measurement normalized the total loaded amount of drug by the volume of the fully hydrated gels based on their known swelling properties. This value represents the local concentration of JM within the volume of the fully hydrated gel. Given no interactions between JM and the gel material, we would expect the final concentration of drug in the gel to be similar to the intrinsic solubility of JM (1070 µg/ml). The local concentration in the dextran gels (766 ± 97 µg/ml) supported this hypothesis as it was slightly less than the intrinsic solubility of JM. In contrast, the local concentration in the γCD gel showed a several fold increase over the solubility of JM in water with a local concentration of 3757 ± 405 µg/mL of JM within the gel. Increasing the local concentration of drug in our delivery system is particularly important for the intended application, because the subconjuctival space is limited. Maximizing drug delivery while minimizing volume may be important to meet surgical constraints and ensure patient comfort.
All the data from indicate that JM can still form complexes with γCD, even after EPI crosslinking. Dextran and γCD are chemically almost identical, with the same glucose monomer backbone, but a different 3D structure. Dextran is composed of branching chains, while cyclodextrins form rings, which we know complex with JM, improving solubility. Based on the improved JM loading in the hydrogel, we can assume that the hydrophobic regions within the cyclodextrin rings are still at least partially accessible and JM/cyclodextrin complexes are able to form inside the gels. That is why, during loading of cyclodextrin gels, the JM concentration in solution decreases as the hydrophobic JM preferentially forms complexes within the gel, which was not observed during the loading of dextran gels. This concentrating effect significantly improves drug loading and local concentration of JM and is similar to the improvement seen with other drugs in cyclodextrin-based hydrogels (Fu et al., Citation2011; Liu et al., Citation2021). Because the use of JM as an antifibrotic drug is still in the investigation stage, the ideal clinical dose is not known. If, in the future, higher drug loading is deemed necessary to achieve the desired therapeutic effect, the total loaded drug could potentially be increased by using solvents, solubilizers or excess drug to increase the concentration of JM in the loading solution.
The stability of JM in γCD gels was also investigated. Drug loaded γCD gels were dried completely, and stored at room temperature for up to six months, with no significant total loss of drug during that time (Figure S1). This result agrees with the aqueous solution stability data () and confirms that there is no degradation of JM due to long-term storage as part of a complex with γCD, which would allow for the development of a JM-loaded CD gel as a room temperature stable, off-the-shelf product.
3.4. Extended drug release from γCD gels
In vitro drug release from josamycin-loaded hydrogels was determined under sink conditions. The results are shown in as the cumulative percent of the total JM released () and the total amount of JM released at each time point (). The release data is also presented in Figure S3 with replicate values and showed no clear relationship between hydrogel batches and release results. γCD gels demonstrated a significantly extended JM release profile compared to the dextran gels. The cumulative percent released values were significantly lower compared to dextran from the 0.5 to 72 h release time points. At the 0.5 h time point, the amount released by the dextran gels was significantly higher than the γCD gels, but from 2 to 96 h, γCD gels release significantly more than the dextran gels, which only released JM for 6 hours. The γCD gels showed a sustained measurable release over the course of 4 days with trace amounts measured on day 7, at which point most measured concentrations were under the quantifiable limit (less than ∼2 µg total released at a given time point). We assume that the significant extension of the release profile in the γCD gels is due to the complex formation between JM and γCD. This affinity of γCD for JM is thought to reduce the burst release and generally slow the release rate of the drug compared to a release rate solely dependent on diffusion (Haley et al., Citation2020).
Figure 4. Prolonged drug release from γCD hydrogels. (A) Cumulative in vitro drug release of JM shown as a percent of the total released over the course of the experiment. γCD gels showed significantly prolonged release profiles. (B) The amount of JM released at each time point also clearly demonstrates a prolonged release from the γCD gel in comparison to dextran gels, which do not release a measureable amount of JM past 6 h. The inset graph shows a magnified view of the data from 0.5 to 6 h. * indicates time points where the groups are significantly different (p > 0.05)
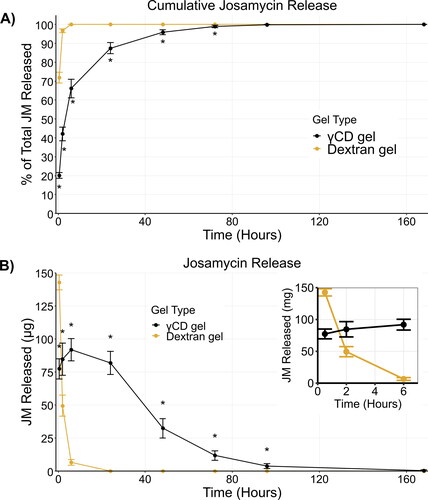
The release of inflammatory cytokines begins immediately after tissue injury during glaucoma filtration surgeries, leading to fibroblast recruitment to the site of injury in as little as 24 hours (Lama & Fechtner, Citation2003; Seibold et al., Citation2012). In the one to three days following surgery, secreted cytokines contribute to fibroblast proliferation and the transdifferentiation of fibroblasts into activated myofibroblasts. These myofibroblasts produce extracellular matrix components and are the main contributors to scarring and fibrosis (Bell et al., Citation2021; Schlunck et al., Citation2016; Seibold et al., Citation2012; Stahnke et al., Citation2017). Based on this timeline and previous work on other antifibrotic treatments after ocular surgery, we set the goal of developing a DDS that can release JM in the surgical area at the eye beginning rapidly after implantation and then continuously over several days to target the initial recruitment and activation of fibroblasts. Studies in rabbits demonstrate ocular delivery of JM via eye drops or subconjunctival injection leads to drug availability lasting only a few hours (Osono & Umezawa, Citation1971). Similarly, the in vitro release of JM from the tested dextran gels last only a few hours. However, detectable amounts of JM were released from γCD gel out to 7 days. While in vitro release data does not always directly correspond to in vivo release profiles, we hope to confirm in future studies that γCD gels show a similar or extended release profile in vivo. Additionally, due to the current investigational nature of JM as an antifibrotic drug, future in vivo testing will be needed to determine the ideal drug release profile needed to inhibit fibrosis. The γCD gel developed here has suitable characteristics for use in future in vivo studies to better understand the efficacy of continuous, prolonged release of JM as an antifibrotic treatment.
3.5. γCD Hydrogel biocompatibility
The biocompatibility of the γCD gels was tested by eluent medium tests following guidelines set out in ISO 10993-5. Eluent medium had no negative effect on the viability of the mouse fibroblast (L929) cells (), with the γCD gel eluent medium having a final relative viability of 112 ± 2% compared to normal medium. From this result, we can assume that the applied washing procedure was sufficient to remove any unreacted EPI crosslinker after the gelation process, as EPI is known to be toxic (Lawrence et al., Citation1972; Shin et al., Citation2010).
Figure 5. γCD Hydrogels demonstrated good biocompatibility. (A) L929 cells were cultured in eluent medium from γCD gels and showed no loss of viability compared to cells grown in normal medium. (B) γCD gels do not support direct cell attachment and L929 cells seeded directly on the gels remain rounded instead of spreading out when seeding on the surface of the γCD gel (left image). However, cells that were seeded on the plate next to the gels grew up to and under the gel, indicating that direct contact is tolerated (right image). The edge of the gel can be seen running vertically through the middle of the image.
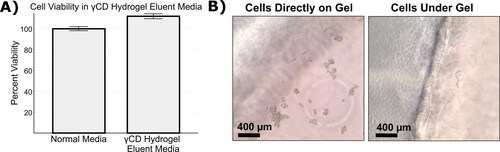
A typical direct contact toxicity assay could not be performed because cells cannot attach to the γCD gel surface. (left image) shows an image taken 48 h after direct seeding with the focal plane at the top surface of a γCD gel, the rounded shape of the cells indicates a lack of attachment to the gel surface. However, cells seeded beside the γCD gel grew normally and were able to grow up to and under the γCD gel, demonstrated in (right image). On the left hand side of that image, cells grew directly on the plate and appear densely packed with normal morphology. On the right hand side, individual cells can be seen which have migrated under the gel. This indicates that, while the γCD gels offer no points for direct cell attachment, direct physical contact is not cytotoxic.
3.6. Enzymatic degradation of γCD gels
The enzyme α-amylase is known to readily digest γCD, but not αCD or βCD (Marshall & Miwa, Citation1981; Loftsson & Stefánsson, Citation2017; Lumholdt et al., Citation2012). Implanted γCD gels are expected to come into contact with α-amylase at the eye in Tenon space because, while the enzyme is primarily produced in the pancreas and salivary glands, it is also produced in the lacrimal gland in the eye (Bodelier et al., Citation1993; van Haeringen et al., Citation1975). To test the potential for enzymatic degradation, γCD gels were incubated with α-amylase for 4 months, but showed no significant loss in mass compared to gels incubated without enzyme (Figure S4). γCD gels with and without exposure to the enzyme showed a ∼5% mass loss over the experimental period, which we hypothesize results from the loss of small pieces of the gel due to the mechanical forces applied by constant shaking and periodic washing. As there is no significant difference between the enzyme and control groups at the end of the experiment, it does not appear that the α-amylase enzyme is sufficient to degrade the γCD gels. It is unknown whether the lack of effect by the enzyme is due to interference between the enzyme and the active site on the γCD molecules due to the crosslinking by EPI, a short chain cross linker, or if the digestion of the crosslinked γCDs was simply not enough to lead to measurable mass loss during the experimental period. The lack of apparent degradation was unexpected, given γCD’s known susceptibility to α-amylase.
We had hypothesized that α-amylase would be able to degrade γCDs even after they were crosslinked into a hydrogel and that this degradation would be observed as a loss of total gel mass over time. However, even with high concentrations of enzyme and extended incubation times, we saw no evidence of degradation. This is a significant limitation to the γCD gels in their current form. For our intended application as a subconjunctivally implanted DDS, biodegradation is necessary. Ideally, the degradation would happen in the weeks to months after implantation, allowing for enough time for the drug to release and exert an antifibrotic effect, but without remaining indefinitely. The permanent presence of the implant could potentially lead to a foreign body reaction and thus increase fibrotic activity that could potentially hinder the function of the trabeculectomy.
Because γCD, but not αCD or βCD, can be degraded enzymatically, and γCD hydrogels are less commonly investigated in the literature than αCD or βCD-based gels, to the best of our knowledge the issue of enzymatic degradation of EPI crosslinked cyclodextrins has not been addressed previously. While the characteristics of the γCD gels generally met the requirements for our application, in this regard, improvements will need to be made. Future work will explore making the hydrogel degradable through the use of degradable crosslinkers or including biodegradable copolymers such as other biocompatible polysaccharides, which can also be crosslinked with EPI with minimal changes to the fabrication method (Liu et al., Citation2021). Additionally, temporary topical application of hydrogel could be explored which would eliminate the need for degradation as the hydrogel could be removed after the drug delivery period is complete.
4. Conclusion
JM has been identified as a possible new antifibrotic drug to prevent fibrosis after glaucoma filtration surgeries. To facilitate further investigation and application of JM as an antifibrotic, we developed a novel DDS designed to compensate for and work with the hydrophobic nature of JM. γCD was shown to significantly increase the solubility of JM in solution and γCD could be quickly and easily crosslinked with EPI, forming a highly swellable, biocompatible hydrogel. Confirming our hypothesis, the crosslinked γCD in the hydrogels was still able to complex with JM, resulting in a significantly increased drug loading efficiency. Additionally, the γCD hydrogels significantly prolonged the in vitro drug release profile. In vitro degradation testing showed that the crosslinking process appeared to interfere with enzymatic degradation. However, the γCD gel developed here demonstrated continuous, prolonged release of JM, which will facilitate the continued investigation of JM as an antifibrotic medication.
Authors’ contributions
J.H.: Conceptualization, Investigation, Writing - Original Draft, Visualization. S.O.: Conceptualization, Investigation, Writing - Review & Editing. H.L.: Conceptualization. K.S.: Investigation, Writing - Review & Editing. T.S.: Conceptualization Writing - Review & Editing. J.M.: Investigation, Formal analysis, Writing - Review & Editing. O.S.: Conceptualization. S.M.: Conceptualization. N.U.: Conceptualization, Writing - Review & Editing. A.P.: Conceptualization, Writing - Review & Editing. A.J: Conceptualization. N.G: Project administration, Conceptualization. G.F.: Project administration, Conceptualization, Writing - Review & Editing. T.E.: Supervision, Conceptualization, Writing - Review & Editing.
Supplemental Material
Download MS Word (477.8 KB)Acknowledgments
The expert technical assistance of Katja Hahn, Gabriele Karsten and Martina Nerger is gratefully acknowledged.
Disclosure statement
J.H., T.E, S.O., N.G., K.S., T.S., O.S., A.J., G.F. are listed as inventors on related pending patents submitted on behalf of the Rostock University Medical Center. The authors declare no other competing financial interests or personal relationships that could have appeared to influence the work reported in this paper.
Data availability statement
The data that support the findings of this study are available from the corresponding author, Jennifer Huling, upon request.
Additional information
Funding
References
- Antlsperger G, Schmid G. (1996). Toxicological comparison of cyclodextrins. In Proceedings of the eighth international symposium on cyclodextrins. Dordrecht: Springer, 149–55. doi: 10.1007/978-94-011-5448-2_32.
- Bell K, Padua Soares Bezerra B. d, Mofokeng M, et al. (2021). Learning from the past: mitomycin C use in trabeculectomy and its application in bleb-forming minimally invasive glaucoma surgery. Surv Ophthalmol 66:109–23. doi: 10.1016/j.survophthal.2020.05.005.
- Bodelier VMW, van Haeringen NJ, Klaver PSY. (1993). Species differences in tears; Comparative investigation in the chimpanzee (Pan troglodytes). Primates 34:77–84. doi: 10.1007/BF02381283.
- Chatzara A, Chronopoulou I, Theodossiadis G, et al. (2019). Xen implant for glaucoma treatment: a review of the literature. Semin Ophthalmol 34:93–7. doi: 10.1080/08820538.2019.1581820.
- Cheng T, Zhao Y, Li X, et al. (2007). Computation of octanol-water partition coefficients by guiding an additive model with knowledge. J Chem Inf Model 47:2140–8. doi: 10.1021/ci700257y.
- El Harti J, Cherrah Y, Bouklouze A. (2012). Improvement of water solubility of josamycin by inclusion complex with γ-cyclodextrin. ISRN Analyt Chem 2012:1–6. doi: 10.5402/2012/673564.
- Fang G, Wang Q, Yang X, et al. (2022). γ-Cyclodextrin-based polypseudorotaxane hydrogels for ophthalmic delivery of flurbiprofen to treat anterior uveitis. Carbohydr Polym 277:118889. doi: 10.1016/j.carbpol.2021.118889.
- Fu AS, Thatiparti TR, Saidel GM, et al. (2011). Experimental studies and modeling of drug release from a tunable affinity-based drug delivery platform. Ann Biomed Eng 39:2466–75. doi: 10.1007/s10439-011-0336-z.
- Gudmundsdottir BS, Petursdottir D, Asgrimsdottir GM, et al. (2014). γ-Cyclodextrin nanoparticle eye drops with dorzolamide: Effect on intraocular pressure in man. J Ocul Pharmacol Ther 30:35–41. doi: 10.1089/jop.2013.0060.
- Haley RM, Zuckerman ST, Dakhlallah H, et al. (2020). Resveratrol delivery from implanted cyclodextrin polymers provides sustained antioxidant effect on implanted neural probes. Int J Mol Sci 21:3579. doi: 10.3390/ijms21103579.
- Helmers G, Munteanu C, Löw U, Seitz B. (2022). Trabeculectomy with mitomycin C and Ologen® implant in comparison to classical trabeculectomy. Ophthalmologie 120:20–6. doi: 10.1007/s00347-022-01686-4.
- Imai H, Misra GP, Wu L, et al. (2015). Subconjunctivally implanted hydrogels for sustained insulin release to reduce retinal cell apoptosis in diabetic rats. Invest Ophthalmol Vis Sci 56:7839–46. doi: 10.1167/iovs.15-16998.
- International Organization for Standardization. (2009). ISO 10993-5 biological evaluation of medical devices: Part 5: Tests for in vitro cytotoxicity. Geneva.
- International Organization for Standardization. (2021). ISO 10993-12 biological evaluation of medical devices: Part 5: Sample preparation and reference materials. Geneva.
- Jumelle C, Gholizadeh S, Annabi N, Dana R. (2020). Advances and limitations of drug delivery systems formulated as eye drops. J Control Release 321:1–22. doi: 10.1016/j.jconrel.2020.01.057.
- Kapetanakis VV, Chan MPY, Foster PJ, et al. (2016). Global variations and time trends in the prevalence of primary open angle glaucoma (POAG): A systematic review and meta-analysis. Br J Ophthalmol 100:86–93. doi: 10.1136/bjophthalmol-2015-307223.
- Kopp F, Eickner T, Polei S, et al. (2017). Ultrahigh field MR imaging of a subconjunctival anti-glaucoma drug delivery system in a rabbit model. Sci Rep 7:15780. doi: 10.1038/s41598-017-15954-w.
- Lama PJ, Fechtner RD. (2003). Antifibrotics and wound healing in glaucoma surgery. Surv Ophthalmol 48:314–46. doi: 10.1016/S0039-6257(03)00038-9.
- Lawrence WH, Malik M, Turner JE, Autian J. (1972). Toxicity profile of epichlorohydrin. J Pharm Sci 61:1712–6. doi: 10.1002/jps.2600611103.
- Leroy-Lechat F, Wouessidjewe D, Andreux J, et al. (1994). Evaluation of the cytotoxicity of cyclodextrins and hydroxypropylated derivatives. Int J Pharm 101:97–103. doi: 10.1016/0378-5173(94)90080-9.
- Liu J, Tian B, Liu Y, Wan J, ‑B. (2021). Cyclodextrin-containing hydrogels: a review of preparation method, drug delivery, and degradation behavior. Int J Mol Sci 22:13516. doi: 10.3390/ijms222413516.
- Loftsson T, Hreinsdóttir D, Másson M. (2005). Evaluation of cyclodextrin solubilization of drugs. Int J Pharm 302:18–28. doi: 10.1016/j.ijpharm.2005.05.042.
- Loftsson T, Stefánsson E. (2017). Cyclodextrins and topical drug delivery to the anterior and posterior segments of the eye. Int J Pharm 531:413–23. doi: 10.1016/j.ijpharm.2017.04.010.
- Lumholdt LR, Holm R, Jørgensen EB, Larsen KL. (2012). In vitro investigations of α-amylase mediated hydrolysis of cyclodextrins in the presence of ibuprofen, flurbiprofen, or benzoapyrene. Carbohydr Res 362:56–61. doi: 10.1016/j.carres.2012.09.018.
- Luo T, Tan B, Zhu L, et al. (2022). A review on the design of hydrogels with different stiffness and their effects on tissue repair. Front Bioeng Biotechnol 10:817391. doi: 10.3389/fbioe.2022.817391.
- Machín R, Isasi JR, Vélaz I. (2013). Hydrogel matrices containing single and mixed natural cyclodextrins. Mechanisms of drug release. Eur Polym J 49:3912–20. doi: 10.1016/j.eurpolymj.2013.08.020.
- Mallone F, Costi R, Marenco M, et al. (2021). Understanding drivers of ocular fibrosis: current and future therapeutic perspectives. Int J Mol Sci 22:11748. doi: 10.3390/ijms222111748.
- Marshall JJ, Miwa I. (1981). Kinetic difference between hydrolyses of γ-cyclodextrin by human salivary and pancreatic α-amylases. Biochim Biophys Acta 661:142–7. doi: 10.1016/0005-2744(81)90093-0.
- Mennini N, Casella G, Cirri M, et al. (2016). Development of cyclodextrin hydrogels for vaginal delivery of dehydroepiandrosterone. J Pharm Pharmacol 68:762–71. doi: 10.1111/jphp.12549.
- Osono T, Umezawa H. (1971). Josamycin, a new macrolide antibiotic of resistance non-inducing type. In: Mitsuhashi S, ed. Drug action and drug resistance in bacteria. Tokyo, Japan: University of Tokyo Press. 41–115.
- Qin Q, Zhang Chengshou Yu Naiji Jia F, Liu Xin Zhang Q, et al. (2023). Development and material characteristics of glaucoma surgical implants. In Adv Ophthalmol Pract Res 3:171–9. doi: 10.1016/j.aopr.2023.09.001.
- R Core Team. (2022). R: A language and environment for statistical computing [Computer software]. R Foundation for Statistical Computing, Vienna, Austria. Available at: https://www.R-project.org/.
- Rafiei F, Tabesh H, Farzad F. (2020). Sustained subconjunctival drug delivery systems: Current trends and future perspectives. Int Ophthalmol 40:2385–401. doi: 10.1007/s10792-020-01391-8.
- Reibaldi A, Uva MG, Longo A. (2008). Nine-year follow-up of trabeculectomy with or without low-dosage mitomycin-c in primary open-angle glaucoma. Br J Ophthalmol 92:1666–70. doi: 10.1136/bjo.2008.140939.
- Reitsamer H, Sng C, Vera V, et al. (2019). Two-year results of a multicenter study of the ab interno gelatin implant in medically uncontrolled primary open-angle glaucoma. Graefe’s Archive for Clinical and Experimental Ophthalmology 257:983–96. doi: 10.1007/s00417-019-04251-z.
- Renard E, Deratani A, Volet G, Sebille B. (1997). Preparation and characterization of water soluble high molecular weight β-cyclodextrin-epichlorohydrin polymers. Eur Polym J 33:49–57. doi: 10.1016/S0014-3057(96)00123-1.
- Rohner NA, Nguyen D, & Recum HA. von (2020). Affinity effects on the release of non-conventional antifibrotics from polymer depots. Pharmaceutics 12:275. doi: 10.3390/pharmaceutics12030275.
- RStudio Team. (2020). RStudio: Integrated development for R [computer software]. Boston, MA: RStudio, PBC. Available at: http://www.rstudio.com/.
- S Khouri A, Huang G, Y Huang L. (2017). Intraoperative injection vs sponge-applied mitomycin c during trabeculectomy: one-year study. J Curr Glaucoma Pract 11:101–6. doi: 10.5005/jp-journals-10028-1233.
- Saarinen-Savolainen P, Järvinen T, Araki-Sasaki K, et al. (1998). Evaluation of cytotoxicity of various ophthalmic drugs, eye drop excipients and cyclodextrins in an immortalized human corneal epithelial cell line. Pharm Res 15:1275–80. doi: 10.1023/a:1011956327987.
- Saokham P, Loftsson T. (2017). γ-Cyclodextrin. Int J Pharm 516:278–92. doi: 10.1016/j.ijpharm.2016.10.062.
- Schlunck G, Meyer-ter-Vehn T, Klink T, Grehn F. (2016). Conjunctival fibrosis following filtering glaucoma surgery. Exp Eye Res 142:76–82. doi: 10.1016/j.exer.2015.03.021.
- Schwartz GF, Quigley HA. (2008). Adherence and persistence with glaucoma therapy. Surv Ophthalmol 53 Suppl1:S57–S68. doi: 10.1016/j.survophthal.2008.08.002.
- Seibold LK, Sherwood MB, Kahook MY. (2012). Wound modulation after filtration surgery. Surv Ophthalmol 57:530–50. doi: 10.1016/j.survophthal.2012.01.008.
- Shin I-S, Park N-H, Lee J-C, et al. (2010). One-generation reproductive toxicity study of epichlorohydrin in Sprague-Dawley rats. In. Drug Chem Toxicol 33:291–301. doi: 10.3109/01480541003734030.
- Sripetch S, Jansook P, Loftsson T. (2020). Effect of porcine pancreatic α-amylase on dexamethasone release from aqueous solution containing natural γ-cyclodextrin. Int J Pharm 585:119452. doi: 10.1016/j.ijpharm.2020.119452.
- Stahnke T, Gajda-Deryło B, Jünemann AG, et al. (2020). Suppression of the TGF-β pathway by a macrolide antibiotic decreases fibrotic responses by ocular fibroblasts in vitro. R Soc Open Sci 7:200441. doi: 10.1098/rsos.200441.
- Stahnke T, Kowtharapu BS, Stachs O, et al. (2017). Suppression of TGF-β pathway by pirfenidone decreases extracellular matrix deposition in ocular fibroblasts in vitro. Plos ONE 12:e0172592. doi: 10.1371/journal.pone.0172592.
- Stahnke T, Löbler M, Kastner C, et al. (2012). Different fibroblast subpopulations of the eye: A therapeutic target to prevent postoperative fibrosis in glaucoma therapy. Exp Eye Res 100:88–97. doi: 10.1016/j.exer.2012.04.015.
- van Haeringen NJ, Ensink F, Glasius E. (1975). Amylase in human tear fluid: Origin and characteristics, compared with salivary and urinary amylases. Exp Eye Res 21:395–403. doi: 10.1016/0014-4835(75)90049-4.
- Vinod K, Gedde SJ, Feuer WJ, et al. (2017). Practice preferences for glaucoma surgery: a survey of the american glaucoma society. J Glaucoma 26:687–93. doi: 10.1097/IJG.0000000000000720.
- Wintgens V, Amiel C. (2010). Water-soluble γ-cyclodextrin polymers with high molecular weight and their complex forming properties. Eur Polym J 46:1915–22. doi: 10.1016/j.eurpolymj.2010.06.014.
- Wolters JEJ, van Mechelen RJS, Al Majidi R, et al. (2021). History, presence, and future of mitomycin C in glaucoma filtration surgery. Curr Opin Ophthalmol 32:148–59. doi: 10.1097/ICU.0000000000000729.
- Worthington KS, Wiley LA, Bartlett AM, et al. (2014). Mechanical properties of murine and porcine ocular tissues in compression. Exp Eye Res 121:194–9. doi: 10.1016/j.exer.2014.02.020.
- Yang SY, Hoonor R, Jin H-S, Kim J. (2013). Synthesis and characterization of cationic and anionic cyclodextrin oligomers and their use in layer-by-layer film formation. Bull Korean Chem Soc 34:2016–22. doi: 10.5012/bkcs.2013.34.7.2016.
- Yoon PS, Singh K. (2004). Update on antifibrotic use in glaucoma surgery, including use in trabeculectomy and glaucoma drainage implants and combined cataract and glaucoma surgery. Curr Opin Ophthalmol 15:141–6. doi: 10.1097/00055735-200404000-00015.
- Zelefsky JR, Hsu W, ‑C, Ritch R. (2008). Biodegradable collagen matrix implant for trabeculectomy. Expert Rev Ophthalmol 3:613–7. doi: 10.1586/17469899.3.6.613.