Abstract
Context/Objective: Spinal cord injury (SCI) causes atrophy of brain regions linked to motor function. We aimed to estimate cortical thickness in brain regions that control surgically restored limb movement in individuals with tetraplegia.
Design: Cross-sectional study.
Setting: Sahlgrenska University hospital, Gothenburg, Sweden.
Participants: Six individuals with tetraplegia who had undergone surgical restoration of grip function by surgical transfer of one elbow flexor (brachioradialis), to the paralyzed thumb flexor (flexor pollicis longus). All subjects were males, with a SCI at the C6 or C7 level, and a mean age of 40 years (range = 31–48). The average number of years elapsed since the SCI was 13 (range = 6–26).
Outcome measures: We used structural magnetic resonance imaging (MRI) to estimate the thickness of selected motor cortices and compared these measurements to those of six matched control subjects. The pinch grip control area was defined in a previous functional MRI study.
Results: Compared to controls, the cortical thickness in the functionally defined pinch grip control area was not significantly reduced (P = 0.591), and thickness showed a non-significant but positive correlation with years since surgery in the individuals with tetraplegia. In contrast, the anatomically defined primary motor cortex as a whole exhibited substantial atrophy (P = 0.013), with a weak negative correlation with years since surgery.
Conclusion: Individuals with tetraplegia do not seem to have reduced cortical thickness in brain regions involved in control of surgically restored limb movement. However, the studied sample is very small and further studies with larger samples are required to establish these findings.
Introduction
Spinal cord injury (SCI) may lead to persistent functional deficits due to the limited repair of severed axonal connections in the central nervous system (CNS).Citation1 Neuroimaging studies suggest that spinal cord injury (SCI) may cause significant anatomical alterations in cerebral cortical structures controlling motor output, and subsequent functional reorganization.Citation2–5 Long-term disruption of motor efferents and sensory afferents that occurs after SCI may result in permanent atrophic changes.Citation2,Citation6 However, in a recent systematic review it is concluded that previous structural neuroimaging studies exploring SCI-related anatomical changes have demonstrated various and partly divergent findings.Citation5 Several studies have identified primary sensorimotor cortex cortical atrophy following SCICitation2,Citation3,Citation7,Citation8 including the denervated leg area of the primary sensorimotor cortex, although others have found atrophy only in somatosensory (S1) cortices but not primary motor cortex (M1).Citation9,Citation10 From a clinical perspective, a notable association exists between increased changes in the brain and spinal cord and poor recovery, whereas decreased rates of atrophy are shown to be associated with improved clinical outcome.Citation7
Most of the motor recovery in upper extremities occurs within the first 6 months and especially within 3 months post-injury,Citation11 but modest improvement can continue thereafter.Citation3,Citation12 For most individuals the functional losses persist long after the spinal cord trauma, usually throughout life.Citation7 Given the challenges in achieving regeneration of the injured spinal cord, a significant amount of research has been devoted to exploring neuroprotective and neuroregenerative approaches.Citation13,Citation14 The vast majority of treatment approaches are applicable at the acute and subacute stages of SCI. For individuals with more severe SCI having limited potential for neurorecovery, rehabilitation approaches focus on utilizing compensatory or assisting techniques to optimize function.Citation15 The selection of restorative and/or compensatory techniques is affected by the severity of SCI.Citation15
Current neuro-rehabilitation strategies take advantage of a fundamental feature of neural circuits, which is the capacity for adaptations in the CNS in response to therapeutic interventions.Citation16,Citation17 CNS’s ability to reorganize and adapt to environmental stimuli or internal changes and thereby optimize functional outcome is termed neuroplasticity.Citation18 The disrupted neural circuity resulting from a SCI constitutes a barrier to plasticity driven restoration of motor function in individuals with SCI.Citation19 A reliable and powerful tool to reverse paralysis after spinal cord injury, independent of the disrupted neural circuity and the time elapsed since injury is reconstructive limb surgery.Citation20 Surgical techniques have been established to restore upper extremity function for tetraplegics. In surgical restoration by tendon transfer, a functioning muscle is moved from one part of the limb to where it is more useful, creating better voluntary control of the arm and hand.Citation20 Previous studies have demonstrated that surgical restoration of upper limb function leads to satisfactory gains in activities of daily living as well as enhanced quality of life.Citation21,Citation22 The basis for surgical restoration of the tetraplegic hand lies in the active mobilization of the paralyzed joint motors.Citation23 Tendon transfers represent the core of traditional procedures in which the distal end of a functionally intact muscle and its tendon are detached from its normal insertion, rerouted and reattached to a paralyzed muscle to replace its original function. Innovative single-stage combined procedures have derived from basic scientific research and clinical studies, and have been proven to offer considerable advantages over traditional approaches.Citation20 An advanced type of grip reconstruction including a combination of seven surgical procedures has previously been developed, providing simultaneous active key pinch and global finger grasp together with passive hand opening.Citation24 Reconstruction of thumb flexion to create key pinch is preferably achieved by transferring one of the three elbow flexor muscles, the tendon of Brachioradialis (BR) to the tendon of the paralyzed thumb flexor; Flexor Pollicis Longus (FPL). Active finger flexion is most commonly restored by transfer of Extensor Carpi Radialis Longus (ECRL) to synergistic Flexor Digitorum Profundus (FDP).Citation20
To optimize recruitment of the restored function after surgery, the rehabilitation program includes early motor reeducation.Citation25 The training regimen has previously been described in detail.Citation26 Recovery of functional pinch depends on how well the patient learns to activate the BR during the pinch task through its new distal attachment, and also to control flexion at the elbow through its proximal attachment.Citation27 In order to achieve an efficient, accurate and smooth functional movement, patients must learn to coordinate the action of the pertinent muscle groups. A strong contraction of the BR in pinch requires synchronized activation of the antagonistic Triceps muscle since the transferred BR continues to produce an elbow flexor moment due to its proximal attachment on the humerus.Citation28
Surgical procedures on the hand is accompanied by organizational changes in the brain, and the outcome of many hand surgical procedures is to a large extent dependent on brain plasticity.Citation29
Integration of the motor output takes place in both M1 cortical circuits within the human brain,Citation30 as well as within the spine.Citation31 It has been suggested that neuroplastic changes within M1 may underlie the learning of novel synergic movements.Citation31 We recently demonstrated that surgical reconstruction of thumb flexion to create key pinch in individuals with tetraplegia is most likely associated with plastic changes of the motor cortex that allow for regained motor control.Citation32 Our findings suggest a neuroplastic mechanism in which motor cortex resources previously dedicated to elbow flexion adapt to control also the thumb. Given that a synchronous activation of BR and triceps is crucial for the capability to isolate the pinch grip, and that the BR retains its function as an elbow actuator also after transfer, we postulated that the previously defined pinch grip control area would not exhibit atrophy (relative to neighboring regions of M1). Demonstration of such alterations in cortical thickness in individuals with tetraplegia who have undergone successful pinch grip restoration would be interesting for two reasons. First, it would complement the previous findings pointing to a plastic mechanism in which the cortical region for elbow flexion may have adapted to control also the paralyzed thumb muscle.Citation32 Second, it could provide an objective neuroimaging marker of learned synchrony of muscle contractions in surgically restored movement. We therefore used magnetic resonance imaging (MRI) to estimate the thickness of selected motor cortices (M1) and compared these measurements to those of matched control subject. The same individuals were examined in our previous functional MRI (fMRI) study of functional plasticity associated with surgery.Citation32 The previous fMRI results allowed us to pinpoint the individuals’ brain regions, which control the surgically restored limb, hereafter defined as the “functionally defined pinch grip control area”.
Materials and methods
Participants
Six right-handed males with tetraplegia with a mean age of 40 (range = 31–48) who underwent right side upper-limb grip reconstructive surgery at Sahlgrenska University hospital, Gothenburg, Sweden between the years 2005 and 2014 participated in the study. The same individuals were included in a previous fMRI study.Citation32 Prior to the surgical grip reconstruction two individuals underwent reconstruction of the elbow extensor (triceps) by a Posterior Deltoid-to-Triceps transfer. Two individuals had undergone grip reconstruction on both hands. The postoperative therapy protocol after surgical grip reconstruction is previously presented in detail.Citation26 Six sex and age matched control subjects was recruited, all right-handed with a mean age of 39 years (range = 29–46). Eligibility criteria were: 1) The surgical intervention must have been performed at least one year prior to inclusion and include restoration of thumb flexion with the goal of reconstructing an active key pinch by a BR to FPL tendon transfer; 2) No motor function below the wrist such as finger- and/or thumb extensors; 3) Complete or incomplete SCIs with an injury level C4–C7 with intact BR control; 4) No history of a medical or other neurological disease that might affect the investigated parameters; 5) No defective vision that require the use of glasses in the MRI assessment; 6) Individual factors that precludes entering the MRI environment (e.g. metal implants which are not compatible with the MRI environment, pacemaker, claustrophobia); 7) Able to speak and understand Swedish; and 8) ability to travel to Gothenburg. Prior to inclusion, all participants did receive oral and written information about the study procedures. Informed consent from all study participants were obtained as well as approval from the Regional Ethics Committee of Research Involving Humans in Gothenburg, Sweden (Dnr: 309-16).
MRI acquisition and functional pinch grip localization
A Philips Gyroscan 3 T Achieva was used to acquire structural T1-weighted scans (flip angle 9°, echo time 3.285 ms, repetition time 7.200 ms, 160 sagittal slices with scan resolution 1.0×1.0×1.0 mm3). After acquisition of structural scans, functional MRI (fMRI) scans were acquired, and the analysis and results are described previously.Citation32 In short, participants performed an isometric pinch grip task. Each scanning session in the fMRI protocol consisted of six runs, each including 10 pseudo-randomized 10s blocks and the movements were repeated a total of 300 times. Coordinates for the activation centers of gravity (CoGs) were then computed in each individual participant.Citation33 The process for converting these CoGs are described in MRI preprocessing and analysis below. Handedness was measured with “The assessment and analysis of handedness: the Edinburgh inventory”.Citation34
MRI preprocessing and analysis
Cortical thickness processing and analysis was performed using the Freesurfer image analysis suite, which is documented and freely available for download online (http://surfer.nmr.mgh.harvard.edu/). We examined average cortical thickness measures obtained from individual level surface reconstructions for two types of regions of interest (ROIs). First, we examined the anatomically localized primary motor cortex in the FreeSurfer Brodmann Atlas, i.e. Brodmann Area 4 (posterior and anterior portions combined). Since all participants were right-handed, we restricted the analyses to the left hemisphere. Second, we examined ROIs derived from the functionally defined motor cortex area that had regained control of the pinch grip, as described above.Citation32 Here, we used the reported individual coordinates of the primary motor cortex CoGs. ROIs were created as volumetric spheres with a 5 mm radius centered on these coordinates. The spherical ROIs were then converted into each participants’ native cortical surface space ROIs using FreeSurfer, and the average cortical thickness in each ROIs was extracted.
Group comparisons and correlation analyses
Statistical assessments were made using MATLAB with the Statistics Toolbox (Release 2017b; The MathWorks, Inc., Natick, Massachusetts, United States). We first assessed group differences in cortical thickness under the hypothesis that the individuals with tetraplegia had less gray matter than controls using one-tailed non-parametric Wilcoxon rank sum tests. We also examined correlations between gray matter thickness in this ROI and years since surgery in the individuals with tetraplegia. Since age may affect gray matter volumes, we computed a partial correlation while controlling for age.
Results
Participant demographics
Demographics, clinical characteristics and a summary of all surgical procedures among the individuals with tetraplegia is presented in . The average number of years elapsed since surgery was 7 (1–10).
Table 1 Demographics, clinical characteristics and surgical procedures included among the tetraplegic individuals.
Cortical thickness
We found that cortical thickness was not reduced in the individuals with tetraplegia compared to controls in the functionally defined pinch grip control area (P = 0.591) ( and A). Excluding the two individuals with bilateral surgery had minor effects on the results (P = 0.619). Cortical thickness correlated positively but not significantly with years since surgery, corrected for age (r = 0.19, P = 0.381) (A).
Figure 1 Gray matter thickness in (A) functionally and (B) anatomically defined areas of the primary motor cortex. Bar charts show cortical thickness in patients and control participants. The scatter plot shows patients’ cortical thickness as a function of time since reconstructive surgery, while controlling for age. Error bars indicate standard deviations, and the dotted lines show the 95% confidence bounds. Abbreviations: n.s., not significant; SCI, spinal cord injury.
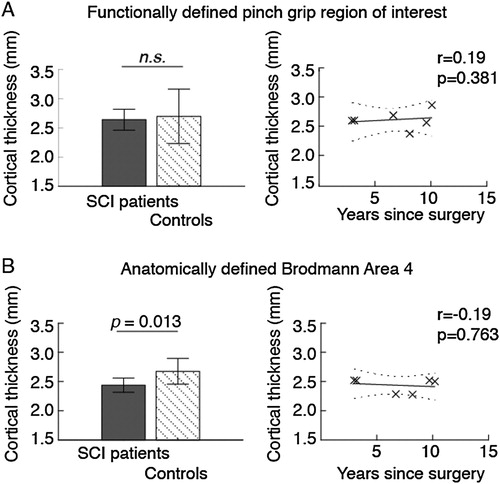
Table 2 Cortical thickness in individuals with tetraplegia and control participants.
Anatomically defined left hemisphere motor cortex thickness was significantly smaller in the individuals with tetraplegia compared to control participants (P = 0.013) ( and B). The group differences remained significant when the two individuals with tetraplegia who had bilateral surgery were excluded (P = 0.019). The correlation analysis found that cortical thickness decreased with time since surgery, but the association was not significant (r = −0.19, P = 0.763) (B). For individual cortical thicknesses, see .
Table 3 Individual cortical thicknesses for individuals with tetraplegia and control participants.
Discussion
We used structural MRI and surface analysis to make regional estimates of cortical thickness in individuals with tetraplegia with successful surgical key pinch grip restoration. We found that cortical thickness in the functionally defined pinch grip area of the motor cortex did not exhibit atrophy as compared to control subjects, whereas the anatomically defined primary motor cortex as a whole exhibited substantial atrophy, with a weak negative correlation with years since surgery. In contrast, cortical thickness in the functionally defined pinch grip area showed a non-significant positive correlation with years since surgery. Since the number of study subjects is small, these results primarily serve as a promising basis for further investigations into potential effects of reconstructive surgery on gray matter alterations in individuals with spinal cord injury.
The human hand represents one of the most complex biological motor systems, and how the brain controls motor actions remains an area of intense interest.Citation35 Even though the restoration of volitional thumb control after tendon transfer is primarily attributable to the direct effects of surgery, the process of re-learning and the establishment of novel synchronized motor pattern is complex and most likely aided by neuroplasticity mechanisms.Citation36–38 It is however unclear how motor programs and synergies are integrated and adapted at the onset of skill learning and where in the brain such operations are expressed.Citation39 An ever-increasing number of brain-imaging studies show that the basal ganglia and the cerebellum are incorporated into the distributed neural circuits subserving movement.Citation40,Citation41
The somatotopic map of M1 was selected as the region of interest in the present study since it is one of the principal brain areas involved in motor function.Citation31 Research on morphologic brain changes as a result of skill acquisition has revealed increases in regional estimates of human brain volume and cortical thickness in task-relevant areas.Citation42 Following a certain period of task-related interventions, increases in gray matter volume or thickness has been demonstrated.Citation43–45 A previous study used fMRI to investigate the mechanisms of learning a novel synergic movement in M1. Healthy study participants were to train the abductor pollicis brevis (APB) and the deltoid muscles for fast synchronous co-contraction. The result indicated that the learned synchrony of muscle contractions was related to rapid increase in functional connectivity between the central M1 representations of the participating muscle groups.Citation31
Accurate movement planning is dependent on proprioceptive and visual input.Citation46 Surgical transfer of the BR to the flexor of a paralyzed thumb induces peripheral feedback that most likely triggers adaptive plasticity mechanisms in the CNS.Citation47,Citation48 Moreover, the brain must adapt to the changed biomechanics,Citation49 as well as changes in muscle architecture and functionality in the extremity.Citation50,Citation51 The neuroplasticity of the brain that allows new learning, adaptation, and compensation at multiple levels of the systemCitation17 is thought to facilitate the process in which patients learn how to activate the BR in a voluntary pinch, through its new distal attachment. As a result, brain activity in cortical areas adjacent to the motor representation of the elbow may constitute a neural signatureCitation52 of the establishment of new representational motor maps in surgically restored key pinch. There are reasons to believe that successful motor relearning after tetraplegia surgery is dependent on establishment of these new representational maps, as indicated in the functional imaging results of our previous study.Citation32 Even though we have come a step closer to understanding the neural circuitry that coordinates re-established motor control after surgical restoration of a key pinch in tetraplegia, the intrinsic structural and molecular mechanisms that mediate the functional recovery could only be surmised at this stage. Most likely, there is a highly distributed network rather than functionally and spatially discrete groups of neurons controlling the movement.Citation35,Citation40,Citation41 This complex organization may be the substrate for functional plasticity in motor cortex, at least within each local subregion.Citation53
We found no significant correlations between gray matter thickness and time elapsed since surgery. However, the correlation between thickness and time since surgery was weakly negative in the anatomically defined motor cortex, and weakly positive in the functionally defined key pinch area. In our previous fMRI study, participants’ cortical thumb flexion representation were not topographically distinct from their elbow activations.Citation32 This findings led us to reason that regained thumb control may be the result of a functional remapping of elbow neurons corresponding to the transferred BR muscle area.Citation32 Since the participants had the spinal cord injured at the C6 or C7 level, proximal limb movements were preserved and the muscles acting as elbow flexion remained intact. This may also have contributed to the findings in the present study, indicating that the relative preservation of neural pathways from cortical structures in the elbow region to the periphery, as well as preserved afferent inputCitation48 may be partly responsible for the relative preservation of gray matter. In contrast, cortical regions responsible for voluntary motor control below the level of injury where a disrupted neural pathway occurs and the commands to move no longer reaches the muscles, exhibit a substantial artrophy. Secondly, is has to be born in mind that the BR is a dual-function muscle (elbow flexion and forearm rotation) that retains its function as an elbow actuator also after transfer.Citation28,Citation54
While this study gives indications of the preservation of cortical thickness in regions responsible for volitional control of restored limb function there are several limitations that must be acknowledged, among which the limited number of study participants is the primary one. A larger sample size and a more homogeneous group of participants is needed to investigate whether reconstructive limb surgery may counteract continuous gray matter loss of the motor cortex. Multiple factors complicate the recruitment of individuals with tetraplegia to an MRI investigation as the present one. First, the limited population of tetraplegic individuals who undergoes grip reconstruction annually and the geographical spread of individuals, made recruitment difficult. Second, the combination of surgical procedure as well as demographics and clinical characteristics should be as similar as possible among the participants, which further hampered the recruitment. On average, around 10–12 individuals undergo some kind of grip reconstruction every year at our specialized center. The eligibility criteria specified that the surgical intervention needed to include restoration of thumb flexion with the goal of reconstructing an active key pinch and that no remaining motor function distal to the wrist was allowed. In case a patient has a limited amount of donor muscles available for transfer one usually choose to reconstruct a passive key pinch instead by strengthening wrist extension by transfer of BR to extensor carpi radialis brevis (ECRB) combined with a tenodesis of the FPL tendon to the distal radius. Individuals who had undergone this latter procedure were thus excluded, as were individuals with remaining motor function below the wrist, such as finger- and/or thumb extensors. These clinical requirements further diminished the number of eligible individuals. Since individuals with tetraplegia with defective vision often prefer using glasses instead of contact lenses due to their impaired hand function, and that the use of glasses in the MRI scanner was not allowed, this criterion caused further exclusion of individuals. Hence, given these prerequisites, the number of included participants in the present investigation is considered fairly acceptable. Another important limitation is that the pinch-grip area in the motor cortex as defined by MRI may vary slightly in individuals and the cross-sectional design of the study that did not allow for pre-operative MRI scans to be taken. Such scans would have further validated the results.
Despite these limitations, the current results, coupled with our previous findings,Citation32 suggests that the restoration of upper limb function by means of tendon transfer after SCI may favor the preservation of the functional and structural properties of regional motor cortices. Even though the possible link between tendon transfer and the preservation of motor cortices responsible for the volitional control of restored movement is highly speculative, these findings may have a translational value in understanding the plasticity mechanisms that enable new motor patterns to emerge after surgery leading to successful pinch grip restoration. The current results may also give indications of possible avenues for future research.
Conclusion
Findings from the current study indicate that individuals with tetraplegia do not seem to have reduced gray matter thickness in brain regions involved in volitional control of surgically restored limb movement. A link between structural plasticity and motor re-establishment after tendon transfer remains however to be demonstrated. These results may provide testable hypotheses for future investigations aiming to explore the plasticity mechanisms mediating the restoration of volitional upper limb movement after tendon transfer.
Disclaimer statements
Contributors All authors contributed to conception and design of the study. LBK performed the data collection and MB performed the statistical analyses. All authors interpreted the data. LB and MB drafted the manuscript. All authors critically revised the manuscript and gave final approval of the version to be published.
Declaration of interest None.
Conflicts of interest Authors have no conflict of interests to declare.
Acknowledgements
The authors gratefully acknowledge the participants for making this study possible.
Additional information
Funding
References
- Raineteau O, Schwab ME. Plasticity of motor systems after incomplete spinal cord injury. Nat Rev Neurosci 2001;2(4):263–73. doi: 10.1038/35067570
- Wrigley PJ, Gustin SM, Macey PM, Nash PG, Gandevia SC, Macefield VG, et al. Anatomical changes in human motor cortex and motor pathways following complete thoracic spinal cord injury. Cereb Cortex 2009;19(1):224–32. doi: 10.1093/cercor/bhn072
- Ziegler G, Grabher P, Thompson A, Altmann D, Hupp M, Ashburner J, et al. Progressive neurodegeneration following spinal cord injury: implications for clinical trials. Neurology 2018;90(14):e1257–66. doi: 10.1212/WNL.0000000000005258
- Freund P, Curt A, Friston K, Thompson A. Tracking changes following spinal cord injury: insights from neuroimaging. Neuroscientist 2013;19(2):116–28. doi: 10.1177/1073858412449192
- Nardone R, Höller Y, Sebastianelli L, Versace V, Saltuari L, Brigo F, et al. Cortical morphometric changes after spinal cord injury. Brain Res Bull 2018;137:107–19. doi: 10.1016/j.brainresbull.2017.11.013
- Chen Q, Zheng W, Chen X, Wan L, Qin W, Qi Z, et al. Brain gray matter atrophy after spinal cord injury: a voxel-based morphometry study. Front Hum Neurosci 2017;11:211. doi: 10.3389/fnhum.2017.00211
- Freund P, Weiskopf N, Ashburner J, Wolf K, Sutter R, Altmann DR, et al. MRI investigation of the sensorimotor cortex and the corticospinal tract after acute spinal cord injury: a prospective longitudinal study. Lancet Neurol 2013;12(9):873–81. doi: 10.1016/S1474-4422(13)70146-7
- Jurkiewicz MT, Mikulis DJ, McIlroy WE, Fehlings MG, Verrier MC. Sensorimotor cortical plasticity during recovery following spinal cord injury: a longitudinal fMRI study. Neurorehabil Neural Repair 2007;21(6):527–38. doi: 10.1177/1545968307301872
- Jurkiewicz M, Crawley A, Verrier M, Fehlings M, Mikulis D. Somatosensory cortical atrophy after spinal cord injury: a voxel-based morphometry study. Neurology 2006;66(5):762–4. doi: 10.1212/01.wnl.0000201276.28141.40
- Freund P, Weiskopf N, Ward NS, Hutton C, Gall A, Ciccarelli O, et al. Disability, atrophy and cortical reorganization following spinal cord injury. Brain 2011;134:1610–22. doi: 10.1093/brain/awr093
- Kirshblum S, O’Connor K. Levels of spinal cord injury and predictors of neurologic recovery. Phys Med Rehabil Clin N Am 2000;11(1):1–27, vii. doi: 10.1016/S1047-9651(18)30144-X
- Field-Fote E. Spinal cord injury rehabilitation. Philadelphia: FA Davis; 2009.
- Ahuja CS, Fehlings M. Concise review: bridging the gap: novel neuroregenerative and neuroprotective strategies in spinal cord injury. Stem Cells Transl Med 2016;5(7):914–24. doi: 10.5966/sctm.2015-0381
- Baptiste DC, Tighe A, Fehlings MG. Spinal cord injury and neural repair: focus on neuroregenerative approaches for spinal cord injury. Expert Opin Investig Drugs 2009;18(5):663–73. doi: 10.1517/13543780902897623
- Burns AS, Marino RJ, Kalsi-Ryan S, Middleton JW, Tetreault LA, Dettori JR, et al. Type and timing of rehabilitation following acute and subacute spinal cord injury: a systematic review. Global Spine J 2017;7(3_suppl):175S–94S. doi: 10.1177/2192568217703084
- Dobkin BH. Motor rehabilitation after stroke, traumatic brain, and spinal cord injury: common denominators within recent clinical trials. Curr Opin Neurol 2009;22(6):563. doi: 10.1097/WCO.0b013e3283314b11
- Winstein C, Lewthwaite R, Blanton SR, Wolf LB, Wishart L. Infusing motor learning research into neurorehabilitation practice: a historical perspective with case exemplar from the accelerated skill acquisition program. JNPT 2014;38(3):190.
- Socolovsky M, Malessy M, Lopez D, Guedes F, Flores L. Current concepts in plasticity and nerve transfers: relationship between surgical techniques and outcomes. Neurosurg Focus 2017;42(3):E13. doi: 10.3171/2016.12.FOCUS16431
- Hou JM, Yan RB, Xiang ZM, Zhang H, Liu J, Wu YT, et al. Brain sensorimotor system atrophy during the early stage of spinal cord injury in humans. Neuroscience 2014;266:208–15. doi: 10.1016/j.neuroscience.2014.02.013
- Fridén J, Gohritz A. Tetraplegia Management Update. J Hand Surg Am 2015;40(12):2489–500. doi: 10.1016/j.jhsa.2015.06.003
- Wangdell J, Carlsson G, Fridén J. Enhanced independence: experiences after regaining grip function in people with tetraplegia. Disabil Rehabil 2013;35(23):1968–74. doi: 10.3109/09638288.2013.768709
- Bunketorp-Käll L, Wangdell J, Reinholdt C, Fridén J. Satisfaction with upper limb reconstructive surgery in individuals with tetraplegia: the development and reliability of a Swedish self-reported satisfaction questionnaire. Spinal Cord 2017;55(7):664–71. doi: 10.1038/sc.2017.12
- Skirven TM, Osterman AL, Fedorczyk J, Amadio PC. Rehabilitation of the hand and upper extremity, 2-volume set: expert consult. Philadelphia: Elsevier Health Sciences; 2011.
- Fridén J, Reinholdt C, Turcsanyii I, Gohritz A. A single-stage operation for reconstruction of hand flexion, extension, and intrinsic function in tetraplegia: the alphabet procedure. Tech Hand Up Extrem Surg 2011;15(4):230–5. doi: 10.1097/BTH.0b013e31821b5896
- Waters RL, Stark LZ, Gubernick I, Bellman H, Barnes G. Electromyographic analysis of brachioradialis to flexor pollicis longus tendon transfer in quadriplegia. J Hand Surg Am 1990;15(2):335–9. doi: 10.1016/0363-5023(90)90119-C
- Wangdell J, Bunketorp-Käll L, Koch-Borner S, Fridén J. Early active rehabilitation after grip reconstructive surgery in tetraplegia. Arch Phys Med Rehabil 2016;97(6):S117–25. doi: 10.1016/j.apmr.2015.09.025
- Johanson ME, Dairaghi CA, Hentz VR. Evaluation of a task-based intervention after tendon transfer to restore lateral pinch. Arch Phys Med Rehabil 2016;97(6):S144–53. doi: 10.1016/j.apmr.2015.12.032
- Johanson ME, Hentz VR, Smaby N, Murray WM. Activation of brachioradialis muscles transferred to restore lateral pinch in tetraplegia. J Hand Surg Am 2006;31(5):747–53. doi: 10.1016/j.jhsa.2006.01.006
- Lundborg G. Brain plasticity and hand surgery: an overview. J Hand Surg Br 2000;25(3):242–52. doi: 10.1054/jhsb.1999.0339
- Devanne H, Cohen LG, Kouchtir-Devanne N, Capaday C. Integrated motor cortical control of task-related muscles during pointing in humans. J Neurophysiol 2002;87(6):3006–17. doi: 10.1152/jn.2002.87.6.3006
- McNamara A, Tegenthoff M, Dinse H, Büchel C, Binkofski F, Ragert P. Increased functional connectivity is crucial for learning novel muscle synergies. Neuroimage 2007;35(3):1211–8. doi: 10.1016/j.neuroimage.2007.01.009
- Bunketorp Kall L, Cooper RJ, Wangdell J, Friden J, Bjornsdotter M. Adaptive motor cortex plasticity following grip reconstruction in individuals with tetraplegia. Restor Neurol Neurosci 2018;36(1):73–82.
- Makin TR, Scholz J, Slater DH, Johansen-Berg H, Tracey I. Reassessing cortical reorganization in the primary sensorimotor cortex following arm amputation. Brain 2015;138(8):2140–6. doi: 10.1093/brain/awv161
- Oldfield RC. The assessment and analysis of handedness: the Edinburgh inventory. Neuropsychologia 1971;9(1):97–113. doi: 10.1016/0028-3932(71)90067-4
- Schieber MH, Santello M. Hand function: peripheral and central constraints on performance. J Appl Physiol 2004;96(6):2293–300. doi: 10.1152/japplphysiol.01063.2003
- Monfils M-H, Plautz EJ, Kleim JA. In search of the motor engram: motor map plasticity as a mechanism for encoding motor experience. Neuroscientist 2005;11(5):471–83. doi: 10.1177/1073858405278015
- Xu T, Yu X, Perlik AJ, Tobin WF, Zweig JA, Tennant K, et al. Rapid formation and selective stabilization of synapses for enduring motor memories. Nature 2009;462(7275):915. doi: 10.1038/nature08389
- Dayan E, Cohen LG. Neuroplasticity subserving motor skill learning. Neuron 2011;72(3):443–54. doi: 10.1016/j.neuron.2011.10.008
- Kargo WJ, Nitz DA. Early skill learning is expressed through selection and tuning of cortically represented muscle synergies. J Neurosci 2003;23(35):11255–69. doi: 10.1523/JNEUROSCI.23-35-11255.2003
- Stoodley CJ, Valera EM, Schmahmann JD. Functional topography of the cerebellum for motor and cognitive tasks: an fMRI study. Neuroimage 2012;59(2):1560–70. doi: 10.1016/j.neuroimage.2011.08.065
- Sokolov AA, Miall RC, Ivry RB. The cerebellum: adaptive prediction for movement and cognition. Trends Cogn Sci 2017;21(5):313–32. doi: 10.1016/j.tics.2017.02.005
- Wenger E, Brozzoli C, Lindenberger U, Lövdén M. Expansion and renormalization of human brain structure during skill acquisition. Trends Cogn Sci 2017;21(12):930–9. doi: 10.1016/j.tics.2017.09.008
- Draganski B, Gaser C, Busch V, Schuierer G, Bogdahn U, May A. Neuroplasticity: changes in grey matter induced by training. Nature 2004;427(6972):311–2. doi: 10.1038/427311a
- Taubert M, Mehnert J, Pleger B, Villringer A. Rapid and specific gray matter changes in M1 induced by balance training. Neuroimage 2016;133:399–407. doi: 10.1016/j.neuroimage.2016.03.017
- Sampaio-Baptista C, Scholz J, Jenkinson M, Thomas AG, Filippini N, Smit G, et al. Gray matter volume is associated with rate of subsequent skill learning after a long term training intervention. Neuroimage 2014;96:158–66. doi: 10.1016/j.neuroimage.2014.03.056
- Lateiner JE, Sainburg RL. Differential contributions of vision and proprioception to movement accuracy. Exp Brain Res 2003;151(4):446–54. doi: 10.1007/s00221-003-1503-8
- Wester K, Hove LM, Barndon R, Craven AR, Hugdahl K. Cortical plasticity after surgical tendon transfer in tetraplegics. Front Hum Neurosci 2018;12:234 doi: 10.3389/fnhum.2018.00234
- Schabrun SM, Ridding MC. The influence of correlated afferent input on motor cortical representations in humans. Exp Brain Res 2007;183(1):41–9. doi: 10.1007/s00221-007-1019-8
- Latash ML. Biomechanics as a window into the neural control of movement. J Hum Kinet 2016;52(1):7–20. doi: 10.1515/hukin-2015-0190
- Lyle MA, Nichols TR, Kajtaz E, Maas H. Musculotendon adaptations and preservation of spinal reflex pathways following agonist-to-antagonist tendon transfer. Physiol Rep 2017;5(9):e13201. doi: 10.14814/phy2.13201
- Fridén J, Shillito MC, Chehab EF, Finneran JJ, Ward SR, Lieber RL. Mechanical feasibility of immediate mobilization of the brachioradialis muscle after tendon transfer. J Hand Surg 2010;35(9):1473–8. doi: 10.1016/j.jhsa.2010.06.003
- Cantou P, Platel H, Desgranges B, Groussard M. How motor, cognitive and musical expertise shapes the brain: focus on fMRI and EEG resting-state functional connectivity. J Chem Neuroanat 2018;89:60–8. doi: 10.1016/j.jchemneu.2017.08.003
- Nudo RJ, Plautz EJ, Frost SB. Role of adaptive plasticity in recovery of function after damage to motor cortex. Muscle Nerve 2001;24(8):1000–19. doi: 10.1002/mus.1104
- Murray WM, Hentz VR, Fridén J, Lieber RL. Variability in surgical technique for brachioradialis tendon transfer: evidence and implications. J Bone Joint Surg Am 2006;88(9):2009–16.