Abstract
Effective moisture diffusivity of cylindrical apple samples (0.7 and 1.4 cm diameter) was determined under convective hot air drying (40, 50, 60, and 70° C and 1.5 and 3 m/s air velocity). The effective moisture diffusivities obtained were in the range of reported values and were found to be temperature dependent according to the Arrhenius relationship. However, the prediction of moisture loss obtained from Fick's diffusion model failed to follow experimental drying curves. Temperature profiles during convective hot air drying showed temperature gradients. This lack of isothermal conditions may lead to inaccurate predictions of moisture loss. Therefore, a combined microwave-convective hot air apparatus, capable of providing isothermal drying conditions, was used to quantify the drying kinetics. Differences in effective diffusivities determined from convective (9.6–18.5 3 10210 m2/s) and isothermal (20.3–37.6 3 10210 m2/s) drying experiments were shown. Using effective diffusivities obtained under isothermal conditions, the Fickian model still did not predict moisture loss during isothermal drying. Due to the developing porous structure of apple during drying, it was hypothesized that drying of a hygroscopic porous material is limited by evaporation of water to water vapor. Therefore, an irreversible first-order kinetic model was proposed to predict isothermal drying of apple. Using the rate constant calculated from the slope of the normalized drying curves (0.08–0.17 min21), the model predicted accurate moisture loss at each temperature throughout the entire moisture range of 0.06–8.5 g/g dry solid.
Introduction
Drying of food materials has been widely accepted as an internally controlled process,[Citation1,Citation2,Citation3] where Fick's second law of diffusion, as shown in Eq. (Equation1), has been used to predict moisture loss during drying.[Citation4,Citation5]
Effective moisture diffusivity (Deff) in this equation is the important parameter that lumps together all of the internal moisture transfer mechanisms. The effective moisture diffusivity is typically determined experimentally from drying curves.[Citation6] In experimentally determining the effective moisture diffusivity, isothermal conditions of the sample are required during drying. The effective diffusivity is strongly affected by temperature. Generally, the temperature dependence can be described by the Arrhenius relationship.[Citation2,Citation7–10] The activation energy (Ea) can be determined from the slope of the Arrhenius plot, ln(Deff ) vs 1/T.
Many researchers have suggested that the discrepancies between the experimental data and model predictions result from the fact that the effective diffusion coefficient is determined when sample temperature gradients exist.[Citation11–13] Most biological materials have a modified Lewis number (Lem = α/D eff ) >60, indicating that thermal gradients or heat transfer effects could be neglected during drying.Citation3] This is consistent with the observation of several researchers that very small temperature gradients existed between the surface and center of various food materials undergoing air-drying.[Citation2,Citation7,Citation8,Citation14,Citation15] However, the sample temperature was found to be time dependent. The sample temperature increased rapidly at the beginning of drying toward the air dry-bulb temperature but did not reach the drying temperature until after about 90% of the moisture content was removed.Citation8,Citation13,Citation15,Citation16] With the temperature not being constant, the difficulty in determining the effective diffusivity and the activation energy is apparent.
Microwave energy and convective hot air were used to develop an isothermal drying apparatus and its application was shown in bread.[Citation17] With isothermal conditions being established immediately and maintained throughout drying as well as on-line sample weight measurements, the apparatus is suitable for determining drying kinetics and investigating the mechanism(s) that limit the drying process for a variety of food materials. The drying kinetics of potato, carrot core and carrot cortex, which are model hygroscopic non-porous materials, were analyzed using the isothermal apparatus and significant differences, as high as 40%, were shown between effective diffusivities measured under convective hot air and isothermal conditions.[Citation18] When the moisture diffusivity was experimentally determined under isothermal conditions, the diffusion equation accurately predicted moisture loss in the potato and carrot samples. The relevance of measuring effective diffusivities under isothermal conditions along with its temperature dependence is the ability to quantify the moisture transfer within a food material experiencing variable temperature gradients, which is the actual temperature profile of a food during conventional dehydration.
In addition to the isothermal assumption, if necessary, dependence of effective diffusivity on moisture content will also be taken into consideration in order to improve accuracy of the diffusion model. The diffusion coefficient as a function of moisture content can be estimated by an analysis of the experimental drying curves applying one of the following methods: the method of slopes[Citation19] and the regular regime method.[Citation20] The first approach requires experimental sorption curves for different equilibrium moisture contents (Me). This method is based on the assumption that the effective diffusivity does not change significantly during drying as long as the moisture loss is small.[Citation21] Thus, the drying curves were obtained at different initial and equilibrium moisture contents with Me slightly lower than the initial moisture content. The effective moisture diffusivity at a given moisture concentration can be determined from the corresponding drying curve by plotting the logarithm of the unaccomplished moisture ratio vs. time. The effective moisture diffusivity as a function of moisture content can then be determined by regression analysis. For the second method, the regular regime theory[Citation9,Citation22] is applied to systems in which the moisture diffusivity decreases with decreasing moisture content below the critical moisture content or for cases where the drying rate is governed by mass transfer inside the drying material. Regular-regime period is defined as the time during a transient diffusion process in which the concentration changes with time are taken into account, but the effect of the initial condition on the process is neglected.Citation5] The regular regime characterizes the internal diffusion process at sufficiently long drying times such that the moisture profiles inside the material are no longer dependent on the initial moisture content but dependent on the diffusion coefficient. This technique requires experimental determination of the regular regime curve at constant surface concentration and at a desired temperature.[Citation5,Citation23]
However, several studies have shown that the Fick's diffusion model was unable to fully describe isothermal drying of a hygroscopic porous material.[Citation24,Citation25,Citation26] Roberts and TongCitation17] found that the diffusion model failed to accurately predict moisture loss during drying of bread, even though the effective diffusivity was properly measured from drying curves under isothermal conditions. It was hypothesized that the rate at which heat can provide the heat of vaporization necessary to vaporize the moisture within a hygroscopic porous material is the rate limiting mechanism and not diffusion. They proposed a first-order irreversible kinetic model that describes that the rate of moisture loss is limited by internal evaporation of bound water to water vapor:
The first-order irreversible kinetic model was shown to accurately predict moisture loss during isothermal drying of porous bread samples.[Citation27] Apple has the bulk porosity of 0.21 after blanching,[Citation28] close to the porosity of a porous material (above 0.25).[Citation29] Furthermore, apple has shown to develop significant porosity (20% to 70%) during drying.[Citation30,Citation31] Since apple develops into a porous material during drying, the hypothesis of this research is that the drying kinetics of apple can be described by the first-order kinetic model.
The objectives of this research were to determine effective diffusivity of apple during convective hot air and isothermal drying and to compare moisture loss predicted from the diffusion and first-order kinetic models to experimental data.
Materials and Methods
Sample Preparation
Cylindrical samples of apple (Red Delicious) were obtained using cylindrical cutters so that the length to diameter ratio was 10. The samples were blanched in a water bath (Isotemp 2028P, Fisher Scientific, Pittsburgh, PA, USA) to inactivate enzymes at 90° C for 50 sec.[Citation32] Initial moisture content of apple samples were determined in a vacuum oven (Model 3608, Lab-Line Instrument, Inc., Melrose Park, IL, USA) by an oven-drying method.Citation33] The cylindrical samples used in drying experiment were sealed at top and bottom ends with epoxy (Devcon 5 minute fast drying epoxy, ITW Brands, Wood Dale, IL, USA) to promote one-dimensional moisture loss. This is an ideal sealant since it does not separate from the food materials as it shrinks during drying.
Experimental Design
Drying kinetic and temperature profile experiments for both convective hot air and isothermal drying were performed using 0.7 cm diameter cylindrical samples at drying temperatures of 40, 50, 60, and 70° C and at an air velocity of 3 m/s. In addition to these experimental conditions, the effects of sample size and air velocity on the effective diffusivity during convective hot air drying were studied. The effect of sample size was studied using cylindrical samples with diameter of 0.7 and 1.4 cm. The effect of air velocity was studied on 0.7 cm diameter samples using air velocities of 1.5 and 3 m/s and drying temperatures of 50 and 70° C. Each experimental condition was conducted in duplicate. Statistical analysis was performed on the significance of the drying parameters on the effective moisture diffusivity using a general linear model.
Convective Hot Air Drying
The apparatus used to obtain drying curves and temperature profiles during convective hot air drying is described by Roberts et al.[Citation34] The weight loss of the samples was measured on-line every 15 seconds throughout drying. Moisture contents at each time interval were calculated from both weight loss data and dry solid weight of the sample, which was determined at the end of drying by the oven-drying method.[Citation33] The effective moisture diffusivity was determined experimentally from the drying curves (plots of moisture content and time) at different temperatures. Based on the assumption that the effective diffusivity is not dependent on moisture content, the solution of Fick's second law equation for a one-dimensional cylinder can be obtained as follows:
or
where M* represents the unaccomplished moisture content, J0(x) is the Bessel function of the first kind of order zero, αn ’s are the roots of this function and Fick number
For long drying times when the Fick number is greater than 0.1 or M* is less than 0.6, only the first term of the series solution is used and the effective moisture diffusivity can be determined from the slope of the linear plot of the logarithm of the unaccomplished moisture ratio (ln M – Me /Mi – Me ) vs time using the following equation:[Citation5,Citation21]
The equilibrium moisture content (Me ) was obtained by extending the drying time until no measurable weight loss was observed. The equilibrium moisture content was also confirmed with respect to the temperature and humidity of the hot air conditions and moisture isotherms for apple proposed by Roman et al.[Citation35] By knowing the initial moisture content, equilibrium moisture content and Deff determined from drying curves, moisture loss as a function of time can be calculated using Eq. (Equation4).
Temperature profiles during convective hot air drying of both 0.7 and 1.4 cm diameter samples were obtained along the radial axis: center, half-radius and surface. Temperature at the center and half-radius were measured using type J thermocouples (5SC-TT-J-36–72, Omega Engineering, Inc., Stamford, CT, USA). Due to the difficulty in placing and maintaining a thermocouple at the surface, a remote infrared (IR) temperature sensor (Thermalert IT Series, Raytek, Inc., Santa Cruz, CA, USA) with type J thermocouple output was used to obtain accurate surface temperature measurements. Details of the temperature mapping apparatus along with the procedures of determining the emissivity of the samples for accurate IR sensor readings are described by Roberts et al.[Citation34]
Isothermal Drying
The isothermal drying apparatus includes microwave energy with continuous variable power control, convective hot air, a feed-back temperature controller, and an analytical balance, as shown in . This apparatus design was developed by Roberts and Tong[Citation17] in obtaining isothermal conditions in porous bread samples. The temperature of the sample was controlled by an on/off system in the previous design, and this control scheme was upgraded to a PID controller to allow continuous power to the sample in this study. After the desired sample temperature is obtained, the power requirement to maintain a sample within drying temperatures is less than 200 W and reduces as drying proceeds, which is between 30% and 1% of the maximum power from the generator. To obtain stable power delivery at low power levels, the waveguide configuration was modified using an inverted variable isolator, which consists of a 3-port circulator and a dummy load with an adjustable power reflecting stub (GAE, Inc., Modesto, CA, USA). With this waveguide configuration, microwave power delivered from the generator will be directed to the dummy load with the adjustable stub. Therefore, the power delivered to the working load can be adjusted using this adjustable stub in order to obtain an optimum forward power level for isothermal drying at each temperature.
Temperatures of the hot air and sample were measured using optical fiber temperature probes and a multi-channel temperature sensing unit (Model 790 Fluoroptic Thermometry System, Luxtron Cooperation, Sata Clara, CA, USA). Two probes, one at the center and other at the surface, were used to monitor the sample temperatures and verify isothermal conditions. A program developed by Gerling Applied Engineering (Modesto, CA, USA) was used to acquire the time-temperature data and to control the sample temperature. The temperature at the center was used as the basis for controlling the input power from the generator. The convective hot air brought into the oven cavity was generated using a heat gun (Model CH 6056, Leister, Kagiswil, Switzerland) and a blower (Model 4C942, Electric Motor Warehouse, Dayton, OH, USA). The flow rate and temperature of the hot air were controlled by variable transformers (Type 3PN1010B for the blower, and Type 3PN2210B for the heater, Staco Energy Products, Co., Dayton, OH, USA). The surface temperature throughout drying was manually controlled by adjusting the variable transformer for the heater.
The drying kinetic experiments were initiated after isothermal conditions had been verified. Only one optical fiber temperature probe was used at the center to monitor and control the temperature during weight loss measurement. To obtain isothermal conditions during the drying kinetic experiments, the microwave power, and the temperature and velocity of hot air were kept the same as those used in the temperature profile experiments. The drying curves obtained during isothermal drying were used to determine Deff as described earlier.
Moisture dependence of effective diffusivity was determined using simplified method of slopes.[Citation36] In the general case of nonlinear drying curve, the method of slopes can be applied to estimate the effective moisture diffusivity at various moisture contents by comparing the experimental drying curve to the theoretical curve for a given shape of material. For a cylindrical shape, the theoretical curve of natural log of the unaccomplished moisture content (logM*) and Fourier number for diffusion (Fo = Dt/r2) can be obtained directly from the series solution (equation Equation4). By assuming that the diffusion equation can be applied to sections of the drying curves, the slopes of the experimental drying curve (dM*/dt)exp and the theoretical curve (dM*/d Fo)th were estimated at a given moisture ratio (M*). The effective moisture diffusivity (Deff) at a given moisture ratio (M*) or moisture content (M) was then calculated from the following equation:
where r = cylindrical radius of the sample. From equation Equation6, the estimated values of Deff vary with the moisture content as a result of change in the slope of the drying curve during drying.
Evaporation was also evaluated as the mechanism for moisture loss in the apple samples. Upon integration of Eq. Equation2, the first-order reaction equation can be obtained as shown in the following equation:
Eqs. 2 and 7 represents a chemical reaction that has proceeded to completion, so the reactant M is zero at infinite time. If the reaction goes to some equilibrium value (Me) at infinite time, the equation becomes:
Like the effective moisture diffusivity, the rate constant (k1) can be determined from the slope of the linear plot of the natural log of unaccomplished moisture content vs. time. To verify a first-order reaction, the log of the unaccomplished moisture content vs time should be a straight line for more than one log cycle.[Citation27] The time it takes for the unaccomplished moisture content to reduce by one log cycle at a given temperature (D-value) can be determined using the following equation:
From Eqs. (8) and (9), the relationship between the rate constant (k1) and D-value can be obtained:
Also, from Eq. (Equation8), the moisture loss as a function of time can be calculated as follows:
By substituting the initial moisture content, equilibrium moisture content and the rate constant obtain from the drying curves into Eq. (Equation11), the moisture content at each drying time interval (same as time interval for weight loss data) can be calculated.
Results and Discussion
Convective Hot Air Drying
Drying kinetics
The plot of the experimental drying curve of 0.7 diameter apple is shown in . Analyzing the rate of moisture loss, there was no constant-rate period of drying and the falling-rate period occurred immediately. The normalized drying curve () shows a linear relationship between the logarithm of the unaccomplished moisture content and time. The drying curves and normalized drying curves at other drying conditions, not shown, were very similar to , respectively. The effective moisture diffusivities, calculated from the slope of the normalized drying curves for dimensionless moisture content below 0.6 using equation (Equation5), are given in .
Figure 2 Convective drying curves of 0.7 cm diameter apple sample at 60° C and 3 m/s air velocity: a) moisture content (g/g dry solid) vs time; b) natural log of the unaccomplished moisture content vs time.
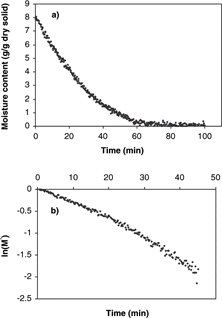
Table 1 Comparison of Deff and Ea values determined from convective and isothermal experiments.
The range of moisture contents in which Deff was determined from the slope of the plot of ln M* vs time was 5.44–0.72 g/g dry solid and the average value of the correlation coefficient (R2) from the regression analysis of the straight line was 0.97. The values of Deff were in the range of reported diffusivities in literature.[Citation6,Citation36] The statistical analysis showed significant differences between conditions I (1.5 m/s, 0.7 cm) and III (3.0 m/s, 0.7 cm) and between conditions II (3.0 m/s, 1.4 cm) and III (3.0 m/s, 0.7 cm). Therefore, the sample size and air velocity had significant effect on the effective moisture diffusivity, which was consistent with the findings in convective drying of porous bread samples reported by Roberts et al.[Citation33] According to Jason,[Citation7] when the air velocity is 1 m/s or above, which was the case in this study, air velocity should not have an effect on drying. In addition, according to Fick's diffusion equation, the sample size was taken into account in calculating the effective moisture diffusivity so the effect of sample size should not be significant. It was hypothesized that the development of porosity during drying may influence the transport mechanism of moisture through the material and a different mechanism other than diffusion may contribute to moisture transfer in apple.
The relationship of the effective moisture diffusivity and the dry-bulb temperature follows the Arrhenius equation:
The activation energy (Ea) was determined from the slope of the Arrhenius plot as shown in .
Prediction of moisture loss
Based on assumptions of a uniform initial moisture distribution, constant diffusion coefficient, negligible external resistance, negligible temperature gradients and negligible shrinkage during drying, the solution of Fick's second law equation for an infinite cylinder (Eq. Equation4) was used to predict moisture loss during convective drying. Predicted values of moisture content and experimental data were compared as shown in . The results showed discrepancies between experimental data and model predictions. Inaccuracy of the model may result from a violation of one or more assumptions during experimental Deff measurements, such as negligible heat transfer effect, negligible dependence of the effective diffusivity on moisture content and negligible shrinkage effect.
Temperature profiles
The results of temperature profiles of the 0.7 and 1.4 cm diameter samples during convective hot air drying at 70° C are shown in . Similar temperature profiles were obtained at 40, 50 and 60° C. Temperature histories and gradients were observed within the samples. With the sample temperature varying with position and time during convective drying, it is difficult to properly determine the effective moisture diffusivity and its dependence on temperature. From the results of temperature profiles, it is apparent that the isothermal assumption is violated and thus could be the most significant cause of error. Therefore, the first step in analyzing the internal moisture transfer is to eliminate the heat transfer effect thus allowing for proper measurement and analysis of the diffusion coefficients.
Isothermal Drying
Temperature profiles
(a–d) show isothermal temperature profiles of 0.7 cm diameter apple samples during combined microwave-convective hot air drying at 40–70° C. The sample temperatures reached the desired temperature within a short time and were maintained at this temperature throughout drying. The center temperature, the basis for controlling the input power, reached the desired temperature immediately. The optimal initial microwave power levels of 1750 W (for drying at 60 and 70° C) and 250 W (for drying at 40 and 50° C) were required to prevent overshooting of sample temperatures, especially at the center. The surface reached the target temperature slower than the center due to evaporative cooling effects from the wet surface. To establish the target temperature at the surface as quickly as possible, the temperature of hot air had to be much greater than the desired drying temperature to counter the evaporative cooling effects. The average starting air temperature for isothermal experiments (40–70° C) of apple was 40° C higher than the target temperature. As the drying proceeded, the moisture content at the sample surface decreased, and thus the evaporative cooling effects diminish. The air temperature was manually reduced as the surface dried, and eventually the air temperature equaled the target temperature. After the surface reached the target temperature, the center and surface temperatures were maintained at the desired drying temperature largely by the convective hot air where the microwave power was less than 30 W.
Drying kinetics
Drying curves and normalized drying curves obtained from isothermal drying experiments were similar to those from convective drying experiments. However, by analyzing the rate of moisture loss, there existed two falling-rate periods. The effective moisture diffusivity and activation energy values of apple samples with 0.7 cm diameter obtained during isothermal drying are summarized in . Diffusivity values from isothermal drying experiments (condition IV) were significantly higher than those from convective hot air drying experiments (condition III). Since uniform sample temperatures were quickly attained and maintained, the effective diffusivity and activation energy values should be more accurate than the value determined during the convective drying experiments.
Prediction of moisture loss
Predicted moisture loss obtained from the Fickian diffusion model was compared with experimental moisture loss during isothermal drying, as shown in for 60° C. The results showed that the Fickian model provided better predictions when effective moisture diffusivity values were experimentally determined under isothermal conditions. This supports the hypothesis that the heat transfer effect contributes to the observed discrepancies between experimental data and model prediction. However, the diffusion model still grossly over-predicted experimental moisture loss of isothermal drying. Therefore, the assumption of negligible dependence of the effective diffusivity on moisture content was changed to assume a moisture dependent effective diffusivity.
Figure 7 Diffusion model predictions of 0.7 cm diameter samples during convective hot air drying at 60° C and 3.0 m/s air velocity.
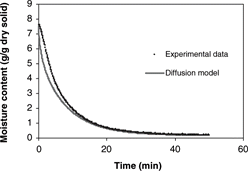
The effective moisture diffusivities of apple at various moisture contents for each isothermal drying temperature obtained from the simplified method of slopes were shown in . A mathematical model was developed to fit the values of effective moisture diffusivity (the dependent variable) as a function of moisture content and temperature (independent variables). From preliminary analysis of the data, the following empirical equation was proposed:
Figure 8 The estimated effective moisture diffusivities at variours moisture contents for each drying temperature (40-70° c) obtained from the method of slops.
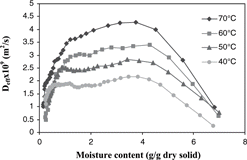
where Po, P1, P2, P3 and P4 were unknown parameters. This equation is of the same form as that of Singh et al.[Citation20] Eq. Equation13 was linearized by logarithmic transformation and a linear regression was performed to determine the unknown parameters. By substituting these parameters, Eq. Equation13 can be given as:
Agreement of the experimental data and predicted effective diffusivities (r2 = 0.744) was shown in (A–D). Goodness of fit (r2) was estimated from:[Citation38]
Figure 9 The effective moisture diffusivity obtained from the slope method compared to predictions obtained from the linear regression analysis at drying temperatures of a) 70° C; b) 60° C; c) 50° C; d) 40° C.
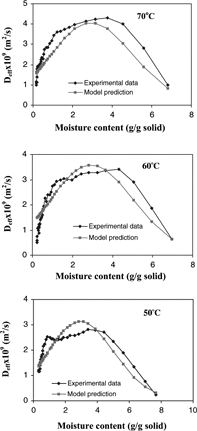
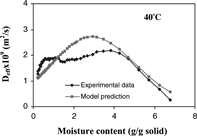
where Y = values of independent variables (Deff)
Effective moisture diffusivities calculated from Eq. Equation14 as a function of moisture content for each isothermal drying temperature were used in Fick's diffusion model for predicting average moisture loss of apple. With moisture dependent Deff, the model provided better predictions as shown in . However, there still existed discrepancies between experimental data and model predictions. This indicates that the diffusion model was unable to fully describe the isothermal drying of apple.
Figure 10 Predictions obtained from diffusion model using moisture dependent Deff for 0.7 cm diameter apple during isothermal drying at 60° C and 3 m/s air velocity.
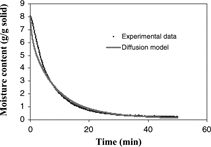
From the plot of effective moisture diffusivity as a function of moisture content (), the effective diffusivity increased as the drying proceeded and maintained at the maximum value in the moisture range of 4.0–1.0 g/g dry solid. This characteristic of the moisture diffusivity of apple may result from change in moisture transfer mechanism during drying. In the first stage of drying, moisture transfer might be limited by liquid diffusion. As the drying proceeded, moisture content decreased and a porous structure was formed where vaporization or vapor diffusion through pore spaces may be the dominant moisture transfer mechanism. In the final stage of drying at moisture contents below 1 g/g dry solid, the diffusivity decreased sharply because water was more tightly bound.
However, with the decreasing diffusivity taken into consideration, the diffusion model still over predicted the moisture loss. According to Zogzas et al.,[Citation31] apple has shown to develop into a porous material with porosity greater than 0.25 when moisture content decreased to the level below 4.5 g/g dry solid. This is consistent with the moisture content at which the diffusivity was maximum (). Therefore, the change in porosity of apple during drying affected the moisture transfer mechanism and thus the effective moisture diffusivity. The low porosity in the early stage of drying corresponded to the low effective moisture diffusivity. High porosity developed in the dried layer of the sample towards the latter half of isothermal drying facilitated the transfer of water vapor, as indicated by high effective moisture diffusivity.
Unlike the case of low porosity in which liquid diffusion predominates, in the case of high porosity, the diffusion equation was not adequate for describing the moisture transport[Citation39] which is likely water vapor transfer or vaporization. Tong and Lund[Citation40] and Roberts and Tong[Citation17] found that the diffusion model based on Fick's second law was unable to fully describe the drying characteristics of bread under isothermal drying. Tong et al.[Citation24] measured the pressure profiles in bread samples as a function of sample porosity during microwave heating. The results showed that pressure gradient was negligible when the porosity was greater than 0.3. It was hypothesized that internal pressure build-up is negligible because water vapor transfer is significantly greater than vaporization. Therefore, they proposed that isothermal drying of a hygroscopic porous material is controlled by vaporization rather than vapor diffusion.
Roberts and Tong[Citation27] showed that modeling evaporation of bound water to water vapor using a first-order irreversible kinetic model impressively predicted the isothermal drying of porous bread samples. Thus, the first-order kinetic model was proposed to predict the moisture loss of apple during isothermal drying. The rate constant values as summarized in were determined from the slope of the normalized drying curves in the range of unaccomplished moisture content (M*) from 0.6 to 0.25, where the major falling rate period was observed from the drying rate analysis. Also, D-values, calculated from Eq. Equation10, were given in . The relationship between the rate constant and temperature follows the Arrhenius equation:
Table 2 The rate constant (k1) and D-values obtained from isothermal experiments of 0.7 cm diameter apple samples, at 40–70° C, and the air velocity of 3 m/s.
The activation energy (Ea) of 22.70 kJ/mol and the constant k0 of 503.58 min−1 were obtained by the regression analysis of the Arrhenius plot. By knowing the rate constant value at each temperature, predicted moisture loss can be calculated from the first-order kinetic model (Eq. Equation11) and was compared with experimental data as shown in . The model was shown to accurately predict the isothermal drying of apple samples throughout the entire moisture range, from 8.5 to 0.06 g/g dry solid.
Conclusion
Although the effective diffusivity was accurately measured from drying curves under isothermal conditions, Fick's model was unable to describe the isothermal drying of apple. A first-order irreversible kinetic model based on evaporation of moisture in the sample accurately predicted moisture loss during isothermal drying of apple. This implies that the isothermal drying of apple is vaporization limited and that heat transfer is the rate limiting mechanism.
Nomenclature
D | = |
moisture diffusivity, m2/s |
Deff | = |
effective diffusivity, m2/s |
D0 | = |
diffusivity constant, m2/s |
Ea | = |
activation energy, kJ/mol |
k1 | = |
first-order rate constant, min−1 |
k0 | = |
reference rate constant, min−1 |
M | = |
moisture content, kg H2O/kg dry solid |
Mi | = |
initial moisture content, kg H2O/kg dry solid |
Me | = |
equilibrium moisture content, kg H2O/kg dry solid |
Mt | = |
moisture content at time t, kg H2O/kg dry solid |
P0-P4 | = |
parameters in Eq. Equation13 |
Rc | = |
universal gas constant,= 8.314 × 10−3 kJ/mol K |
r | = |
radial dimension from surface in a cylinder or sphere, m |
T | = |
absolute temperature, K |
t | = |
time, second |
α | = |
thermal diffusivity, m2/s |
Acknowledgments
This research was supported by the New York State Agricultural Experiment Station federal formula funds no. NYG-623568 received from CSREES, USDA.
Notes
aThe linear relationships (between log Deff and 1/T) for the four drying conditions were evaluated by regression analysis. Slopes from the four drying conditions were uniform (−59.79 ± 1.86) and intercepts differed significantly from each other (3.71 ± 0.04, 3.58 ± 0.04, 3.95 ± 0.04, 4.43 ± 0.04, p < 0.001).
References
- King , C.J. 1968 . Rates of moisture sorption and desorption in porous dried foodstuffs . Food Technol. , 22 ( 502 ) : 165 – 171 .
- Saravacos , G.D. and Charm , S.E. 1962 . A study of the mechanism of fruit and vegetable dehydration . Food Technol. , 16 ( 1 ) : 78 – 81 .
- Young , J.H. 1969 . Simultaneous heat and mass transfer in a porous hygroscopic solid . Trans. ASAE. , 12 : 720 – 725 .
- Karel , M. 1975 . “ Dehydration of foods ” . In Principles of Food Science. Part II: Physical Principles of Food Preservation , Edited by: Fennema , O.R. , Leud , D.B. and Karel , M. 309 – 332 . New York : Marcel Dekker, Inc. .
- Rizvi , S.S.H. 1986 . “ Thermodynamic properties of foods in dehydration ” . In Engineering Properties of Foods , Edited by: Rao , M.A. and Rizvi , S.S.H. 133 – 214 . New York : Marcel Dekker, Inc. .
- Saravacos , G.D. and Maroulis , Z.B. 2001 . Transport Properties of Foods , 415 New York : Marcel Dekker, Inc. .
- Jason , A.C. 1958 . “ A study of evaporation and diffusion processes in the drying of fish muscle ” . In Fundamental Aspects of Dehydration of Foodstuffs , 103 – 135 . New York : Soc. Chem. Ind.: London and MacMillan Co. .
- Vaccarezza , L.M. , Lombardi , J.L. and Chirife , J. 1974a . Kinetics of moisture movement during air drying of sugar beet root . J. Food Technol. , 9 : 317 – 327 .
- Luyben , K.A.M. , Olieman , J.J. and Bruin , S. 1980 . “ Concentration dependent diffusion coefficients derived from experimental drying curves ” . In Drying ‘80 , Edited by: Mujumdar , A.S. Vol. 2 , 233 – 243 . New York : Hemisphere Publishing Corp. .
- Suarez , C. , Viollaz , P. and Chirife , J. 1980 . Diffusional analysis of air drying of grain sorghum . J. Food Technol. , 15 : 523 – 531 .
- Chen , C.S. and Johnson , W.H. 1969 . Kinetics of moisture movement in hygroscopic materials. I. Theoretical considerations of drying phenomena . Trans. ASAE , 12 : 109 – 113 .
- Vaccarezza , L.M. , Lombardi , J.L. and Chirife , J. 1974b . Heat transfer effects on drying rate of food dehydration . Can. J. Chem. Eng. , 52 : 576 – 579 .
- Chirife , J. 1983 . “ Fundamentals of the drying mechanism during air dehydration of foods ” . In Advances in Drying , Edited by: Mujumdar , A.S. Vol. 2 , 73 – 102 . New York : Hemisphere Publishing Corporation .
- Chirife , J. 1971 . Diffusional process in the drying of tapioca root . J. Food Sci. , 36 : 327 – 330 .
- Alzamora , S.M. , Chirife , J. and Voillaz , P. 1979 . A simplified model for predicting the temperatures of foods during air dehydration . J. Food Technol. , 14 ( 4 ) : 369 – 380 .
- Rovedo , C.O. , Suarez , C. and Viollaz , P.E. 1995 . Drying of foods: evaluation of a drying model . J. Food Eng. , 26 ( 1 ) : 1 – 12 . [CROSSREF]
- Roberts , J.S. and Tong , C.H. 2003a . The development of an isothermal drying apparatus and the evaluation of the diffusion model on hygroscopic porous material . Int. J. Food Prop. , 6 ( 1 ) : 165 – 180 . [CROSSREF]
- Srikiatden , J. and Roberts , J.S. 2005 . Measuring moisture diffusivity of potato and carrot (core and cortex) during convective hot air and isothermal drying . J. Food Eng. , (in press)
- Perry , R.H. 1984 . In Perry's Chemical Engineers’ Handbook, 6th Ed. , Edited by: Green , D.W. , Perry , R.H. and Maloney , J.O. London : McGraw-Hill .
- Singh , R.K. , Lund , D.B. and Buelow , F.H. 1984 . “ An experimental technique using regular regime theory to determine moisture diffusivity ” . In Engineering and Food , Edited by: McKenna , B.M. Vol. 1 , 415 – 423 . London : Elsevier Applied Science Publishers, B.V. .
- Crank , J. 1975 . In The Mathematics of Diffusion , 2nd , London : Oxford University Press .
- Schoeber , W.J.A.H. Regular Regimes in Sorption Processes . 1976 . Ph.D. Thesis, Eindhoven University of Technology, The Netherlands
- Gekas , V. 1992 . Transports Phenomena of Foods and Biological Materials , Boca Raton, FL : CRC Press, Inc. .
- Tong , C.H. , Fu , Y.C. and Lund , D.B. 1990 . Temperature, pressure, and moisture content of porous and non-porous materials during microwave heating, . IDS ‘90 Seventh International Drying Symposium . Aug 26–30 1990 , Prague, Czechoslovakia.
- Parent , A. , Tong , C.H. and Lund , D.B . 1993 . Temperature and moisture distributions in porous food materials during microwave heating, Sixth International Congress on Engineering and Food . May 23–27 1993 .
- Doulia , D. , Tzia , K. and Gekas , V. A . 2000 . knowledge for the apparent mass diffusion coefficient (Deff) of foods . Int. J. Food Prop. , 3 ( 1 ) : 1 – 14 .
- Roberts , J.S. and Tong , C.H. 2003b . Drying kinetics of hygroscopic porous materials under isothermal conditions and the use of a first-order reaction kinetic model for predicting drying . Int. J. Food Prop. , 6 ( 3 ) : 355 – 367 . [CROSSREF]
- Karathanos , V.T. , Kanellopoulos , N.K. and Belessiotis , V.G. 1996 . Development of porous structure during air drying of agricultural plant products . J. Food Eng. , 29 : 167 – 183 . [CROSSREF]
- Waananen , K.M. and Okos , M.R. 1996 . Effect of porosity on moisture diffusion during drying of pasta . J. Food Eng. , 28 : 121 – 137 . [CROSSREF]
- Lozano , J.E. , Rotstein , E. and Urbicain , M.J. 1983 . Shrinkage, porosity, and bulk density of foodstuffs at changing moisture contents . J. Food Sci. , 48 : 1497 – 1502 .
- Zogzas , N.P. , Maroulis , Z.B. and Marinos-Kouris , D. 1994 . Densities, shrinkage and porosity of some vegetables during air drying . Drying Technology , 12 ( 7 ) : 1653 – 1666 .
- Sjoholm , I. and Gekas , V. 1995 . Apple shrinkage upon drying . J. Food Eng. , 25 : 123 – 130 . [CROSSREF]
- AOAC. 1990 . Official Methods of Analysis , 15th , Vol. 2 , 614 Arlington : Association of Official Analytical Chemists, Inc. .
- Roberts , J.S. , Tong , C.H. and Lund , D.B. 2002 . Drying kinetics and time-temperature distribution of pregelatinized bread . J. Food Sci. , 67 ( 3 ) : 1080 – 1087 .
- Roman , G.N. , Urbicain , M.J. and Rotstein , E. 1982 . Moisture equilibrium in apples at several temperatures: experimental data and theoretical constiderations . J. Food Sci. , 47 : 1484 – 1488 .
- Karathanos , V.T. , Villalobos , G. and Saravacos , G.D. 1990 . Comparison of two methods of estimation of the effective moisture diffusivity from drying data . J. Food Sci. , 55 ( 1 ) : 218 – 224 .
- Sablani , S. , Rahman , S. and Al-Habsi , N. 2000 . “ Moisture diffusivity in foods—an overview ” . In Drying Technology in Agriculture and Food Sciences , Edited by: Mujumdar , A.S. 35 – 59 . Enfield, NH : Science Publishers, Inc. .
- Motulsky , H. and Christopoulos , A. 2004 . Fitting Models to Biological Data Using Linear and Nonlinear Regression , 32 – 37 . London : Oxford University Press .
- Briun , S. and Luyben , K.A.M. 1990 . “ Drying of food materials: a review of recent developments ” . In Advances in Drying , Edited by: Mujumdar , A.S. Vol.1 , 155 – 215 . New York : Hemisphere Publ. Corp. .
- Tong , C.H. and Lund , D.B. 1993 . Microwave heating of baked dough products with simultaneous heat and moisture transfer . J. Food Eng. , 19 : 319 – 339 . [CROSSREF]