Abstract
Rice dreg protein could be a valuable source of plant-based proteins, as an alternative to soy proteins in some food products. Here, nutritional properties of rice dreg protein were compared with those of soy protein isolate. The protein content of rice dreg protein was approximately 62.6 g/100 g sample, with large amounts of fat, carbohydrate, and ash. The denaturation temperatures of rice protein isolate from rice dreg protein were 47.4 and 97.2°C, respectively. This indicated that these proteins could be denatured during rice syrup processing to form aggregates, but were relatively more stable than rice endosperm protein and soy protein isolate. The main amino acids in rice dreg protein and rice protein isolate were Glu, Pro, Arg, Asp, and Leu, with Lys as the lowest content. Most of essential amino acids and nutritional parameters of rice protein isolate and rice dreg protein met the suggested nutritional requirements for a child according to FAO/WHO, and were relatively higher than those of soy protein isolate. In addition, rice protein isolate showed better digestibility than soy protein isolate during four hours sequential pepsin and pancreatin digestions. The final digestibility value was 96.66% for rice protein isolate compared to 91.41% for soy protein isolate. Thus rice dreg protein could potentially replace soy proteins as a good source of value-added protein for human nutrition in response to the increasing demand for plant proteins.
INTRODUCTION
Plant proteins provide nearly 65% of the protein supply for humans worldwide, and are valuable ingredients in many food and pharmaceutical applications. Higher demand for plant proteins has been attributed to the increase in global population, higher living costs, a limited supply of animal proteins, and increasing concerns for human nutrition.[Citation1,Citation2] In recent years, numerous studies have focused on characteristics, nutritional, and functional properties of low cost protein sources for food ingredients and nutritional supplements. Examples include sesame, soya bean, cottonseed, sunflower seed, barley, linseeds, rapeseed, rice bran, flaxseed, buckwheat, groundnut, bitter melon seed, and others.[Citation1,Citation3−Citation7]
Rice is a major cereal source of energy and protein in the world, and is consumed as a staple food in most Asian countries. Rice protein has unique nutritional and hypoallergenic properties compared to other cereals and legume proteins,[Citation8,Citation9] and is suitable as a hypoallergenic protein source to replace milk and soy infant formulas.[Citation9,Citation10] So far, the use of rice protein as food ingredient has been limited due to their lower protein content (7–9 g/100 g) compared to that of soybean (on average ≥ 40 g/100 g), lower digestibility by humans and monogastric animals,[Citation11] and poor functional properties including solubility.[Citation12]
Rice dreg protein (RDP), which is the cheap by-product of rice syrup, contains more than 50% protein content and is used as a protein source, including in protein-enriched rice flours.[Citation13] With the massive of rice starch syrups industry in China, RDP is available in large amounts and at minimal costs. In Jiangxi province of China alone, the annual production of RDP is >200,000 tons, most of which are currently used as animal feed with low economic benefits. Efforts are underway to develop efficient methods to recover proteins from RDP for human consumption that could significantly increase economic and social benefits.
A common method of extracting rice protein is by alkali extraction, followed by protein precipitation by adjusting the pH to its isoelectric point.[Citation14] Various carbohydrate-hydrolyzing enzymes, such as α-amylase and viscozyme L can be used to remove carbohydrate components in RDP to prepare the rice isolate.[Citation11] Previously it was demonstrated that protein hydrolysate of RDP could be obtained with ≥75% of protein content and less than 9% of degree of hydrolysis using trypsin as the protease by limited hydrolysis,[Citation15] showing its potential as an effective protein source to balance the diet of early-weaned babies.[Citation16] A pilot scale laboratory in Nanchang University uses enzymatic hydrolysis of RDP by trypsin with ultrafiltration membranes to produce biologically active peptides.
RDP is a desirable starting material to prepare rice protein isolate (RPI), which can be used as food ingredients to partially replace soy protein isolate (SPI) in milk, flour, and meat products. During drying, milling, and storage, heat and high-pressure treatments could induce the denaturation of rice endosperm protein,[Citation17] such that the composition and structure of the original rice protein would differ from the protein-enriched RDP from the production of rice syrup.[Citation18] To the best of the authors knowledge, there has yet to be a study done to evaluate nutritional contents and digestibility of RDP isolate (RPI) for food manufacturing. Here, the properties of RDP and RPI were characterized to investigate the possibility of using these widely available and cheap protein sources as an alternative to SPI.
MATERIALS AND METHODS
Materials
Rice dreg (RD) was provided by Jiangxi Hengtian Co. Ltd. (Jiangxi, China). It contained about 60% protein on a dry weight basis (). Raw RD was defatted twice with petroleum ether in a 5:1 ratio for 4 h, and then air-dried at ambient temperature. The defatted RD was sieved through a 100 mesh screen and stored in a refrigerator at 4°C before use. SPI (91.2% protein, on a dry-weight basis, a standard protein) was obtained from XIANGCHI Cereal and Oil Co. Ltd. (Shandong Province, China). Pepsin (from porcine gastric mucosa, catalog no. P-7000; 800–2500 units per mg of protein) and pancreatin (from porcine pancreas; catalog no. P-7545) were purchased from Sigma Chemical Co. (St. Louis, USA). All the chemicals and solvents used in the experiments were of analytical grade.
Table 1 Proximate composition of RDP and RPI
Preparation of RPI
The defatted RD (100 g) was washed three times with 1 L deionized water (50°C) and then separated by centrifugation. The residue was then re-dispersed in deionized water (1:10, w/v), and the pH of the dispersion was adjusted to 11.0 with 2 M NaOH. The resulting dispersion was gently stirred for 4 h at 40°C, and centrifuged at 5000 rpm for 15 min. This procedure was repeated to extract additional proteins from the residue. The proteins from combined supernatants from the extractions were isoelectrically precipitated at pH 4.5 with 2 M HCl and kept at 4°C for 1 h. The precipitate was recovered by centrifugation at 5000 rpm for 15 min, and then washed with deionized water. The pH value was adjusted to 7.0, before they were freeze-dried and stored at 4°C until further use.
Chemical Analysis
The moisture, protein (N × 5.95, %), starch, fiber, fat, and ash contents of RD and RPI were determined by approved AOAC methods[Citation19] with data presented in .
Differential Scanning Calorimetry (DSC)
DSC measurements were performed using a Perkin Elmer Pyris Diamond DSC (Pyris software 6.5) (Perkin Elmer, USA). Samples (5–8 mg) were weighed into aluminum pans, sealed, and heated at a rate of 10°C/min in a temperature range of 30–160°C using an empty aluminum pan as reference. To ensure good reproducibility, all experiments were conducted in triplicate.
Amino Acid Analysis
The protein isolates were hydrolyzed with 6 M HCl for 24 h at 110°C in a sealed tube. The amino acid composition, except for the tryptophan, was measured by an automatic amino acid analyzer (L-8800, Hitachi, Japan). Briefly, amino acids separated by cation-exchange chromatography were spectrophotometrically detected after the post-column reaction with ninhydrin reagent. Tryptophan content was determined after alkaline hydrolysis by a method previously reported by Landry.[Citation20] The amino acid compositions for RDP, RPI, and SPI were reported as g/100 g protein.
Evaluation of Nutritional Parameters
Essential to total amino acids ratio (E/T, %)
Protein efficiency ratio (PER)
The PER values of RDP, RPI, and SPI were calculated by the three regression equations based on the Leu, Pro, Tyr, Met, and His contents (g/100 g protein) as proposed by Alsmeyer et al.[Citation21] below.
Predicted biological values (BV)
The predicted BV values were calculated using the following regression equation.[Citation22]
Essential amino acid index (EAAI)
The EAAI was defined as the geometric mean of the egg ratio and calculated by the previously described method:[Citation23]
Amino Acid Score (AAS)
AAS is defined as the content of the limiting amino acid in the food protein, expressed as the percentage of the limiting amino acid content in a reference amino acid pattern (g/100 g protein), as recommended by FAO/WHO.[Citation24] It indicates the most limiting amino acid of the test protein compared to a reference amino acid pattern.
Chemical score (CS)
The CS was computed according to the procedure of Jood et al.[Citation23] to compare the content of EAA in a food protein to that of a whole egg protein as described by FAO/WHO,[Citation24] using the following formula:
In vitro Protein Digestibility
Digestibility of RPI and SPI was evaluated using the two-stage model system simulating gastric and small intestinal digestion with sequential pepsin and pancreatin digestion model, on the basis of the in vitro digestion method as described by Lo and Li-Chan[Citation25] and Zhu et al.[Citation26] with minor modifications. RPI or SPI solution (1% w/v, in deionized water) was adjusted to pH 2.0 with 1 M HCl, after which pepsin (2% w/w, on protein basis) was added. The solution was then incubated in a shaking bath at 37°C for 2 h. Subsequently, the pepsin-digested hydrolysate was de-activated by changing the pH to 7.5 using 1 M NaOH, followed by the addition of appropriate pancreatin (2% w/w, on initial protein basis). The digestion process continued in the 37°C shaking water bath for another 2 h. To terminate the digestion, the test tubes were submerged in a boiling water bath for 10 min. All experiments were carried out in triplicate.
SDS-PAGE analysis of digestion process
Aliquots (200 μL) of digestion mixtures were taken at 30 min interval from 0–240 min during the pepsin-pancreatin sequential digestion. These mixtures were directly mixed with the same volume of the SDS-PAGE sample buffer (with 5% v/v 2-β-ME and without 2-β-ME). SDS-PAGE analyses were conducted according to the discontinuous buffer system at 5% stacking gel and 12.5% separating gel using a Bio-Rad Mini PROTEAN® 3 system (Bio-Rad Laboratories, Hercules, CA, USA). The sample was heated for 10 min in a boiling water bath for SDS-PAGE analysis. Protein bands were stained with Coomassie Brilliant Blue R-250. Pre-stained protein molecular weight markers (10–170 kDa) were also run on the same gel to identify the molecular weight of the protein bands in the stained gels.
Nitrogen release analysis of digestion process
The Trichloroacetic acid soluble nitrogen index method[Citation4] was used with some modifications. Aliquots of digestion mixtures taken at the same time intervals as in the digestion process were directly mixed with the same volume of 15% (w/v) TCA and centrifuged at 10,000 rpm for 15 min to obtain the precipitates. The precipitates were washed with the same volume of 15% (w/v) TCA and separated by centrifugation at the same condition. The nitrogen content of precipitate and initial protein were measured by the Kjeldahl method (RPI for N × 5.95 and SPI for N × 6.25, %). The % nitrogen release was calculated using the formulae as follows:
Statistical Analysis
All analyses were performed on triplicate samples and were repeated twice. Data were analyzed by analysis of variance (ANOVA) using Origin Pro 8.0 statistics programme (OriginLab Corporation, Northampton, MA, USA) and expressed as means ± standard deviations. Statistical differences were defined as P ≤ 0.05, while the differences between the means were identified by the Tukey test.
RESULTS AND DISCUSSION
Proximate Analysis
The proximate composition of RDP and RPI are shown in . The original RDP was composed of protein (62.62 ± 0.96%), fat (10.47 ± 0.36%), and carbohydrate (9.37 ± 0.04%). By comparison, RPI had a significantly higher protein content (87.38 ± 0.31%), and significantly lower contents of other components to RDP (P < 0.05). The increase of protein content for RPI may be attributed to the process of degreasing and wash, which could reduce fat and carbohydrate contents, respectively. Starch is the major non-protein contaminant (>90%) of rice endosperm,[Citation13] while the solubility of starch syrups in water as impurities could hinder the protein extraction from RDP and could be responsible for the RPI protein content as observed here.
Thermal Property
The thermal properties of RPI could provide information about behavioral changes including denaturation, glass transition, or other reactions during starch syrups industrial processing, which could be useful for protein processing strategies. Thermal analyses of RPI and SPI were conducted using an endothermic heat scanning DSC from 30 to 160°C (; ). Denaturation temperature (Tp) is indicative of thermal stability, with higher Tp usually associated with higher thermal stability.[Citation27] Thus, the thermal stability was in the order of SPI < RPI. Higher Tp of RPI in comparison to SPI may be caused by the protein structure and amino acid composition contributing to the stability of the interior core of the proteins.[Citation28] Only a single enthalpy peak was observed for SPI, while two major peaks were noted for RPI. The two peaks of RPI could be due to different protein components with different Tp. The previously reported Tp values for albumin, globulin, prolamin, glutelin, and RPI from rice endosperm, were 46.2°C (or 73.3°C, probably due to the contaminated globulin), 78.9, 79.3, 82.2, and 81.3°C, respectively.[Citation3,Citation12,Citation17] Thus, the lower Tp for RPI may be attributed to albumin. The higher Tp was about 15°C higher than those of all fractions of rice endosperm, and also higher than the Tp of rice starch.[Citation29] This phenomenon could be attributed to the formation of starch-protein aggregates or glycosylation of RDP subunits, since starch or sugars could act as protective barriers for proteins in RDP against thermal denaturation.[Citation30] The results suggested that RPI should be more thermally stable than the protein from rice endosperm.
Table 2 Onset temperature (To), denaturation temperature (Tp), end temperature (Te), and denaturation enthalpy (ΔH) of RPI and SPI
Denaturation enthalpy (ΔH) provides information about the energy required to unfold or denature the protein structure, thus indicating the proportion of un-denatured protein in a sample, or the extent of ordered structure.[Citation27] Ju et al.,[Citation17] Paraman et al.,[Citation12] and Adebiyi et al.[Citation3] reported that the ΔH of RPI from rice endosperm was 1.79 J/g; however, ΔH values of RPI from RDP were much higher (). These data suggested that the extent of ordered structures in RDP was higher than those in rice endosperm. During starch syrups industrial processing, RDP is commonly treated at about 95–105°C, so that they may be completely denatured. However, these results clearly indicated the increase in ordered structures of RPI. A possible explanation for this phenomenon was that the industrial processing treatment probably resulted in structural rearrangement of denatured RDP through unfolding, refolding, and/or reassociation of protein, accompanied by the formation of ordered supramolecular structures. Based on their high stability, RPI could be suitable for applications in specific products requiring higher temperatures during processing.
Amino Acid Composition and Evaluation
The amino acid compositions (g/100 g of protein) of RDP, RPI, and SPI are shown in , alongside suggested EAAs requirements by FAO/WHO.[Citation24] The amino acid contents in were further used for nutritional evaluation (). The contents of all EAAs, except Lys and Thr, were higher or similar for all samples in comparison to those suggested by FAO/WHO[Citation24] as EAA requirements. shows that RDP and RPI were rich in Glu, Pro, Arg, Asp, and Leu, with the total amounts of these five amino acids of 52.93 g/100 g and 50.70 g/100 g, respectively, in agreement with previously published reports, especially for Glu and Asp,[Citation22,Citation31] and were comparable to SPI with 51.50 g/100 g. RDP had the highest percentage of hydrophobic amino acids, which could be responsible for its poor solubility in water. In comparison, the EAAs Lys, Trp, Met, and Cys, and Phe, and Tyr of RDP were to various degrees lower than those of SPI, although other EAAs were higher. Interestingly for RPI, all EAAs except for Thr, Lys, and Met and Cys were higher than those of SPI. In terms of nutritional requirement, FAO/WHO[Citation24] recommended that preschool children (2–5 years old) have protein-rich diets in nine amino acids: Thr, Val, Leu, Ile, Lys, Phe and Tyr, and Met and Cys, due to their rapid growth and immature gastrointestinal functions. All EAAs of RDP and RPI, with the exception of Lys, were close to these minimum requirements.
Table 3 Amino acid compositiona and the FAO/WHO suggested essential amino acid requirements (g/100 g of protein)
Table 4 Nutritional evaluation of RDP, RPI and SPI a according to FAO/WHO (1973).[Citation24]b,c
illustrated the protein quality parameters of RDP, RPI, and SPI. According to FAO/WHO,[Citation24] the ideal protein pattern recommends that the essential to total amino acids ratio (E/T) should be at least 36%. Both RDP and RPI scored higher in this criterion, with all E/T values being well above 36%. As expected, RPI with the ratio of 42.21% ranked the highest. The result suggested that RPI had more nutritional amino acid patterns than SPI. On the basis of AAS, the total aromatic amino acids (Phe and Tyr), Leu, and Val of all these proteins exceeded the criterion of standard protein as recommended by FAO/WHO,[Citation24] although Lys and Thr had lower levels than the standard. Lys was the first limiting amino acid of RDP, RPI, and SPI, as reported by Paraman et al.[Citation12] All EAAs of RPI, except for Lys, and the total sulfur amino acids (Met and Cys), and Thr satisfied the requirement by FAO/WHO[Citation24] for a child. On the basis of CS, it was found that the first limiting amino acid was almost similar to AAS result, with the total aromatic amino acids (Phe and Tyr) and Leu in RPI higher than the whole egg protein standard, in agreement with Padhye and Salunkhe.[Citation22]
The EAAI suggested that RDP (0.710) and RPI (0.721) had lower nutritional value than SPI (0.740). However, the PER values, ranging from 1.11 to 3.90, were much higher than the previously reported.[Citation22] For RDP and RPI, except for PER III of RPI, the values were higher than that of SPI at well above 2.00, which classified the protein as of good to high quality.[Citation32]
In vitro Digestibility
The low digestibility of a protein is one of the main drawbacks limiting its nutritional quality. Here, the digestibility of RPI and SPI under the sequential pepsin and pancreatin digestion model at different digestion time points was evaluated by reducing and non-reducing SDS-PAGE and nitrogen release analyses, as shown in and , respectively. During pepsin digestion of RPI, the subunits were rapidly hydrolyzed by pepsin after about 1 min, resulting in the production of hydrolysate with molecular weights of < 35 kDa (reduced) and < 25 kDa (unreduced). It could be distinctly observed that the molecular weight distribution of the subunits decreased with increasing incubation time, and the hydrolysate of the digestion consisted of several polypeptides in the 10 to 25 kDa range. After 60 min of pepsin digestion, polypeptides were still faintly visible, but ranged in mass from 10 to 15 kDa. The hydrolysis conditions was adjusted to pH 7.5 after 2 h of pepsin digestion, and the addition of pancreatin led to a further decline in the MW distribution of polypeptides. The 10–15 kDa subunits belonging to prolamin in RPI were still faintly present after 60 min with pancreatin. The result may be due to the poor digestibility of the prolamin in agreement with Resurreccion, et al.[Citation33] Under the same sequential pepsin and pancreatin digestion model, the protein constituents of SPI were hydrolyzed very slowly from 0 to 10 min, and no significant difference in the SDS-PAGE profiles was found after 60 min incubation time in the two digestion steps.
Figure 2 Reduced and unreduced SDS-PAGE profiles of RPI and SPI digested with sequential pepsin and pancreatin.
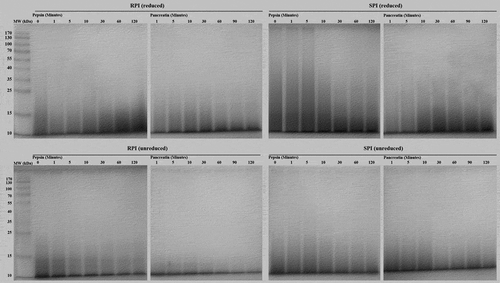
Figure 3 The nitrogen release (%) of RPI and SPI during sequential pepsin and pancreatin digestion. The error bars indicate the standard deviations of duplicate measurements.
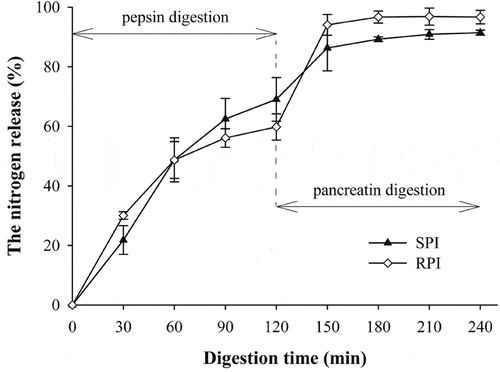
Changes in the in vitro protein digestibility of RPI and SPI during pepsin and subsequent pancreatin digestion at different time points had been well reflected by the release of TCA-soluble nitrogen. During the pepsin digestion, the percent nitrogen release for RPI increased rapidly in a linear fashion within the initial 30 min, faster than that of SPI, in agreement with the result of the previous SDS-PAGE analysis on their initial speed of hydrolysis, after which it gradually reached the maximum upon further digestion. However, the percent nitrogen release for SPI increased faster during the first 30–60 min, and then more slowly up to the maximum (although at a faster rate than that of RPI). After digestion with pepsin for 120 min, the digestibility values of RPI and SPI were 59.8 and 69.0%, respectively. The difference in the pepsin digestion pattern may be due to the differences in protein solubility and structural conformation.[Citation34] During pancreatin digestion, the percent nitrogen release for RPI increased much faster than that of SPI in the initial 30 min digestion, then increased gradually up to the maximum, with much higher increase for RPI than that of SPI during the last 30–120 min of pancreatin digestion. shows the data for RPI and SPI under non-reducing condition, indicating that most of the protein constituents were in the form of aggregates on the top of separating gel with MW of above 170 kDa. This suggested that the difference in the pancreatin digestion pattern for SPI may be attributed to the difference in size of aggregates formed from the pepsin-released peptides. On the other hand, the denaturation process of RDP could open up their structure and therefore made them less resistant to proteases, which consequently increased the accessibility of the proteins to enzymatic attack.[Citation35] Thus, the difference of pancreatin digestion pattern for RPI may be due to the denatured RPI being more susceptible to hydrolysate by pancreatin. After the two steps digestion, the final digestibility values of RPI and SPI were 96.7 and 91.4%, respectively. This outcome indicated that RPI performed better than SPI in terms of digestibility, and could be a good alternative source of protein for human nutrition.
CONCLUSION
RPI could be denatured during processing but they were more thermally stable than SPI and rice endosperm protein, as they could form starch-protein aggregates or glycosylation products. All EAAs, except for Lys, Thr, and Met and Cys in RPI and Lys and Trp in RDP, met the suggested requirements for a child’s nutrition according to FAO/WHO.[Citation24] RPI and RDP were shown to contain almost all the required nutritional parameters, while the in vitro pepsin and pancreatin digestibility of RPI was found to be better than that of SPI. Hence, this work demonstrated the potential of RDP to be utilized for human consumption as a cheaper protein source than soy proteins, which could partially replace soy proteins in some food products in response to the increasing demand for plant proteins. In addition, the utilization of this particular protein could provide a significantly added value to the by-products from rice syrup processing for higher economic and social benefits, especially in developing countries.
ACKNOWLEDGMENT
The authors gratefully acknowledge Key Program for Oil Processing and Quality Control of the Department of Science and Technology (Gankefa 2010J217), Science and Technology Platform Construction Program (2010DTZ01900), Leading Technological Innovation Team Program (Gankefa 2010J156) of Jiangxi province.
FUNDING
The authors gratefully acknowledge the financial support provided by National Natural Science Foundation of China (No. 21266020), the Programs of State Key Laboratory of Food Science and Technology of Nanchang University (SKLF-KF-201006 and SKLF-MB-201005).
REFERENCES
- Enujiugha, V.N.; Ayodele Oni, O. Evaluation of nutrients and some anti nutrients in lesser known, underutilized oilseeds. International Journal Food Science Technology 2003, 38, 525–528.
- Young, V.R.; Pellett, P.L. Plant proteins in relation to human protein and amino acid nutrition. The American Journal of Clinical Nutrition 1994, 59, 1203S–1212S.
- Adebiyi, A.P.; Adebiyi, A.O.; Hasegawa, Y.; Ogawa, T.; Muramoto, K. Isolation and characterization of protein fractions from deoiled rice bran. European Food Resource Technology 2008, 228, 391–401.
- Tang, C. Functional properties and in vitro digestibility of buckwheat protein products: Influence of processing. Journal Food Engineering 2007, 82, 568–576.
- Horax, R.; Hettiarachchy, N.; Kannan, A.; Chen, P. Protein extraction optimisation, characterisation, and functionalities of protein isolate from bitter melon (Momordica charantia) seed. Food Chemistry 2011, 124, 545–550.
- Adebowale, Y.A., Schwarzenbolz, U.; Henle, T. Protein isolates from Bambara groundnut (Voandzeia Subterranean L.): Chemical characterization and functional properties. International Journal of Food Properties 2011, 14, 758–775.
- Alu’datt, M.H.; Ereifej, K.; Abu-Zaiton, A.; Alrababah, M.; Almajwal, A.; Rababah, T.; Yang, W., Anti-oxidant anti-diabetic, and anti-hypertensive effects of extracted phenolics and hydrolyzed peptides from barley protein fractions. International Journal of Food Properties 2011, 15, 781–795.
- Eggum, B.O.; Cabrera, M.I.Z.; Juliano, B.O. Protein and lysine digestibility and protein quality of cooked Filipino rice diets and milled rice in growing rats. Plant Foods Human Nutrition 1993, 43, 163–170.
- Fiocchi, A.; Travaini, M.; D’Auria, E.; Banderali, G.; Bernardo, L.; Riva, E. Tolerance to a rice hydrolysate formula in children allergic to cow’s milk and soy. Clinical & Experimental Allergy 2003, 33, 1576–1580.
- Reche, M.; Pascual, C.; Fiandor, A.; Polanco, I.; Rivero-Urgell, M.; Chifre, R.; Johnston, S.; Martín-Esteban, M. The effect of a partially hydrolysed formula based on rice protein in the treatment of infants with cow’s milk protein allergy. Pediatric Allergy and Immunology. 2010, 21, 577–585.
- Oszvald, M.; Tömösközi, S.; Tamás, L.; Békés, F. Role of rice and added wheat protein in the mixing properties of different rice flours. Acta Alimentaria 2008, 37, 399–408.
- Paraman, I.; Hettiarachchy, N.S.; Schaefer, C.; Beck, M.I. Physicochemical properties of rice endosperm proteins extracted by chemical and enzymatic methods. Cereal Chemistry 2006, 83, 663–667.
- Shih, F.F.; Daigle, K.W. Preparation and characterization of rice protein isolates. Journal of the American Oil Chemists’ Society 2000, 77, 885–889.
- Paraman, I.; Hettiarachchy, N.S.; Schaefer, C. Preparation of rice endosperm protein isolate by alkali extraction. Cereal Chemistry 2008, 85, 76–81.
- Li, X.; Xiong, H.; Yang, K.; Peng, D.; Peng, H.; Zhao, Q. Optimization of the biological processing of rice dregs into nutritional peptides with the aid of trypsin. Journal of Food Science Technology 2012, 49, 537–546.
- Li, X.; Xiong, H.; Yang, K.; Peng, D.; Li, W.; Yin, Y.; Liu, J. Effects of rice dreg protein and its hydrolysate on growth performance and small intestine morphology of early weaned rats. Journal of Science Food Agriculture 2011, 91, 687–693.
- Ju, Z.Y.; Hettiarachchy, N.S.; Rath, N., Extraction denaturation, and hydrophobic properties of rice flour proteins. Journal of Food Science 2001, 66, 229–232.
- Shih, F.F. An update on the processing of high protein rice products. Food/Nahrung 2003, 47, 420–424.
- AOAC. Official Methods of Analysis of the Association of Official Analytical Chemists. 15th Ed. Association of Official Analytical Chemists, Washington, D.C., USA. 1990.
- Landry, J.; Delhaye, S. Simplified procedure for the determination of tryptophan of foods and feedstuffs from barytic hydrolysis. Journal of Agricultural and Food Chemistry 1992, 40, 776–779.
- Alsmeyer, R.H.; Cunningham, A.E.; Happich, M.L. Equations predict PER from amino acid analysis. Food Technology 1974, 28, 34.
- Padhye, V.; Salunkhe, D. Extraction and characterization of rice proteins. Cereal Chemistry 1979, 56, 389–393.
- Jood, S.; Kapoor, A.C.; Singh, R. Amino acid composition and chemical evaluation of protein quality of cereals as affected by insect infestation. Plant Foods Human Nutrition 1995, 48, 159–167.
- FAO/WHO. Energy and protein requirements. Report of FAO Nutritional Meeting Series, Number 52. FAO: Rome, Italy. 1973.
- Lo, W.M.Y.; Li-Chan, E.C.Y. Angiotensin I converting enzyme inhibitory peptides from in vitro pepsin-pancreatin digestion of soy protein. Journal of Agricultural and Food Chemistry 2005, 53, 3369–3376.
- Zhu, L.; Chen, J.; Tang, X.; Xiong, Y.L. Reducing, radical scavenging, and chelation properties of in vitro digests of alcalase-treated zein hydrolysate Journal of Agricultural and Food Chemistry 2008, 56, 2714–2721.
- Arntfield, S.D.; Murray, E.D. The influence of processing parameters on food protein functionality. I. Differential scanning calorimetry as an indicator of protein denaturation. Canadian Institute of Food Science and Technology Journal 1981, 14, 289–294.
- Horax, R.; Hettiarachchy, N.; Over, K.; Chen, P.; Gbur, E., Extraction fractionation, and characterization of bitter melon seed proteins. Journal of Agricultural Food Chemistry 2010, 58, 1892–1897.
- Li, Y.; Shoemaker, C.F.; Ma, J.; Luo, C.; Zhong, F. Effects of alcalase/protease N treatments on rice starch isolation and their effects on its properties. Food Chemistry 2009, 114, 821–828.
- Meng, G.T.; Ma, C.Y. Thermal properties of Phaseolus angularis (red bean) globulin. Food Chemistry 2001, 73, 453–460.
- Steenson, D.F.; Sathe, S.K. Characterization and digestibility of Basmati rice (Oryza sativa L.var. Dehraduni) storage proteins. Cereal Chemistry 1995, 72, 275–280.
- Friedman, M. Nutritional value of proteins from different food sources. A review. Journal of Agriculture and Food Chemistry 1996, 44, 6–29.
- Resurreccion, A.P.; Li, X.; Okita, T.W.; Juliano, B.O. Characterization of poorly digested protein of cooked rice protein bodies. Cereal Chemistry 1993, 70, 101–104.
- Tang, C.; Sun, X.; Yin, S.; Ma, C. Transglutaminase-induced cross-linking of vicilin-rich kidney protein isolate: Influence on the functional properties and in vitro digestibility. Food Research International 2008, 41, 941–947.
- Zhao, Q.; Xiong, H.; Selomulya, C.; Chen, X.D.; Zhong, H.; Wang, S.; Sun, W.; Zhou, Q. Enzymatic hydrolysis of rice dreg protein: Effects of enzyme type on the functional properties and antioxidant activities of recovered proteins. Food Chemistry 2012, 134, 1360–1367.