Abstract
A model for strawberry (Fragaria × ananassa cv. San Andreas) respiration rate was determined as a function of O2 and CO2 concentrations and temperature. Strawberries were enclosed in containers under different gaseous compositions (0–24% O2 and 0–15% CO2) and temperatures (10, 19, 23°C). Respiration rate was determined as O2 consumption and CO2 production. Respiration rate data was fitted to Michaelis-Menten models, with temperature dependence according to Arrhenius type equation. Non–linear regression was applied to calculate model parameters. No CO2 inhibition was verified, so a simple Michaelis–Menten model was selected (R2 = 0.91).
INTRODUCTION
Fresh fruits and vegetables packaged in modified atmosphere have experienced a significant growth in the market in recent years, given the increased consumer demand for healthier and more convenient food products and the possibility that this technology offers shelf life extension when combined with cold-temperature storage.[Citation1] Modified atmosphere packaging (MAP) effectiveness as a postharvest preservation technique is conditioned to the development of adequate atmospheres inside packages; gaseous composition and temperature should be tailored to each specific product and to its postharvest handling chain so that product quality can be retained throughout its shelf life.
MAP design is necessary for selection of suitable packaging materials for each horticultural product, on the basis of gas concentrations that will be achieved inside a package.[Citation2]
In recent years, modelling of transport and biological phenomena occurring inside MA packages has become a useful tool in MAP design, allowing prediction of package performance through the analysis of the interplay that occurs between produce respiration, O2 and CO2 permeation through packaging film, and storage temperature fluctuations.[Citation3−Citation5] Several authors working on MAP design have highlighted the importance of the availability of reliable respiration rate models for different horticultural products.[Citation2,Citation5−Citation8] Rennie and Tavoularis tested two different respiration rate models in a simulation of perforation-mediated MAP of strawberries (cv. Oso Grande) and found significant differences in resulting gas composition inside the package, reinforcing the importance of obtaining accurate respiration rate data for the given variety and conditions of the stored commodity.[Citation4,Citation5] Hertog and Banks stressed the relevance of a systematic characterization of different products’ respiration rate, as a function of at least O2, CO2, and temperature, to enable fundamental approach to MAP design.[Citation9]
Respiration rate is known to be dependent upon several factors relative to product and packaging conditions. Respiration rate models encountered in the literature take into account gaseous composition and temperature effects.[Citation10] Michaelis–Menten type equations have been used to describe respiration rate dependence on O2 and CO2 compositions,[Citation11,Citation12] and Arrhenius-type equations have been used to account for temperature influence.[Citation7]
MAP modeling and simulation as a tool for efficient MAP design for packages of strawberries under passive modified atmosphere is the focus of our current research. In particular, we are working with San Andreas strawberries because it is a cultivar that is gaining acceptance in our country due to its adaptability to different climates, which makes them available to consumers throughout a longer period of time (September–May). In fact, San Andreas strawberries are one of the most important in the Uruguaian market. Only few respiration models for strawberry are reported in the literature and we found no models for this strawberry variety. In this context, the aim of the present research was to develop a model for respiration rate of strawberry (cv. San Andreas) as a function of O2, CO2, and temperature, in order to incorporate this model in MAP simulations.
MATERIALS AND METHODS
Fruit Material and Sample Preparation
Fresh strawberries (Fragaria x ananassa cv. San Andreas), harvested at commercial maturity stage, were obtained from a local producer in Montevideo, Uruguay. Within 12 h of harvest, strawberries were transported under 13–15°C to the laboratory. Strawberries were qualitatively selected based on color, size, and absence of defects to obtain a homogeneous batch. Different strawberry batches were used for each respiration rate determination.
Experimental Design
Respiration rate of strawberries was determined in a closed system[Citation3] at three different temperatures (10, 19, and 23°C) and seven different initial gaseous compositions (0–24% O2 and 0–15% CO2; ). These gaseous compositions were chosen in order to test atmospheres to which strawberries can be exposed during storage in packages with modified atmospheres. A weighted quantity (400 ± 10 g) of strawberries was equilibrated for 2 h at the test temperature and then placed in hermetic stainless steel reactors (2.5 liter capacity) under different initial gas compositions. Reactors were stored under temperature controlled conditions (±1°C), and evolution of O2 and CO2 concentration inside them was measured. Temperature and relative humidity inside reactors were registered using iButtons (Maxim Integrated Products, Inc., CA, USA).
Table 1 RRO2 values ± standard deviation and RRO2 reduction percentage values (when temperature was decreased from 23 to 10°C) for each of the initial gaseous compositions assayed
A complete factorial experimental design was used to combine factors’ temperature and initial O2 and CO2 concentrations, so 21 batches of strawberries were used in the experiment. Since each treatment (combination of factors) was evaluated in triplicate, a total of 63 respiration rate determinations were carried out. Replicates of each treatment were allocated randomly to each strawberry batch.
Gas Composition Measurement
Monitoring of O2 and CO2 evolution was done by triplicate for a time span of 4 h, assuming that in this time the product did not age significantly and O2 and CO2 concentrations did not vary enough so as to influence respiration rate.
O2 concentration in a reactor’s headspace was determined via non-invasive measurements taken with O2 optic mini-sensors (PreSens GmbH, Regensburg, Germany). Stainless steel reactors were specially adapted for optical O2 measurement; a small acrylic window was built in the reactor’s top lid and O2 sensor spots were glued on inside the face of this window (facing inside of the chamber). On the outside face of the window, a plastic tube was adapted to receive the optic fiber cable and connect it precisely to the sensor spots. Data acquisition was done automatically every 1 min by the Oxy4v2_11FB software (PreSens GmbH, Regensburg, Germany). Temperature compensation was implemented for acquired data. O2 measurements taken with O2 mini-sensors were validated with gas chromatography measurements.
CO2 concentration in a reactor’s headspace was determined with a Shimadzu GC-14B (Japan) gas chromatograph equipped with a thermal conductivity detector and an Alltech CTR1 column. Helium was used as a carrier gas. Every 40 min, a 1-ml headspace gas sample was extracted with a syringe through a rubber septum in the reactor’s top lid and injected in the chromatograph.
Respiration Rate Determination
Respiration rate was determined as consumption rate of O2 (RRO2) and CO2 production rate (RRCO2) calculated using Eqs. (1) and (2), respectively:
Respiration Rate Modeling
Nonlinear regression procedures of XLSTAT Version 2009.4.02 (Addinsoft SARL) were used to fit different model equations to respiration rate data.
Fitting accuracy of the different models was evaluated through analysis of coefficient of determination, R2. The closer the R2 value to 1, the better the adequacy of the model to describe experimental data.
Statistical Analysis
An analysis of variance (ANOVA) was performed for respiration rate data considering temperature or O2 concentration as fixed sources of variation. When the effects were significant, mean values were calculated. Significant differences between treatments were calculated using the LSD Fisher test. Differences were considered significant when p ≤ 0.05.
Model Validation
To validate the kinetic model developed for strawberry respiration rate, a simulation of strawberry storage in modified atmosphere packaging was run and simulation results were compared to experimental results. A weighted mass of strawberries (200 ± 10 g) was conditioned in rigid polypropylene (PP) trays (19 × 26 × 5 cm) and packaged under normal air composition in 30 μm polyethylene film (20 × 30 cm; PO2 = 1900 cm3/m2d at 0% R.H. and 23°C; PCO2 = 4300 cm3/m2d at 0% R.H. and 23°C; PH2O = 5 g/m2d at 100% R.H. and 37.8°C) using a Supervac GK105/1 packaging machine (Wien, Austria). Packages were stored for 80 h in increasing temperature conditions: 2 d at 4°C, 1 d at 10°C, and 8 h at 20°C, in order to test strawberry respiration model at different temperatures. Strawberry packages were analyzed periodically in triplicate and O2 and CO2 concentrations inside packages were determined using a Servomex 1450C Food Package Analyzer (Crowborough, Sussex, UK).
Mass balances for O2 and CO2 were applied to describe O2 and CO2 evolution inside packages. Equations describing O2 and CO2 evolution were as follows:
Model predictions for O2 and CO2 evolution inside packages were calculated with Eqs. (4) and (5) and were compared to experimental data obtained for O2 and CO2 headspace concentration evolution. Differences between simulated (simul) and experimental (exp) results for O2 and CO2 for the n sampling points, were evaluated by means of root mean square error (RMSE), calculated by the following equation:[Citation13]
Equations (4) and (5) were solved numerically by an explicit Runga-Kutta algorithm as performed by MATLAB software (Mathworks, Nattick, MA, USA).
RESULTS AND DISCUSSION
O2 and CO2 Concentration Influence on Respiration Rate
Experimental RRO2 values for different O2 and CO2 concentrations at temperatures assayed are shown in . Increasing both temperature and O2 concentrations resulted in higher respiration rates (). This responds to a well-known relationship between temperature, O2 concentrations, and plant metabolism.[Citation14] Increased temperature accelerates metabolic reactions, thus hastening produce respiration, while high O2 concentrations accelerate O2 uptake because respiration enzymes are exposed to greater levels of reaction substrate.
Table 2 Analysis of variance table for respiration rate (RRO2) at 10, 19, and 23°C
Figure 1 Experimental O2 consumption rate (RRO2) as a function of O2 concentration, for the three temperatures assayed. Vertical bars represent standard deviation.
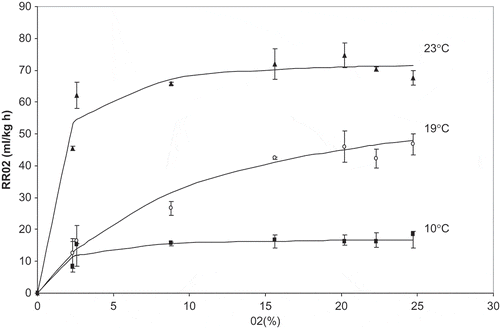
An analysis of variance (ANOVA) was performed to compare respiration rate data under each initial gaseous composition, for each of the temperatures assayed (Table 2). Significant differences (p < 0.05) were found between RRO2 values for the different O2 concentrations applied to strawberries at 19 and 23°C, ranging from 12.6 to 74.7 ml kg−1 h−1. At 10°C, the differences between RRO2 values were of the order of the experimental error and LSD Fisher test could not discriminate between them.
The highest RRO2 values were observed, at all temperatures, when O2 concentrations were high (15–25%), regardless of CO2 concentrations (). This showed that O2 concentration had a higher influence on respiratory metabolism than CO2 inhibition, because CO2 concentrations as high as 11% could not inhibit O2 consumption when O2 concentration was in the 15–25% range. This absence of inhibition of CO2 on O2 consumption at high O2 concentrations was also observed by other authors for fruits, such as pear[Citation6] and apples.[Citation8] Other fruits and vegetables presented CO2 inhibition on respiration kinetics.[Citation10,Citation15−Citation18] For the particular case of strawberry, Li and Kader[Citation19] and Talasila et al.[Citation20] reported CO2 inhibition on O2 consumption. Strawberry behavior is known to be very dependent on variety,[Citation21Citation−Citation23] so lack of inhibition observed for San Andreas strawberry could be a distinct characteristic of this variety.
At 23 and 10°C, RRO2 showed its lowest values when O2 concentration was low and CO2 concentration was high. At 19°C, when O2 was reduced to the lowest levels (2.3–2.6%), RRO2 was the same in the presence of 5.3 or 12.5% CO2 (p > 0.05). ANOVA showed that differences in respiration rates between different initial O2 and CO2 concentrations were accentuated with increasing temperature (data not shown). This could be showing that when strawberries have a higher metabolic rate, they respond more noticeably to changes in surrounding atmosphere, whereas when they have a reduced metabolism, external changes in gaseous composition exert a lower effect on respiration rate. This is a clear reason why maintaining a low temperature for fresh produce storage is so important; not only does it reduce a product’s metabolic rate, it could protect it against atmosphere modification effects. This effect was also reported by Fonseca et al.[Citation15] for Galega kale, where higher reductions in RRO2 were obtained at 20°C than at 1°C, when changing gaseous composition from air to 1% O2 and 20% CO2. Iqbal et al.[Citation17] observed that for mushrooms, a decrease in RRO2 due to low O2 and high CO2 atmospheres was 47% at 4°C and 56% at 20°C. Emond et al.[Citation24] and Li et al.[Citation14] also reported this higher sensitivity to external atmosphere composition at elevated temperatures. In the present study, O2 concentration decreases from its highest to its lowest value resulting in a reduction percentage in RRO2 of 55% at 10°C and 73% at 19°C.
Temperature Influence on Respiration Rate
As expected, temperature had a very significant influence on respiration rate of strawberry. Strawberries exposed to all initial gaseous compositions assayed showed increased respiration rate with temperature (). This is in accordance to the general knowledge that temperature is a major factor in metabolic reaction acceleration. An ANOVA was carried out on respiration rate data for temperatures assayed, for the different gaseous mixtures (). Significant differences (p < 0.05) were found between RRO2 values for the temperatures assayed in almost all gaseous mixtures applied. Lack of difference in low O2 mixtures between 10 and 19°C could be due to high dispersion in RRO2 data, due to difficulties in experimental determinations at such low O2 concentrations.
RRO2 reduction percentages when temperature decreased from 23 to 10°C are shown in . For all mixtures assayed at these temperatures, reduction percentages in RRO2 were in the 72–82% range. These values were considerably higher than reduction percentages obtained when O2 was decreased from 20–25% to 2% at 10 and 23°C. Reduction percentages obtained in this study were similar to those reported for other products, such as apples,[Citation10] Galega kale,[Citation15] and shredded carrots.[Citation16]
RRO2 Model–Combined Influence of O2, CO2, and Temperature on Respiration Rate
Different model equations, corresponding to Michaelis–Menten models for enzymatic reactions, were tested on respiration rate data for O2 consumption of strawberries. Temperature influence was accounted for by modeling the maximum respiration rate according to an Arrhenius type equation, while Michaelis–Menten and inhibition constants were considered to exhibit no temperature dependence. This last assumption was based on the analysis done by Hertog et al.,[Citation7] who theorized that since Michaelis–Menten constants are derived from kinetic constants relative to substrate and inhibitor union with enzyme complex, a similar effect of temperature on each kinetic constant can be assumed, and therefore temperature effects on Michaelis–Menten constants are cancelled.
Combined Michaelis–Menten–Arrhenius models tested in this study are shown in (Eqs. 7 to 11).[Citation10,Citation12,Citation18] In these equations, A is a pre-exponential factor (ml kg−1 h−1) corresponding to respiration rate when temperature tends to ∞; Ea stands for respiration activation energy (J mol−1); R is the ideal gas constant (J mol−1 K−1); T is system temperature (K); Km (%) is the Michaelis–Menten constant, corresponding to the O2 percentage at which respiration rate is equal to half the maximum respiration rate; and Ki (%), Kj (%), and Kn (%) are competitive, uncompetitive, and non-competitive inhibition constants, respectively. Inhibition constants’ values are specific for each product, variety, and physiological state.[Citation25] Models I to V are theoretical-based, meaning that calculated parameters can be related to physiological processes.[Citation18] Even though the respiration process in fresh produce is more complicated than only one limiting enzyme reaction with O2 as a substrate, we found these models to be more appropriate than empirical best-fitted models, because they can be related to biological phenomena. shows estimated model parameters and coefficients of determination (R2) for each model.
Table 3 Models tested on respiration rate data
Table 4 Model parameters for five combined Michaelis-Menten–Arrhenius models
Coefficients of determination (R2) obtained for all five models tested showed acceptable fit to experimental data (R2 = 0.9). A simple Michaelis–Menten model with no CO2 inhibition on O2 consumption was selected to describe strawberry respiration rate because it was the simplest of the models tested. Selection of this model was in accordance to experimental observations already mentioned in this article, where CO2 concentrations as high as 11% did not inhibit O2 consumption when O2 concentration was high (20–25%). CO2-enriched atmospheres (10–15%) are used to extend postharvest life of strawberries.[Citation19] The effect of high CO2 atmospheres on strawberry quality is reported to be related to inhibition of ripening-associated changes, such as red color development and darkening,[Citation26] to reduced incidence and severity of decay,[Citation27] and to enhancement of fruit firmness through precipitation of soluble pectins in fruit cells.[Citation28]
Models II to V showed very high values for inhibition constants Ki, Kj, and Kn. When inhibition constants take on such high values, the inhibition term (1 + (cCO2/Kx)) in Eqs. (8) to (11) tends to 1, and all equations resemble Eq. (7), corresponding to a model with no CO2 inhibition on O2 consumption.[Citation29] A similar appreciation was made by Gomes et al.[Citation6] in their study of fresh-cut pear.
Earlier modeling works regarding strawberry considered exponential and polynomial[Citation20] and competitive–uncompetitive inhibition models.[Citation21] According to Fonseca et al.,[Citation3] fit quality of exponential and polynomial models was not good. The competitive–uncompetitive inhibition model considered by Hertog et al.[Citation21] for O2 consumption by ‘Elsanta’ strawberry presented infinite values for competitive inhibition constant and uncompetitive inhibition constant, thus behaving as a simple no-inhibition Michaelis–Menten model, as in our study. Michaelis–Menten constant for ‘San Andreas’ strawberries was 1.9%, whereas this value for ‘Elsanta’ was 2.63%. Differences in Km are probably due to varietal variations in strawberry, but it ultimately refers to an affinity between O2 and respiration enzymes: the lower the Km, the higher the affinity. In this work, inhibition constants were considered as independent of temperature, and all temperature influence was attributed to maximum respiration rate. The hypothesis for this assumption is that the activation energies of the reactions involved in O2 consumption and CO2 inhibition were of the same magnitude.[Citation7]
Arrhenius-type temperature dependence of maximum RRO2 yielded activation energy (Ea) of 64.2 kJ mol−1 for ‘San Andreas’ strawberry respiration. ‘Elsanta’ strawberries evaluated in the work by Hertog et al.[Citation21] exhibited an Ea value of 74.8 kJ mol−1. Ea values for fruits and vegetables lie between 29–93 kJ mol−1, according to the work by Exama et al.[Citation30]
shows fitted data for Model I. The graph clearly shows the influence of both O2 concentration and temperature on respiration rate: reduced metabolic state of strawberries can be achieved by lowering both temperature and O2 concentration. At the initial gaseous mixtures assayed, anaerobic respiration was not established (RQ between 0.5 and 1.3; data not shown). This information could be useful for MAP design: atmospheres which yield lower metabolic rate without establishment of anaerobic respiration should be pursued inside modified atmosphere packages for shelf life extension of strawberries.
Model Validation
shows experimental and predicted O2 and CO2 concentrations throughout 80 h storage time for packaged strawberries in increasing temperature conditions. Increasing temperature conditions resulted in significant O2 reduction (<10%) and CO2 accumulation (12%). In this study, increasing temperature conditions were imposed on strawberries to test respiration model at different temperatures in a short time span. A respiration model adequately described strawberry respiration throughout 80 h storage at 4, 10, and 20°C: RMSE values obtained for O2 and CO2 curves were 1.2 and 0.68, respectively, all of them being lower than 2. RMSE values lower than or close to 2 validate accuracy of the modified atmosphere packaging model, according to Yang and Chinnan.[Citation13] In this way, the kinetic model developed for strawberry respiration rate was validated for San Andreas strawberries.
CONCLUSIONS
As expected, both temperature and atmosphere gaseous composition (O2 and CO2 concentrations) influenced respiration rate of strawberry, cv. San Andreas, measured as O2 consumption per unit mass of product. Temperature had a higher impact on respiration rate than gaseous concentration, with a 72–82% decrease in respiration rate when temperature was reduced from 23 to 10°C for all gaseous mixtures studied. Higher O2 concentrations increased respiration rate at all temperatures, regardless of CO2 concentrations. No evidence of CO2 inhibition on respiration was registered in this study at high O2 concentrations. A simple Michaelis–Menten model, with maximum respiration rate varying with temperature according to Arrhenius type equation, adequately fitted respiration rate data (R2 = 0.91) and could be incorporated in MAP simulations to design appropriate passive modified atmosphere packages for ‘San Andreas’ strawberries.
ACKNOWLEDGMENTS
The authors wish to thank Dr. Gastón Ares for his help with statistical analysis.
FUNDING
The authors are indebted to CSIC (Comisión Sectorial de Investigación Científica, Uruguay) and Comisión Administradora del Mercado Modelo (Intendencia Municipal de Montevideo) for financial support.
REFERENCES
- Day, B.P.F. Novel MAP applications for fresh-prepared produce. In: Novel Food Packaging Techniques; Ahvenainen, R.; Ed.; CRC Press: Boca Ratón, FL, 2003; 189.
- Robertson, G.L. Packaging of horticultural produce. In: Food Packaging. Principles and Practice; CRC Press: Boca Ratón, FL, 2006; 372.
- Fonseca, S.C.; Oliveira, F.A.R.; Brecha, J.K. Modelling respiration rate of fresh fruits and vegetables for modified atmosphere packages: A review. Journal of Food Engineering 2002, 52, 99–119.
- Rennie, T.J.; Tavoularis, S. Perforation–mediated modified atmosphere packaging. Part I. Development of a mathematical model. Postharvest Biology and Technology 2009, 51, 1–9.
- Rennie, T.J.; Tavoularis, S. Perforation–mediated modified atmosphere packaging. Part II. Implementation and numerical solution of a mathematical model. Postharvest Biology and Technology 2009, 51, 10–20.
- Gomes, M.H.; Beaudry, R.M.; Almeida, D.P.F.; Malata, F.X. Modelling respiration of packaged fresh-cut ‘Rocha’ pear as affected by oxygen concentration and temperature. Journal of Food Engineering 2010, 96, 74–79.
- Hertog, M.L.A.T.M.; Peppelenbos, H.W.; Evelo, R.G.; Tijskens, L.M.M. A dynamic and generic model of gas exchange of respiring produce: The effects of oxygen, carbon dioxide and temperature. Postharvest Biology and Technology 1998, 14, 335–349.
- Torrieri, E.; Cavella, S.; Masi, P. Modelling the respiration rate of fresh-cut Annurca apples to develop modified atmosphere packaging. International Journal of Food Science and Technology 2009, 44, 890–899.
- Hertog, M.L.A.T.M.; Banks, N.H. Improving MAP through conceptual models. In: Novel Food Packaging Techniques; Ahvenainen, R.; Ed.; CRC Press: Boca Ratón, FL, 2003; 356.
- Torrieri, E.; Perone, N.; Cavella, S.; Masi, P. Modelling the respiration rate of minimally processed broccoli (Brassica rapa var. sylvestris) for modified atmosphere package design. International Journal of Food Science and Technology 2010, 45, 2186–2193.
- Lee, D.S.; Haggar, P.E.; Lee, J.; Yam, K.L. Model for fresh produce respiration in modified atmospheres based on principles of enzyme kinetics. Journal of Food Science 1991, 56,1580–1585.
- Peppelenbos, H.W.; van’t Leven, J. Evaluation of four types of inhibition for modelling the influence of carbon dioxide on oxygen consumption of fruits and vegetables. Postharvest Biology and Technology 1996, 7, 27–40.
- Yang, C.C.; Chinnan, M.S. Modelling the effect of O2 and CO2 on respiration and quality of stored tomatoes. Transactions of the American Society of Agricultural Engineers 1988, 31,920–925.
- Li, X.; Wang, X.; Wang, J.; Liu, Z. Respiration rate models of Agaricus bisporus under modified atmosphere packaging. International Journal of Food Engineering 2009, 5 (4), 1–13.
- Fonseca, S.C.; Oliveira, F.A.R.; Frias, J.M.; Brecht, J.K.; Chau, K.V. Modelling respiration rate of shredded Galega kale for development of modified atmosphere packaging. Journal of Food Engineering 2002, 54, 299–307.
- Iqbal, T.; Rodrigues, F.A.S.; Mahajan, P.V.; Kerry, J.P. Mathematical modelling of the influence of temperature and gas composition on the respiration rate of shredded carrots. Journal of Food Engineering 2009, 91, 325–332.
- Iqbal, T.; Rodrigues, F.A.S.; Mahajan, P.V.; Kerry, J.P. Mathematical modelling of O2 consumption and CO2 production rates of whole mushrooms accounting for the effect of temperature and gas composition. International Journal of Food Science and Technology 2009, 44, 1408–1414.
- Saenmuang, S.; Al-Haq, M.I.; Samarakoon, H.C.; Makino, Y.; Kawagoe, Y.; Oshita, S. Evaluation of models for spinach respiratory metabolism under low oxygen atmospheres. Food and Bioprocess Technology 2012, 5, 1950–1962.
- Li, C.; Kader, A.A. Residual effects of controlled atmospheres on postharvest physiology and quality of strawberries. Journal of the American Society for Horticultural Science 1989, 114, 629–634.
- Talasila, P.C.; Chau, K.V.; Brecht, J.K. Effects of gas concentrations and temperature on O2 consumption of strawberries. Transactions of the American Society of Agricultural Engineers 1992, 35, 221–224.
- Hertog, M.L.A.T.M.; Boerrigter, H.A.M.; van der Boogaard, G.J.P.M.; Tijskens, L.M.M.; van Schaik, A.C.R. Predicting keeping quality of strawberries (cv. ‘Elsanta’) packed under modified atmospheres: An integrated model approach. Postharvest Biology and Technology 1999, 15,1–12.
- Deewatthanawong, R.; Nock, J.F.; Watkins, C.B. γ-Aminobutyric acid (GABA) accumulation in four strawberry cultivars in response to elevated CO2 storage. Postharvest Biology and Technology 2010, 57, 92–96.
- Watkins, C.B.; Manzano-Mendez, J.E.; Nock, J.F.; Zhang, J.; Maloney, K.E. Cultivar variation in response of strawberry fruit to high carbon dioxide treatments. Journal of the Science of Food and Agriculture 1999, 79, 886–890.
- Emond, J.P.; Chau, K.V.; Brecht, J.K. Modeling respiration rates of blueberry in a perforation-generated modified atmosphere package. In: Proceedings of the 6th International Controlled Atmosphere Research Conference; Blanpied, G.D.; Barstch, J.A.; Hicks, J.R.; Eds.; Ithaca, New York, 1993; 134–144.
- Cefola, M.; Calabrese, N.; Carito, A.; Vanadia, S.; Pace, B. Fruits and vegetables passive refrigerated transport: Modelling the respiratory process. Acta Horticulturae 2010, 858, 485–488.
- Shin, Y.; Ryu, J.A.; Liu, R.H.; Nock, J.F.; Polar-Cabrera, K.; Watkins, C.B. Fruit quality, antioxidant contents and activity, and antiproliferative activity of strawberry fruit stored in elevated CO2 atmospheres. Journal of Food Science 2008, 73, 339–344.
- Allende, A.; Marín, A.; Buendía, B.; Tomás-Barberán, F.; Gil, M.I. Impact of combined postharvest treatments (UV-C light, gaseous O3, superatmospheric O2 and high CO2) on health promoting compounds and shelf-life of strawberries. Postharvest Biology and Technology 2007, 46, 201–211.
- Harker, F.G.; Elgar, H.J.; Watkins, C.B.; Jackson, P.J.; Hallet, I.C. Physical and mechanical changes in strawberry fruit after high carbon dioxide treatments. Postharvest Biology and Technology 2000, 19, 139–146.
- Ho, Q.T.; Verboven, P.; Verlinden, B.E.; Lammertyn, J.; Vandewalle, S.; Nicolaï, B.M. A continuum model for metabolic gas exchange in pear fruit. PLoS Computational Biology 2008, 4, 1–13.
- Exama, J.P.; Arul, J.; Lencki, R.W.; Lee, L.Z.; Toupin, C. Suitability of plastic films for modified atmosphere packaging of fruits and vegetables. Journal of Food Science 1993, 58, 1365–1370.