Abstract
Foods may contain more than one type of protein, and food formulators sometimes combine different proteins for desired synergistic textural benefits. Egg albumin, fish protein isolate, or soy protein isolate were blended with calcium caseinate or whey protein isolate and mixed in water adjusted to pH 2.5, 6.8, and 9.0 at 25 or 60°C. The effect of pH and temperature on solubility, viscosity, and the structure of the resulting gels were determined. The viscosity at the most soluble concentration at 25°C were: egg albumin (175.2 mPa.s/35 wt%), fish protein isolate (2207.4 mPa.s/30 wt%), soy protein isolate (2531.5 mPa.s/10 wt%), calcium caseinate (1115.8 mPa.s/15 wt%), and whey protein isolate (161.2 mPa.s/35%). In mixed protein systems viscosity values were reduced. The values for calcium caseinate or whey protein isolate with egg albumin, at the protein level of 15 g/100 g were: calcium caseinate/egg albumin (10:5 wt%) 535.1 mPa.s and whey protein isolate/egg albumin (10:5 wt%) 8.7 mPa.s. Microscopy imaging revealed changes in protein aggregation clusters during heating of calcium caseinate, egg albumin, and whey protein isolate. Egg albumin acted synergistically to increase viscosity, while fish protein isolate acted antagonistically to reduce viscosity. This knowledge is useful to manufacturers who may seek to enhance food texture by blending different proteins.
INTRODUCTION
Proteins are essential food materials, and their complex interactive sub-structures play key roles in food functionality through the types of linkages formed by their highly charged amino acid building blocks. The microstructure and surface forces from the polarity of amino acid chains may affect protein solubility; polar and nonpolar functional groups have a tendency to form interfaces between hydrophilic and hydrophobic molecules in mixed protein systems.[Citation1] In the bulk phase, proteins properties depend on the extent of their solubility in a given medium.[Citation2]
Protein solubility is also affected by the shape of the molecule, the state of denaturation, concentration, and other factors in its domain, such as temperature, pH, and the presence of other molecules.[Citation2,Citation3] Proteins act in different ways in food systems depending on the conditions present, affecting product quality characteristics. The concentration of casein micelles and soy protein affected the miscibility or ability to form stable emulsions in a mixed system resulting in unstable thermodynamic gels.[Citation4] Thermodynamic incompatibility resulting in phase separation is typical with mixed proteins from different sources (casein and soy globulins), or in different states (native or denatured), or in low or high concentration.[Citation5]
Dumetz et al.[Citation6] and Fitzsimons et al.[Citation7] have shown that protein phase behavior in aqueous solution may exist in two states: associative interactions that may form strong aggregate gel networks or dissociative interactions resulting in destabilized or phase separate systems. Popular foods, such as yogurt, luncheon meats, and seafood analogs, are formulated with proteins for enhanced binding and textural properties.[Citation8] When mixed with nonprotein materials, starches and food gums, proteins can be used to enrich the nutritional content foods.[Citation9,Citation10] Textured protein foods are formed by synergistic interactions of proteins with polysaccharides induced by chemical cross-linking or covalent physical entanglements.[Citation7,Citation11] Glibowski[Citation12] showed that inulin and whey proteins formed strong gel networks with significantly increased viscoelastic properties at low concentration of whey proteins (<7%).
Combining different proteins under different conditions of pH or enzyme treatment, and/or varying the concentration ratios may produce strikingly new behavior upon heating.[Citation13] When casein and whey proteins were combined and heated, they formed strong aggregate gels as in yogurt.[Citation14] Some studies on the mixing of whey proteins (α-lactalbumin, β-lactoglobulin, and bovine serum albumin) resulted in a synergistic interaction and co-aggregation forming irreversible networks.[Citation15,Citation16] Other research on whey proteins suggested nonspecific mixed network and rheological properties depending on total protein concentration, and type of proteins mixed.[Citation17] Whey protein isolate solutions with less than 10% solids behaved like Newtonian fluids, and concentrations up to 50% behaved like non-Newtonian fluids.[Citation10]
In a mixed soy protein and micellar casein system heated to 90°C, concentrations in the range of 7.5 to 12.5% exhibited phase separation, low viscosity, and low yield stress, while concentrations greater than 15% showed protein aggregation and incipient gel network formation.[Citation4] In a casein/soy protein system (1:1) ranging from 2 to 15 wt% concentration, limited miscibility resulted in a weak shear thinning phase-separated network due to negatively charged proteins.[Citation4]
Strong protein networks may be formed by combining different types of proteins, for example, egg albumin and whey protein isolate. Ngarize et al.[Citation8] showed a strong synergistic interaction for a 15 wt% or higher concentration of an egg albumin/whey protein isolate mixture, postulating that the protein network was strengthened by hydrogen bonding, hydrophobic effect, and changes in disulfide bonds. Mixed protein systems containing high concentrations of proteins (>20%) are affected by macroscopic and molecular level interactions, which depend on their respective solubility; for example, the viscosity of whey protein concentrations of 40 wt% or higher changed from time-independent to time-dependent shear-thinning.[Citation18] It has been reported that the relative concentration of a casein/pectin gel system affected the morphology of the gelation process because of the relative kinetics of phase separation.[Citation19]
Solubility of food proteins depends on their state, native or denatured, pH, and process temperature. Solubility is a very important factor affecting other protein functional properties, such as emulsion, gelation, and viscosity.[Citation20,Citation21] Solubility relates to surface hydrophobicity resulting from protein to protein or protein to water interactions in food.[Citation2] Classical methods of quantifying the solubility of food proteins have relied on determining the insoluble portion in water at pH 7.0 as predictors of surface hydrophobicity properties.[Citation22] Other methods, such as turbidity measurements between 600 and 800 nm wavelengths, were reported for determining casein micelle and whey protein dispersions; transitions were observed at the point of denaturation, where values increased sharply with aggregation.[Citation23] The purpose of this research was to determine the effect of temperature and pH on the solubility of individual or mixed food proteins, and to evaluate their viscosity and microstructural properties.
MATERIALS AND METHODS
The proteins used for the study were spray dried egg albumin (EA) from (Primera Foods Corp., Cameron, WI, USA), fish protein isolate (FPI) from (Federal Laboratories Corporation, Alden, NY, USA), soy protein isolate (SPI) from (Pro-Fam® 974, ADM Specialty Food Ingredients Division, Decatur, IL, USA), calcium caseinate (CC) purchased from (American Casein Company, Burlington, NJ, USA), and whey protein isolate (WPI) from (Provon® 190, Glanbia Nutritionals, Inc., Monroe, WI, USA). The proximate compositions of the protein materials specified by the manufacturers are presented in .
Table 1 Proximate composition of calcium caseinate (CC), whey protein isolate (WPI), egg albumin (EA), fish protein isolate (FPI), and soy protein isolate (SPI)
Protein Solubility
The solubility of food proteins was determined by modifying methods as described in Kilara and Mangino[Citation23] and Kilara[Citation24] in the following way. The point of highest solubility for each protein or proteins blend treated at 25 or 60°C, and pH 2.5, 6.8, and 9.0 were determined. Varying concentrations (0.5 to 50 wt%) of the individual proteins were prepared with the appropriate amount of deionized water (adjusted to pH 2.5, 6.8, or 9.0 with 0.1 N Hcl or 1 N NaOH) in centrifuge bottles held at 25 or 60°C. The bottles were shaken vigorously, then placed in an orbital shaker at 3000 rpm and kept at 25 or 60°C for 2 h. After shaking, aggregated proteins were centrifuged for 40 min at 12,000 rpm; the supernatant was decanted, weighed, and dried overnight in a Model #29 Precision vacuum oven (Winchester, VA, USA) set to 105°C. The solids content in the decanted liquid was used to calculate the solubility of the protein following Kilara[Citation24] as solids content in the dried decanted supernatant divided by sample weight and multiplied by one hundred.[Citation25]
Light Transmission
Solubility was further determined by transmission colorimetric spectrophotometry estimation as described below.[Citation26] The ColorQuest XE (HunterLabs, Inc., Reston, VA, USA) diode array xenon flash colorimeter/spectrophotometer with a wavelength range from 400 to 750 nm that reads both reflectance and transmittance was used. The spectral patterns of the supernatant from various concentrations of EA, FPI, SPI, CC, or WPI were measured with the colorimeter/spectrophotometer through a 10-mm aperture in a 20-ml transmission cell. The sample was then scanned in the transmittance (TTRAN) mode under the following settings: the large reflectance port was set to (1.00[Citation1]); Illuminant at D65 (MI Illuminant Fcw), and observer 10°. Triplicate readings were taken following Davies et al.[Citation26] An increase in soluble material corresponded to a decrease in % transmission values (increase in absorption of light), particularly at specific wavelengths between 400 and 700 nm (data not shown); specifically, the amount of light absorbed at 480 nm changed as the protein concentration changed. Therefore, for analyses the widest separation of proteins at 480 nm wavelengths was used. All tri-stimulus integrations are based on a triangular band-pass of 10 nm and a wavelength interval of 10 nm. The instrument sensor uses a specially coated plastic integrating sphere (diameter of 6 in.) that diffuses the light from the xenon light source. The light source illuminates and is transmitted through the sample. A lens located at an angle of 8° perpendicular to the sample surface collects the transmitted light and directs it to a diffraction grating that separates the light into its component wavelengths. The intensities of these component wavelengths of transmitted light are then measured by 2 polychromators each with 40-element diode array detectors. The instrument was standardized as per company specifications and blanked on an empty cuvette using the method described by Davies et al.[Citation26]
Viscosity Measurements
After determining the optimum solubility of proteins adjusted to pH 2.5, 6.8, or 9.0 at a given concentration, the proteins were combined in ratios with water, based on the optimal soluble concentration for the less soluble of the two proteins. The mixture was placed into a 25 or 60°C water bath and blended for 15 min with a high speed mixer at 1800 rpm; immediately after, approximately 60 mL of the specimen were poured into a thermosel system with a UL spindle attached to a DV-III Ultra Brookfield Viscometer (Middleboro, MA, USA). Viscosity was measured at 100 rpm. All apparent mean viscosity values of three specimens at 100 rpm were shown in –.
Table 2 The effect of temperature and pH on solubility of proteins at near-optimal concentration for calcium caseinate (CC), whey protein isolate (WPI), egg albumin (EA), fish protein isolate (FPI), and soy protein isolate (SPI)
Table 3 The effect of temperature and pH on solubility and viscosity and conductivity at optimal concentration for calcium caseinate (CC), whey protein isolate (WPI), egg albumin (EA), fish protein isolate (FPI), and soy protein isolate (SPI)
Scanning Electron Microscopy (SEM)
Concentrated protein solutions (16–22 wt%) and the blended protein mixtures were prepared at 25 or 60°C and placed in dialysis membrane tubing (Spectrum Laboratories, Rancho Dominguez, CA, USA) and equilibrated with a solution of 2.5% glutaraldehyde-0.1 M imidazole buffer at pH 7.2. The dialysis membrane was washed before use by boiling in water with a low concentration of sodium bicarbonate. After washing in the buffer solution, the protein was fixed chemically (crosslinking) by dialysis overnight, then dehydrated in a graded series of ethanol solutions (50%, 80%, and absolute), and freeze-fractured. The specimens were washed out in fixative solutions two times with 15-mL washes in the imidazole buffer (pH 7.0) over a 30 min period with agitation, dehydration in a graded series of alcohol solutions for 1 to 2 h before the usual freeze fracture. Finally, the crosslinked proteins were washed in distilled water, with a preservative and stored at 4°C to discourage microbial contamination. Fragments of the proteins were mounted on SEM sample stubs, coated with a thin layer of gold by DC sputtering and imaged with a Quanta 200 scanning electron microscope (FEI Co., Inc., Hillsboro, OR, USA), operated in the secondary electron imaging mode.
Statistical Analyses
Statistical analysis was conducted using the SAS Statistical Software version 9.2 (SAS Institute Inc., Cary, NC, USA). The effect of protein concentration (including amount of each protein and type), pH, and temperature of the single proteins, and their combinations were evaluated using the SAS procedure ‘Proc Mixed’ to determine the main effect of the variables and interactions of protein concentration, pH, and temperature on the responses. Mean separations were performed using the lsmeans option and Bonferroni adjustment to account for multiplicity. Slices were performed on the effects of each of the three factors while the other two were held constant. Regression models correlated with the main protein effect on solubility indicating the innate differences in source or type of protein. An overall correlation between the variables was computed.
RESULTS AND DISCUSSION
Protein Solubility
The effect of adding other food proteins to CC or WPI at 25 and 60°C was studied to determine optimal soluble concentration. Mixtures of CC or WPI with EA, FPI, or SPI slurries with water at 25°C were blended to make up various protein ratios. The slurries were stirred continuously for 15 min and heated to 60°C to form incipient gels. The solubility of the protein slurries in water at 25°C or gels at 60°C, both at pH 2.5, 6.8, and 9.0 were identified. Every one of the five proteins had a more specific range of solubility ranging from 5 to 45 wt% concentration; the optimal soluble concentration range for each protein was as follows: CC (15 to 25%), EA (30 to 40%), FPI (20 to 30%), SPI (5 to 10%), and WPI (30 to 40%). The values of the solubility of the five proteins near their optimal soluble concentrations at 25 and 60°C, at pH 2.5, 6.8, and 9.0 are in . Patocka et al.[10] showed that there is a definite relationship between the solubility of whey protein concentrates and their physical properties, which depended on the concentration, pH, temperature, and other factors. Also, Pelegrine and Gasparetto[Citation2] showed that the solubility of proteins, especially in mixed systems, is of primary importance because it affects all subsequent functionality, such as gelation and viscoelastic properties. Our results showed differences in solubility and concentration depending on type of protein.
The range for the solubility values for food proteins varied. WPI in concentration from 30 to 40 wt% was the most soluble at all temperatures and pH (). WPI was more moderately soluble at pH 2.5 at both 25 and 60°C. CC was more soluble at 25°C than at 60°C in concentration ranging from 15 to 25 g/100 g. CC was most soluble at 25°C (pH 2.5 and 9.0), and at 60°C (pH 6.8 and 9.0). The solubility of CC was affected more by pH than concentration. In most cases, CC held at pH 6.8 and 9.0 exhibited higher solubility except at 20 g/100 g pH 2.5. Egg albumin was higher in solubility than WPI. WPI was soluble at 25°C in concentrations up to 40 g/100 g. The pH of EA was less of a factor influencing its solubility than temperature. The solubility of EA was reduced significantly at 60°C, and decreased more when the pH was lowered to 2.5. SPI was moderately soluble at both temperatures, 25 and 60°C, in concentrations ranging from 5 to 10 g/100 g, but more soluble at pH 6.8 and 9.0. SPI was much less soluble at pH 2.5 and 60°C. FPI was the least soluble at both pH and temperature conditions studied. The overall solubility of FPI was less than 10% under all conditions. Temperature and pH affected the solubility of the five proteins studied. Others have reported similar effects of temperature and pH on solubility. Pelegrine and Moraes Santos Gomes[Citation22] reported solubility minimum at pH 5 for whey proteins in the temperature range of 40 to 90°C. Tang et al.[Citation27] showed that the concentration, temperature, and pH of whey protein concentrate solutions affected the flow and other physical functions. In dry heated protein systems (105 to 145°C), Mohammed et al.[Citation18] showed that the solubilities of heated food proteins were less than 20% in a 1% sodium docecyl sulfate plus 1% beta-mercaptoethanol, except for casein. The degree of solubility determined by Mohammed et al.[Citation18] was: whey protein, milk powder, and β-lactoglobulin is more soluble than egg albumin and bovine serum albumin, which is more soluble than soy isolate, and sodium caseinate, which is more soluble than gluten which is more soluble than gelatin. However, the solubility of unheated proteins ranged from 95 to 100%. This was similar to the pattern determined in this study.
The optimal relative solubility of the proteins was obtained at the following concentrations: EA (50 wt%), WPI (40 wt%), FPI (35 wt%), CC (20 wt%), SPI (<10%) at 25 and 60°C, and pH of 2.5, 6.8, and 9.0. The histograms of the solubility patterns for the different proteins under the temperature and pH conditions studied are shown in . The solubility of proteins at 25 or 60°C and pH 2.5, 6.8, and 9.0 were for CC (), WPI (), EA (), FPI (), and SPI (). The difference in optimal concentration reflects individual solubility pattern of the proteins at temperatures under 60°C. CC, EA, and SPI were much less soluble at 60°C than at 25°C. At 25°C, EA and WPI were more soluble (>80%) on the average across all pH conditions than the other proteins. WPI was slightly more soluble than EA at pH 6.8 and 9.0, except at pH 2.5 where solubility was only 33%. EA was more soluble at pH 2.5 and 9.0 than at pH 6.8. CC (70%) and SPI (45%) were moderately soluble at 25°C, except for CC at 25°C, pH 6.8, which was low at 36% solubility. At 60°C, WPI was the most soluble of all proteins at 82%, followed by CC (40%) and SPI (35%) at pH 6.8 and 9.0. All proteins were affected by pH conditions at 25°C. CC was least soluble at pH 6.8, but was comparatively twice as soluble at pH 2.5 and 9.0. At 60°C, CC was not soluble at pH 2.5, but was slightly more soluble at pH 6.8 and above. Egg albumin was soluble at all pH conditions at 25°C, but less so at 60°C. The pH made a difference in EA solubility pH 9.0. Fish protein isolate was mostly insoluble (<8% concentration), at all conditions of pH and temperature. Though protein solubility is a function of many factors, the state and source or type of protein is intrinsically important.[Citation20] Anandharamakrishna et al.[Citation21] suggested that denatured state of proteins is not enough to cause loss in solubility, but aggregation must also occur. de Wit and Klarenbeek[Citation28] showed that altered conformational structures due to heat affects the solubility of proteins. However, aggregation is heavily influenced by the pH, surface charges, the net charge of the system, and the electrostatic repulsive forces between the molecules.[Citation2,Citation3] Pelegrine and Gasparetto[Citation2] showed that the pH and net charge of the protein affects solubility and, consequently, other physical functions. This study also shows that the optimum solubility of proteins are affected by pH, temperature, and type of proteins.[Citation2]
Figure 1 Solubility of proteins at 25 or 60°C and pH 2.5, 6.8, and 9.0. (A) Calcium caseinate, (B) whey protein isolate, (C) egg albumin, (D) fish protein isolate, and (E) soy protein isolate.
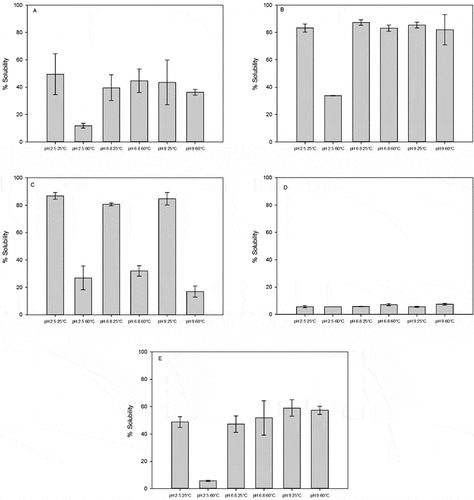
Indeed, the net charges of the five proteins and their blends varied significantly (P < 0.05) ranging from 1.03 to 11.04 mS/cm (). Protein solubilities are sensitive to salt and hydrogen ion concentration.[Citation27] Though the net charges of the proteins may produce attraction or repulsion at the same pH, as a generalized rule, proteins are more soluble in low acids or high alkali.[Citation22] Solubility and surface hydrophobicity can be used as predictors of surface properties of proteins.[Citation23] The rheological behavior of products is affected by the level of the concentration of soluble proteins; for example, the rheology of yogurt was affected by the solubility of added whey protein isolates.[Citation2]
Viscosity Measurements
Viscosity values were intrinsically different for the five proteins in response to changing temperature and pH. No significant correlation was found between the solubility of the proteins and viscosity (). High viscosity values were recorded for CC at pH 2.5 and 9.0. EA had the highest value at 60°C, pH 2.5, which was greater than 5999 mPa.s. EA over-ranged the Brookfield Viscometer (>5999 mPa.s) at pH 2.5 and 60°C. The viscosity of WPI was independent of pH at 25 and 60°C, but was slightly decreased at 60°C (pH 2.5). WPI had low viscosity values (<50 mPa.s) at all pH and temperature conditions reflecting very weak solutions. SPI over-ranged at 10 wt% at both 25 and 60°C, pH 2.5 and 9.0 (data not shown). SPI specimens were optimally soluble at the concentration of less than 8% or less; values reported at 5% and 60°C concentration were below 35 mPa.s at all conditions (). Statistically, solubility was not significantly (P > 0.05) related to the viscosity of the mixed proteins (R2 = 0.397) for all five proteins combined. Significant main effects (P < 0.01) for the individual proteins were determined for protein concentration, pH, temperature, and their interactions ().
Table 4 Statistical analyses using the mixed model procedure and analysis of variance type 3 test of fixed effects of calcium caseinate (CC), whey protein isolate (WPI), egg albumin (EA), fish protein isolate (FPI), and soy protein isolate (SPI): The effect of temperature, pH, and concentration on solubility and viscosity
CC was affected by concentration, pH, and the cross-interactions of pH and temperature, but no cross effects interaction (). EA and WPI were affected by concentration, pH, and temperature conditions, and their interaction. SPI was affected by all conditions and interactions except for the protein/temperature. In this study, significant differences were observed for FPI by concentration, pH/temperature interactions. Others have reported that the viscosity and gelling effects depend on multiple factors, such as the concentration of proteins, amount of water present, ionic strength, pH, and temperature;[Citation29] as such, it is highly unlikely that viscosity can be related strongly to only one factor. In this study the multi-factor effect on viscosity was observed. Dumetz et al.[Citation6] showed that events that produce protein gels are hard to reproduce disordered temperature-dependent events; hence, the low correlation of solubility with viscosity. Our findings also agree with those of Ren et al.[Citation30] who showed shear thinning for a soy protein/micellar casein system, while apparent yield stress increased with increasing viscosity.
Table 5 The effect of temperature and pH on viscosity and conductivity of mixed proteins, calcium caseinate (CC), whey protein isolate (WPI), egg albumin (EA), fish protein isolate (FPI), and soy protein isolate (SPI)
Mixed Protein Viscosity
At the optimal concentration of 15 g/100 g of protein, the viscosity values for calcium caseinate were: 4914.5 mPa.s (pH 2.5), 374.2 mPa.s (pH 6.8), and 3502.3 mPa.s (pH 9.0) in . As 5 g/100 g EA was substituted for CC, the viscosity of the CC/EA mixed gel was reduced significantly (P < 0.05) to 631.7 mPa.s (pH 2.5), 34.9 mPa.s (pH 6.8), and 433.5 (pH 9.0) in . However, the viscosity values of CC at 60°C (16.1 mPa.s) increased from 16.1 to approximately 58 mPa.s at pH 2.5, and from 17 to 28.0 mPa.s at pH 9.0. In the EA dominated system CC (5 g)/EA (10 g), viscosity increased significantly at 60°C, from 16.1 to 434.7 mPa.s (pH 2.5) and from 17.5 to 124.8 mPa.s at pH 9.0. Blending EA into calcium caseinate reduced the viscosity of the resulting slurry at 25°C, but increased the gel strength at 60°C. When EA dominated CC (5 g)/EA (10 g), the viscosity increased even more at 60°C. Depending on concentration and ratios in combined proteins with EA, EA/CC, for example, viscosity increased synergistically (). One fundamental parameter, which characterizes the flow behavior of liquid and semi-liquid foods, is its intrinsic resistance to motion (flow) with shear stress.[Citation31] Our findings are in agreement with Fitzsimons et al.,[Citation7] who showed that in a mixed protein system combining gelatin and whey protein isolate, unheated slurries at ambient temperature were very weak, but that increased concentration and temperature (85°C) increased gel properties.
There was a clear pH and temperature effect on the mixed CC/EA system at 25°C as well, but the only increase in viscosity was at 60°C. Blending CC (10 g) with FPI (5 g) decreased viscosity at 25°C, and even more so at 60°C, for all pH conditions (). The mixture containing higher amounts of fish protein isolate, CC (5 g)/FPI (10 g) was completely dominated by low viscosity for both temperatures and pH conditions. FPI was mostly antagonistic with other proteins. Blending soy protein isolate with CC had a similar viscosity response as with EA: significant decrease in viscosity at 25°C, but an increase in values at 60°C for the three pH conditions. The combination of CC and WPI resulted in weaker slurry systems except at 25°C (pH 9.0), which had a viscosity of 417.9 mPa.s. The rest of the blends were of extremely low viscosity ().
Thus, depending on protein concentration, pH, and temperature, mixing CC with EA or with SPI may increase viscosity synergistically, but blending CC with WPI and FPI may be antagonistic and reduce viscosity significantly. The viscosity of a heated mixed protein system may depend on the nature of the gel system formed. For instance, in a casein-whey protein blend, heterogeneous aggregates or particulates may result in low viscosity gels.[Citation24] van Vliet et al.[Citation14] showed that in unheated acid-induced milk gels from mixtures of casein and whey proteins, casein contributed to gel formation at ambient conditions, but when heated in the presence of whey proteins, particularly the β-lactoglobulin fractions, made the gel much stiffer, minimizing syneresis. van Vliet et al.[Citation14] showed that after heating the mixed slurry of CC and WPI, whey protein aggregates had a large impact on the structure and mechanical properties of the resulting gel; other impactful factors were especially the pH and temperature.
Blending other proteins with whey protein isolate changed the properties of the WPI systems at an optimal concentration of 35 g/100 g, the viscosity values were 453.1 mPa.s (25°C) and 128.6 mPa.s (60°C) at pH 2.5, 441.9 mPa.s (25°C) and 335.4 mPa.s (60°C) at pH 6.8, and 385.5 mPa.s (25°C) and 451.7 (60°C) at pH 9.0 in . The viscosity of WPI (25 g/100 g) mixed with EA (10 g/100 g) increased significantly at all temperature and pH conditions, except at 25°C (pH 2.5) in . Viscosity also increased for all pH and temperature conditions when EA (25 g/100 g) was dominant in the WPI (10 g/100 g) mixed system. The increase in viscosity of the EA predominant system was more significant at 60°C. Mixing FPI with WPI reduced the viscosity at all temperature and pH conditions; though for the FPI dominant system with WPI (5 g/100 g) and FPI (20 g/100 g), the viscosity values were higher than the WPI predominant system ().
Table 6 The effect of temperature and pH on viscosity and conductivity of mixed proteins, whey protein isolate (WPI), egg albumin (EA), fish protein isolate (FPI), and soy protein isolate (SPI): The effect of temperature and pH
The viscosity of the WPI (5 g/100 g) and SPI (5 g/100 g) mixed gel was very low. In general, blending other food proteins with WPI reduced viscosity values significantly, except for blending with EA. The interaction of WPI with other food proteins was different than those of CC with the same proteins. Adding EA to replace part of the protein increased the mixed system viscosity. In general, the viscosity of CC increased synergistically by blending with EA and SPI. In contrast, WPI increased synergistically with only EA; blending all other food proteins reduced the viscosity of WPI significantly (P < 0.05). Polyakov et al.[Citation5] showed that in general, incompatibility is common for proteins from different classes (casein or soy globulins), or different conformations (native or denatured).
High phase separation occurs when incompatible proteins are mixed in water in low concentrations (10 to 20 wt%), except when high shear processing tools are used to force the mixing, enabling entangled network formation. O’Kennedy and Kelly[Citation32] reported that heat denatured whey proteins increased gel strength, and that pH exerted a great influence on complex formation in CC even at ambient conditions, which favored increased casein aggregation. Immiscibility is an important factor that can impede interaction of mixed proteins, such as with casein micelles or soy proteins. However, miscibility depends on concentration, temperature, and molecular weight of the proteins.[Citation33,Citation34] Also, Framelart et al.[Citation35] showed similar increases in mixed β-lactoglobulin and egg albumin. Our results were similar showing increased aggregation with temperature and pH.
Campo-Deano and Tovar[Citation36] showed that blending EA at the concentration of 1.5 to 2.5% increased the gel strength of imitation crabsticks made with fish proteins at 20°C, but weakened it at 60°C. It was postulated that EA coagulated forming a solid-like network, which clumped together allowing the rest of the protein to separate into the serum.[Citation36] Also, Brenner et al.[Citation37] showed that fish protein isolate gels could form at ambient temperatures between pH 8.5 to 11, but that the strength would increase with concentration and temperature. In mixed protein systems, for example the mixture of gelatin and egg albumen, the compatibility of the two proteins increased the gelling properties.[Citation38] These results are in agreement with our findings that EA improves gel strength in some protein blends, and that pH and concentration are important factors in mixed protein systems.
Comparatively, mixing the two dairy proteins (CC and WPI) under the conditions of this study produced limited synergy on viscosity, but combined independently with EA and SPI the viscosity increased. Increasing temperature up to 60°C resulted in increased solubility and viscosity greater than in CC (25 wt%) at pH 6.8 and 9.0, EA (35 wt%) at pH 6.8 and 9.0 (), and WPI (35 wt%) at pH 9.0 (). In mixed protein systems, synergistic increases in viscosity occurred with EA (35 wt%) at 60°C by 2720 mPa.s (pH 6.8) and by 656 mPa.s at pH 9.0 (). Similar increases occurred with WPI (35 wt%) by 67 mPa.s at pH 9.0 (). In the mixed protein systems, synergies that increased gel viscosity at 60°C were CC/EA (5/10 wt%) by 162 mPa.s at pH 2.5 and 108 mPa.s at 9.0 (). Matringe et al.[Citation39] reported a synergistic increase in gelation of heated skim milk/egg albumin system, and antagonistic effect on foaming. Usually, synergies that increase network strength are formed by heat-induced aggregation.[Citation8]
The viscosity of WPI and EA mixed slurry (25°C) and incipient gel (60°C) increased with both temperature and pH conditions (). In the WPI dominated system, WPI/EA (25/10 wt%), the viscosity increased by 491 mPa.s (pH 2.5), 77 mPa.s (pH 6.8), and 203 mPa.s (pH 9.0). In the EA dominated system, WPI/EA (10/25 wt%), viscosity increased by 3181 mPa.s (pH 2.5), 455 mPa.s (pH 6.8), and by 1885 mPa.s (pH 9.0). Only a small increase in viscosity was observed with the WPI/FPI (5/20 wt%) system at 60°C by 31 mPa.s (pH 9.0). In mixed dairy protein systems, adding EA increased viscosity synergistically for CC gels at 60°C, WPI slurries at 25°C, and WPI gels at 60°C. FPI increased viscosity in WPI gels slightly at 60°C (). Combining EA and FPI, EA/FPI (20/5 wt%) increased viscosity at 60°C by 3984 mPa.s (pH 2.5), 560 mPa.s (pH 6.8), and 983 mPa.s (pH 9.0). The EA/WPI (5/5 wt%) system increased viscosity at 60°C by 342 mPa.s. EA/SPI (5/5 wt%) increased viscosity at 60°C by 851 mPa.s ().
Table 7 The effect of temperature and pH on viscosity and conductivity mixed proteins, egg albumin (EA), fish protein isolate (FPI), and soy protein isolate (SPI)
The viscosity values for egg albumin at 25°C were 285.9 mPa.s (pH 2.5), 514.8 mPa.s (pH 6.8), and 756.4 mPa.s (pH 9.0); at 60°C, the values were >5999 mPa.s (pH 2.5), 3284.6 mPa.s (pH 6.8), and 1411.7 mPa.s (pH 9.0) in . The viscosity of mixed EA (20 g/100 g) and FPI (5 g/100 g) was significantly reduced at 25°C, but was less so at 60°C (). As the concentration of FPI increased EA (5 g/100 g/FPI (20 g/100 g), all viscosity measurements were reduced significantly across all conditions. The blend that combined EA (5 g/100 g) and SPI (5 g/100 g) was significantly very low, but at pH 9.0 (25°C) and at pH 2.5 or 9.0 (60°C) was the highest. EA, a high viscosity protein by itself, decreased in viscosity values when blended with FPI and SPI.
Past research on blending milk proteins showed that the rheological properties may depend solely on protein concentration, and that the network formed from different types of protein was nonspecific.[Citation17] Hines and Foegeding[Citation15] reported a synergistic effect from the interaction and co-aggregation involving the three proteins: α-lactalbumin, β-lactoglobulin, and bovine serum albumin. Ngarize et al.[Citation8] demonstrated that large deformation testing can detect synergistic interactions in EA/WPI mixtures, and Raman spectroscopy showed that the protein network was strengthened by hydrogen bonding, hydrophobic effect, and changes in disulfide bonds.
Light Transmission
The light transmission spectral profiles of the individual proteins at 25°C and 60°C, from 400 to 700 nm wavelength revealed unique patterns for each protein EA, WPI, SPI, and CC; by temperature () and pH (). These unique protein transmission patterns did not change due to temperature or pH, but the bands shifted upward by an increase in concentration of protein and by solubility (). The transmission profile estimates were protein-specific between 400 and 700 nm. For each particular protein and concentration combination, the amount of light absorbed increased as the amount of soluble proteins in the supernatant increased (). Percent light transmittance was higher at 60°C than at 25°C.
Table 8 Spectral measurements concentrations of calcium caseinate (CC), whey protein isolate (WPI), egg albumins (EA), fish protein isolate (FPI), and soy proteins isolate (SPI) at pH 6.8 and 25°C temperature
Figure 2 (A) Spectral curves at 25 and 60°C and pH 6.8. Calcium caseinate (CC) or whey protein isolate (WPI), egg albumin (EA), fish protein isolate (FPI), soy protein isolate (SPI). (B) Spectral curves at 60°C of CC, EA, FPI, SPI, and WPI at pH 2.5, 6.8, and 9.0.
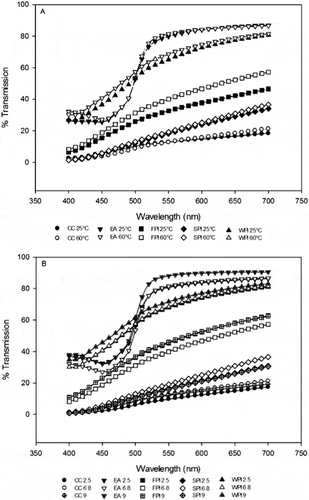
Figure 3 Transmission spectroscopy spectral profiles of proteins at 25 or 60°C and pH 2.5, 6.8, and 9.0. (A) Calcium caseinate, (B) whey protein isolate, (C) egg albumin, (D) fish protein isolate, and (E) soy protein isolate.
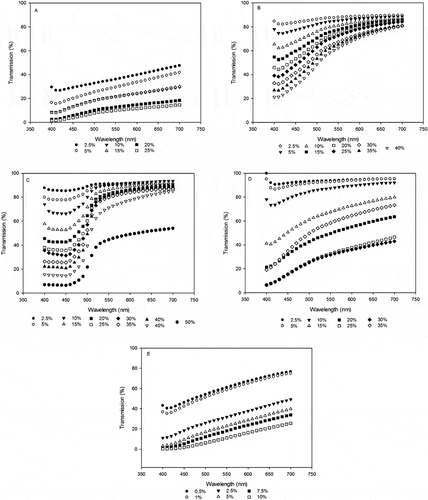
Below 500 nm, EA at 60°C had the highest transmission, and above 500 nm, EA had the highest transmission at both temperature conditions of 25 and 60°C. CC had the lowest transmission values at both temperatures (). The order of light transmission for the proteins was EA > WPI > FPI > SPI > CC. When compared to the solubility order for the proteins WPI > EA > FPI> SPI > CC, a definite correlation between solubility and light transmission was established. In a previous study, Ikeda[Citation13] confirmed the aggregation of whey protein gels using Raman scattering spectroscopy. Fitzsimons et al.[Citation7] characterized increased viscosity and turbidity by absorbance at 600 nm, which correlated with increasing gel strength with temperatures from 45 to 85°C. Fitzsimons et al.[Citation7] demonstrated both unique protein patterns, and shifts in spectral patterns with respect to concentration, temperature, and pH.
The unique patterns and orderly shifts of protein band patterns depending on concentration remained, with respect to temperature and pH (). The transmission patterns above 500 nm followed the same order as temperature EA > WPI > FPI > SPI > CC. Except for SPI at pH 6.8, increasing pH did not shift transmission spectra as much as temperature (). This shows that it is possible to identify these food protein types based on transmission patterns (); though the concentration of each protein may shift the profile with increasing solubility, the patterns remain the same. The spectral values for the proteins are presented in . For the five proteins, spectral profiles at 480 nm and solubility trends were consistent except for a few anomalies with FPI, SPI, and WPI, which affected correlation of 480 nm transmission and solubility.
The five proteins were separated into two groups by solubility profiles (). The R2 values for the top high solubility protein group EA, WPI, SPI, and CC was (R2 = 0.549). The low solubility protein FPI was (R2 = 0.029). Using the spectral profile for solubility, the proteins were characterized by spectrometric transmission values in the order: WPI > EA > SPI > CC > FPI. The spectral linear regression model R2 value for each protein was CC (R2 = 0.823); EA (R2 = 0.012); FPI (R2 = 0.602); SPI (R2 = 0.772), and WPI (R2 = 0.745). The linear correlation values were significant (P < 0.05) except for EA. Peng et al.[40] demonstrated that protein-protein interactions were affected by solubility through turbidity measurements. The turbidity of the mixture of soy proteins and skeletal muscle myosin was a consistent characteristic pattern for solubility and turbidity.[Citation40] Though the patterns of mixed protein blends were not measured, it is expected that similar patterns will hold true as observed by Peng et al.[Citation40] Schorsch et al.[Citation25] showed that the turbidity of a mixed system of casein micelle and whey dispersions measured by light scattering at 800 nm decreased due to particulate aggregation visible through transmission electron micrographs. Because of the low R2 value for EA, it is surmised that highly structured gels may be difficult to measure by spectrophotometer.
Scanning Electron Microscopy (SEM)
The SEM images for the five proteins were structurally different from each other at both temperatures 25 and 60°C, pH at 6.8 (). CC () and WPI () both appear granular, with CC displaying a more open surface. CC at 25°C () displays granular aggregates ranging from 20 to 200 nm with small interspatial gels. CC at 60°C () shows that the size of the aggregates increase to a range of 50 to 500 nm; the length and size of the aggregates increased with larger interspatial voids. The WPI slurry at 25°C () was larger than the CC with more ovoid shapes ranging from 50 to 400 nm; at 60°C (), WPI showed that some of the aggregate increased up to 800 nm. The mixed slurry of CC (10 wt%)/WPI (5%) shows a dried, fused mass, with tissues and very small undistinguished aggregates <50 nm (). The mixed incipient gel of CC (10 wt%)/WPI (5%) at 60°C showed large aggregate protein bodies >1000 nm in diameter ().
Figure 5 Scanning electron microscopy of calcium caseinate (CC) or whey protein isolate (WPI), and CC/WPI slurries (25°C) and gels (60°C) at pH 6.8. (A) CC (25°C), (B) CC (60°C), (C) WPI (25°C), (D) WPI (60°C), (E) CC/WPI (10:5 wt%) 25°C, and (F) CC/WPI (10:5 wt%) 60°C.
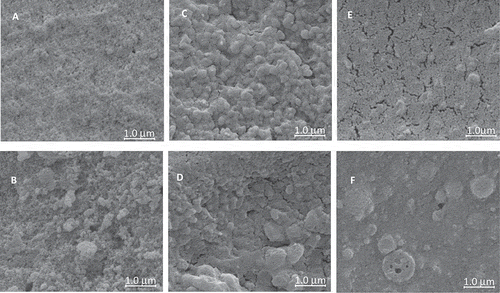
Proteins with smaller aggregates did not have interspatial voids. Maltais et al.[Citation41] showed through transmission electron microscopy that filamentous and particulate networks were related to gel strength, and that cold-set soy protein hydrogels, and particulate aggregate gels were more elastic while filamentous gels were more viscous. CC, WPI, and CC/WPI incipient gels at 60°C, pH 2.5, and pH 9.0 were significantly different in structures than at pH 6.8 (). CC at pH 2.5 () had large globular aggregates 300 to 800 nm in length with defined network structures; the globular structures disappeared with a more pronounced network remaining at pH 9.0 (). WPI incipient gels at 60°C, pH 2.5 were loose networks of stretched proteins (); the networks were more organized and stronger at pH 9.0 (). The mixed CC/WPI gel (10/5 w%) at pH 2.5 was a globular gel system with large globules >1000 nm and multiple smaller globules <500 nm (); the structure became networked at pH 9.0 (). CC gels were globular at pH 2.5; WPI networks were formed at both pH 2.5 and 9.0.
Figure 6 Scanning electron microscopy of calcium caseinate (CC) or whey protein isolate (WPI), and CC/WPI gels at 60°C. (A) CC (pH 2.5), (B) CC (pH 9.0), (C) WPI (pH 2.5), (D) WPI (pH 9.0), (E) CC/WPI (10:5 wt%) pH 2.5, and (F) CC/WPI (10:5 wt%) pH 9.0.
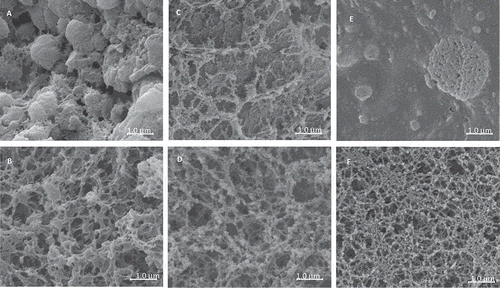
Overall, the mixed protein viscosities increased over individual protein viscosity values, and mixed protein flow indices were not affected except for a few cases where they increased over both individual values and a few that decreased below both individual values. Relative viscosity was in decreasing order: SPI, EA, CC, WPI, and FPI. Further evidence is needed to determine what protein properties produced these effects. Because multiple protein gels are complex systems, many aspects of their flow behavior must be studied individually to fully understand what effects shear, time, and temperature have on the gels. In classical models for multiple emulsions, the relative viscosity depends on the volume fraction of the total dispersed phase, and the ratios of the primary constituents.[Citation42] Knowledge of characteristic protein dispersion and the relationship between solubility, viscoelastic properties, and microstructure will allow protein-enriched foods to be tailored toward specific functional properties.[Citation43]
CONCLUSION
The optimal solubility concentration for the different proteins varied significantly with whey protein isolate and egg albumin being more soluble at higher concentrations than the rest. The effect of mixing different food proteins on the physical properties of slurries and gels was protein source specific, responding differently to the external conditions of pH, temperature, and concentration. In particular, pH affected solubility of calcium caseinate; temperature had the most effect on egg albumin performance, but protein concentration had the most effect on solubility of calcium caseinate and soy protein isolate. There was evidence of protein-protein interaction, which enhanced the viscosity of the calcium caseinate, whey protein isolates, or egg albumin. The viscosity of the mixed proteins decreased compared to the individual proteins except for certain egg albumin blends. Adding egg albumin to dairy proteins increased the viscosity of calcium caseinate and whey protein isolate. Mixed food protein systems are complex and can change functional behaviors, such as viscosity, depending on the miscibility of the proteins. Differences in co-solubility of mixed proteins may create unique networks and gel structures. It will useful to develop a prori estimation of the effects of combining food proteins from different sources on texture and gel structure in foods.
ACKNOWLEDGMENTS
The authors wish to thank Erica Torres and Eric Tilman for the help with solubility and viscosity assays and Dr. Peter Cooke and Guoping Bao for help with the microscopy.
REFERENCES
- Damodaran, S. Amino acids, peptides, and proteins. In: Fennema’s Food Chemistry, 4th Edition; Damodaran S.; Parkin, K.L.; Fenenema, O.R., Eds.; CRC Press, Taylor & Francis Group: Boca Raton, FL, 2008; 271–329.
- Pelegrine, D.H.G.; Gasparetto, C.A. Whey proteins solubility as function of temperature and pH. LWT–Food Science and Technology 2005, 38, 77–88.
- Roth, C.M.; Neal, B.L.; Lenhoff, A.M. Van der Waals interactions involving proteins. Biophysical Journal 1996, 70, 977–987.
- Beliciu, C.M.; Moraru, C.I. The effect of protein concentration and heat treatment temperature on micellar casein–soy protein mixtures. Food Hydrocolloids 2011, 25, 1448–1460.
- Polyakov, V.I.; Grinberg, V.Ya.; Tolstoguzov, V.B. Thermodynamic incompatibility of proteins. Food Hydrocolloids 1997, 11, 171–180.
- Dumetz, A.C.; Chockla, A.M.; Kaler, E.W.; Lenhoff, A.M. Protein phase behavior in aqueous solutions: Crystallization, liquid-liquid phase separation, gels, and aggregates. Biophysical Journal 2008, 94, 570–583.
- Fitzsimons, S.M.; Mulvihill, D.M.; Morris, E.R. Segregative interactions between gelatin and polymerized whey protein. Food Hydrocolloids 2008, 22, 485–491.
- Ngarize, S.; Adams, A.; Howekk, N.K. Studies on egg albumin and whey protein interactions by FT-Raman spectroscopy and rheology. Food Hydrocolloids 2004, 18, 49–59.
- Pogaku, R.; Seng, C.E.; Boonbeng, L.; Kallu, U.R. Whey protein isolate-starch system—A critical review. International Journal Food Engineering 2007, 3, 1–28.
- Patocka, G.; Cervenkova, R.; Narine, S.; Jelen, P. Rheological behavior of dairy products as affected by soluble whey protein isolate. International Dairy Journal 2006, 16, 399–405.
- Nunes, M.C.; Raymundo, A.; Sousa, I. Rheological behaviour and microstructure of pea protein/k-carrageenan/starch gels with different setting conditions. Food Hydrocolloids 2006, 20, 106–113.
- Glibowski, P. Rheological properties and structures of inulin-whey protein gels. International Dairy Journal 2009, 19, 443–449.
- Ikeda, S. Heat-induced gelation of whey proteins observed by rheology, atomic force microscopy, and Raman scattering spectroscopy. Food Hydrocolloids 2003, 17, 339–406.
- van Vliet, T.; Lakemond, C.M.M.; Visschers, R.W. Rheology and structure of milk protein gels. Current Opinion in Colloid & Interface Science 2004, 9, 298–304.
- Hines, M.E.; Foegeding, E.A. Interactions of α-lactalbumin and bovine serum albumin with β-lactoglobulin in thermally induced gelation. Journal of Agricultural and Food Chemistry 1993, 41, 341–346.
- van der Linden, E.; Venema, P. Self-assembly and aggregation of proteins. Current Opinion in Colloid & Interface Science 2007, 12, 158–165.
- Kavanagh, G.M.; Clark, A.H.; Gosal, W.S.; Ross-Murphy, S.B. Heat-induced gelation of β-lactoglobulin/α-lactalbumin blends at pH 3 and pH 7. Macromolecules 2000, 33, 7029–7037.
- Mohammed, Z.H.; Hill, S.E.; Mitchell, J.R. Covalent crosslinking in heated protein systems. Journal of Food Science 2000, 65, 221–226.
- Matia-Merino, L.; Singh, H. Acid-induced gelation of milk protein concentrates with added pectin: Effect of casein micelle dissociation. Food Hydrocolloids 2007, 21, 765–775.
- Pace, C.N.; Trevino, S.; Prabhakaran, E.; Scholtz, M. Protein structure, stability and solubility in water and other solvents. Philosophical Transactions of the Royal Society B: Biological 2004, 359, 1225–1235.
- Anandharamakrishna, C.; Rielly, C.D.; Stapley, A.G.F. Loss of solubility of ∞-lactalbumin and β-lactoglobulin during the spray drying of whey proteins. LWT–Food Science and Technology 2008, 41, 270–277.
- Pelegrine, D.H.G.; Moraes Santos Gomes, M.T. Whey proteins solubility curves at several temperature values. Ciencia e Natura, Universidade Federal de Santa Maria 2008, 30, 17–25.
- Kilara, A.; Mangino, M.E. Relationship of solubility of whey protein concentrates to thermal properties determined by differential scanning calorimetry. Journal of Dairy Science 1991, 56, 1448–1449.
- Kilara, A. Standardization of methodology for evaluating whey proteins. Journal of Dairy Science 1984, 67, 2734–2744.
- Schorsch, C.; Wilkins, D.K.; Jones, M.G.; Norton, I.T. Gelation of casein-whey mixtures: Effects of heating whey proteins alone or in the presence of casein micelles. Journal of Dairy Research 2001, 68, 471–481.
- Davies, A.R.; Fish, W.W.; Perkins-Veazie, P. A rapid hexane-free method for analyzing lycopene content in watermelon. Journal of Food Science 2003, 68, 328–332.
- Jenkins, W.T. Three solutions of the protein solubility problem. Protein Science 1998, 7, 376–382.
- de Wit, J.N.; Klarenbeek, G. Effects of various heat treatments on structure and solubility of whey proteins. Journal of Dairy Science 1984, 67, 2701–2710.
- Tang, Q.; McCarthy, O.J.; Munro, P.A. Oscillatory rheological comparison of the gelling characteristics of egg white, whey protein concentrates, whey protein isolate, and β-lactoglobulin. Journal of Agricultural and Food Chemistry 1994, 42, 2126–2130.
- Ren, C.; Tang, L.; Zhang, M.; Guo, S. Interactions between whey soybean protein (WSP) and β-conglycinin (7S) during the formation of protein particles at elevated temperatures. Food Hydrocolloids 2009, 23, 936–941.
- Tabilo-Munizaga, G.; Barbosa-Canovas, G.V. Rheology for the food industry. Journal of Food Engineering 2005, 67, 147–156.
- O’Kennedy, B.T.; Kelly, P.M. Evaluation of milk protein interactions during acid gelation using a simulated yoghurt model. Milchwissenschaft 2000, 55, 187–190.
- Moraru, C.I.; Lee, T-C.; Karwe, M.V.; Kokini, J.L. Phase behavior of a meat—Starch extrudates illustrated on a state diagram. Journal Food Science 2002, 67, 3026–3032.
- Tolstoguzov, V.B. Functional properties of food proteins and role of protein–polysaccharide interaction. Food Hydrocolloids 1991, 4, 429–468.
- Famelart, M-H.; Tomazewski, J.; Piot, M.; Pezennec, S. Comparison of rheological properties of acid gels made from heated casein combined with beta-lactoglobulin or egg ovalbumin. International Dairy Journal 2003, 13, 123–134.
- Campo-Deano, L.; Tovar, C. The effect of egg albumen on the viscosity of crab sticks made from Alaska Pollock and Pacific Surimi. Food Hydrocolloids 2009, 23, 1641–1646.
- Brenner, T.; Nicolai, T.; Johannsson, R. Rheology of thermo-reversible fish protein isolate gels. Food Research International 2009, 42, 915–924.
- Badii, F.; Howell, N. Fish gelatin: Gelling properties and interaction with egg albumin proteins. Food Hydrocolloids 2006, 20, 630–640.
- Matringe, E.; Phan Tan Luu, R.; Lorient, D. Functional properties of milk-egg mixtures. Journal of Food Science 1999, 64, 787–791.
- Peng, I.C.; Dayton, W.R.; Quass, D.W.; Allen, C.E. Investigations of soybean 11S protein and myosin interaction by solubility, turbidity and titration studies. Journal of Food Science 1982, 47, 1976–1983.
- Maltais, A.; Romondetto, G.E.; Subirade, M. Soy protein cold-set hydrogels as controlled delivery devices for nutraceutical compounds. Food Hydrocolloids 2009, 23, 1647–1653.
- Pal, R. Viscosity models for multiple emulsions. Food Hydrocolloids 2008, 22, 428–438.
- Tung, M. Rheology of protein dispersions. Journal of Texture Studies 1978, 9, 3–31.