Abstract
Gelation property, thermal property, protein subunits distribution, and molecular forces involved in gelation of alcohol-extracted soy protein isolate were investigated using texture analyzer, differential scanning calorimeter, sodium dodecyl sulfate polyacrylamide gel electrophoresis, and various reagents. Results showed that salts and pH played important roles in gel firmness, and a power law relationship between gel firmness and protein concentration was observed. The effects of various reagents revealed that disulfide bonds play a major role in soy protein gel formation, while the involvement of electrostatic interactions, hydrogen bonds, and/or hydrophobic interactions also occurred in gel networks. Thermal analysis indicated that both alcohol-extracted soy protein isolate and commercial soy protein isolate (isoelectric precipitation) have undergone serious denaturation, while the gel firmness of alcohol-extracted soy protein isolate was significantly greater than that of commercial soy protein isolate. Sodium dodecyl sulfate polyacrylamide gel electrophoresis image showed that there was almost no difference for protein subunits among alcohol-extracted soy protein isolate, commercial soy protein isolate, and soy powder. Hence, as an alternative environmental friendly extraction method, alcohol-extraction of soy protein isolate has a great prospect to replace presently applied isoelectric precipitation method.
INTRODUCTION
Soybeans are an abundant and relatively cheap source of proteins that are recognized for their high nutritional value and superior functional properties. Among these functional properties, gel forming ability upon heating is especially important for the application in emulsified meat products. Although a number of studies have focused on gelation properties of plant proteins, especially soy proteins, commercial soy protein isolate (SPIc) is usually extracted by acid precipitation method, and this approach results in serious environmental contamination. Hence, to induce an environmental friendly extraction procedure, alcohol extraction of soy protein has been developed and applied to produce alcohol-extracted soy protein isolate (SPIae).
Protein gelation is the formation of a three-dimensional network through the cross-linking of its polypeptide chains. Different molecular forces result in cross-linking of proteins and may involve hydrogen bonds, ionic attractions, disulphide bonds, hydrophobic associations, or a combination of the above.[Citation1] Some works have been done to investigate the effect of salt (ionic strength) and pH on gelation properties of plant proteins,[Citation2,Citation3] and it was shown that gelation properties of plant proteins were affected by salt and pH and stronger gels formed at adequate salt concentration (0.3–0.6 M NaCl) and in the acidic pH range (3–6).
The molecular forces involved in the gel network formation are dependent on the protein. It can be deduced from effects of pH, salts, reducing agents, and dissociating agents that there is an involvement of different molecular forces in the formation of protein gels.[Citation4−Citation6] Gel strength is affected by pH and salts when electrostatic forces are involved in gel formation of whole plasma protein.[Citation7−Citation11] The effects of salts on protein structure involve three mechanisms: electrostatic shielding effects, non-specific charge neutralization, and direct ion-macromolecule interactions.[Citation12] It is believed that the ion specific effects arise from changes in the hydrophobic core of the protein.[Citation12] At low ionic strengths, salts are believed to mainly influence electrostatic interactions by interacting with charged groups on the proteins, while at higher concentrations the ion specific effects, or lyotropic effects, become prominent.[Citation13] At higher concentrations, NaSCN has been shown to be a destabilizing salt while NaCl stabilizes protein structure.[Citation13,Citation14] Therefore, involvement of electrostatic interactions can be determined by the effect of salts and pH. Sulfhydryl/disulfide interchange has been proposed to be involved in soy protein gelation based on the gelling property when β-mercaptoethanol (β-ME or 2-ME) was involved.[Citation6,Citation15−Citation18] Hydrogen bonds and hydrophobic interactions can be destabilized by urea and guanidine hydrochloride (GuHCl). Urea denatures a protein molecule, dehydrates the molecules and causes repulsion between proteins, and stabilizes the unfolded form through preferential adsorption with charged protein solutes.[Citation19] Therefore, urea most likely interferes with both hydrophobic interactions and hydrogen bonding by dehydrating protein molecules and interacting through hydrogen bonds.
The objectives of this research were to determine gelation properties of SPIae and evaluate the effects of salts (NaSCN and NaCl), chemicals that target noncovalent interactions (PG, urea, and GuHCl), and covalent interactions (2-ME) on its gelling property. This allows us to compare the difference of gel firmness between SPIae and SPIc, elucidating the molecular forces involved in gel network formation and maintenance.
MATERIALS AND METHODS
Materials
Commercial soy protein isolate (SPIc, HGK-A807, high gelling capacity) was extracted by isoelectric precipitation and kindly provided by Harbin Hi-tech Soybean Food Co., Ltd. SPIae was kindly provided by Faculty of Food Science, Northeast Agricultural University, China. The two proteins contained 91.42 and 91.97% of protein as determined by Kjeldahl method using an N to protein conversion factor of 6.25,[Citation20] respectively. Commercial soybean was ground using a Falling Number Laboratory Mill 3100, with a 0.8-mm screen to make soy powder. Sodium dodecyl sulfate–polyacrylamide gel electrophoresis (SDS–PAGE) molecular weight standard (10–170 kDa) was purchased from SunShine Biotechnology Co. Ltd., China. All other chemicals were of analytical grade.
Gel Preparation
The method of Sun and Arntfield[Citation21] was followed with minor modifications to prepare gels. Initially, 50 mL of SPIae (7, 9, 11, 13, 15, 17, and 19%, w/v) were prepared using designed NaCl (0, 0.1, 0.2, 0.3, 0.4, 0.5, and 0.6 M) or other chemical solutions including NaSCN (0, 0.3, 1, and 3 M), urea (0, 1, 2, 3, 5, and 8 M), PG (0, 5, 10, 15, and 20%), 2-ME (0, 0.1, 0.2, and 0.3 M), and GuHCl (0, 0.3, 1.0, and 3.0 M) to achieve desired concentrations, pH was adjusted using 1 M HCl or 1 M NaOH to 5.5, 6.0, 6.5, 7.0, 7.5, 8.0, and 8.5 if necessary. SPIc (15%, w/v) was dispersed in 0.2 M NaCl. All samples were prepared in 200-mL aluminum cans according to the experimental design, covered with lids, and then heated in an oven at 95°C for 30 min. SPIae gel samples were cooled down overnight at room temperature and then texture analysis was performed.
Texture Analysis of Protein Gels
To evaluate the texture of protein gels, the method of Sun and Arntfield[Citation21] was followed with minor modifications. A TA.XTPlus texture analyzer (Stable Micro Systems, Godalming, UK) equipped with a 1-cm diameter metal sphere probe was used to test gel firmness of different samples in the cans. The equipment was set as follows: pre-test speed: 5.0 mm/s; test speed: 0.1 mm/s; post-test speed: 10.0 mm/s; rupture test distance: 5.0 mm; trigger force: 1 g. All samples were prepared in duplicate and tested six times.
Differential Scanning Calorimeter (DSC)
The thermal properties of the SPIc and SPIae suspensions were examined using a DSC Q2000 (TA Instruments, New Castle, DE, USA). Instrumental conditions were as described previously.[Citation22] In a typical experiment, 20–30 μL of a 10% (w/v) protein sample was sealed in a pre-weighed aluminum pan and the pan was weighed again. An empty pan was used as reference. The sample was heated over a temperature range of 30–150°C in a standard DSC cell. Thermal curves were obtained at a heating rate of 10°C/min. Each sample was analyzed in duplicate.
SDS–PAGE
To evaluate subunits in the protein gels, the method of Poysa et al.[Citation23] was followed with minor modifications. To start, 5 mg of SPI samples and 12 mg of soybean powder were weighed into a microcentrifuge tube and 1 mL sample buffer (4% SDS, 20% glycerol, 10% 2-mercaptoethanol, 0.125 M Tris–HCl, and 0.01% Pyronin Y, pH 6.8) was added. Samples were vortexed for 1 min, boiled for 10 min in a water bath, and vortexed for an additional 1 min to promote dissolution. The samples were then centrifuged at 2200× g in a minicentrifuge to settle out any remaining particulate matter and 5 μL of the supernatant were applied to each well of a gel containing a 4% acrylamide stacking gel and 12% acrylamide separating gel. Next, 5 μL SDS–PAGE molecular weight standards were loaded into one well. Gels were run at 10 amp per gel (mini-PROTEAN Tetra Cell, Bio-Rad, China) for about 2 h until the dye reached the bottom of the gel. The gel was exposed to a staining solution consisting of 0.1% (w/v) Coomassie Brilliant Blue G-250, 10% (w/v) acetic acid, and 40% (w/v) methanol overnight to visualize the protein bands. A destaining solution containing 20% methanol and 10% acetic acid was used to destain the gels. The gels were photographed using a Canon EOS 450D digital camera to analyze band intensity and molecular weights.
Statistical Analysis
All data were analyzed for significant differences by one-way analysis of variance (ANOVA) between means, with the minimum significant level set at 5% (P < 0.05), using Tukey’s Multiple Comparison Test by GraphPad InStat software version 3.06 (GraphPad Software Inc., La Jolla, CA, USA).
RESULTS AND DISCUSSION
Effect of NaCl and NaSCN on Gel Firmness of SPIae
As seen in , the firmness value of formed gel without adding NaCl (0 M) was very small. This low gel firmness may be due to aggregation of protein molecules since without salt soy protein solubility is low and the suspension is unstable, easily denatured by heating and the denatured proteins would aggregate to form aggregates. Due to severe aggregation, soy protein isolate was more likely to form macroaggregates and was unfavorable for gel formation. Another explanation is that electrostatic repulsion between proteins prevented gel formation. Studies on the various forces involved in network formation and their influence on rheological properties of milk β-Lactoglobulin (β-Lg) protein gels suggested that excessive repulsive forces created a high enough energy barrier to prevent denatured protein molecules from associating to form strong self-supporting gels when NaCl was not added.[Citation5,Citation24]
FIGURE 1 (a) Effect of NaCl on gel firmness of SPIae, 15% (w/v) soy proteins isolate, natural pH 6.93. (b) Effect of NaSCN on gel firmness of SPIae, 15% SPI (w/v), 0.2 M NaCl. Error bars represent standard deviation. a–dDifferent letters indicate statistically significant differences among samples.
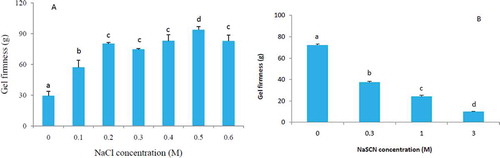
The higher gel firmness values were seen between 0.2 and 0.6 M NaCl and gel firmness value was the highest at 0.5 M NaCl, which was significantly higher than other salt concentrations (p < 0.05, ). The firmest gels were found between 0.2 and 0.6 M NaCl as the balance between attractive and repulsive forces was suitable for gel formation. At higher salt concentrations, gel firmness gradually decreased. Hermansson and Akesson[Citation25] have suggested that an increase in protein-solvent interaction could weaken protein gels at higher salt concentrations. Our results were in agreement with their findings.
As NaSCN is a chaotropic salt that destabilizes proteins in solution and promotes protein solubility, its effect on protein gel network formation was interesting. In a study of chaotropic anions on the release of insoluble membrane proteins, Hincha[Citation26] observed that the presence of chaotropic salts reduced the energy barrier for the dissociation of proteins from their binding sites on the membrane. In the present study, this reduction in the energy at the relatively low NaSCN concentration led to a dramatic reduction in gel firmess (). Higher NaSCN concentrations were examined to further investigate the impact of this chaotropic salt. With 1 M NaSCN, the gel firmness was continually reduced, and with 3 M NaSCN, gel firmness was lower than 20 g. Some researchers proposed that the binding of SCN− at the higher concentrations resulted in a change in overall protein charges such that the structure was destabilized, thus gel forming ability was greatly inhibited.[Citation13,Citation14] Our result was in agreement with their conclusion.
Effect of pH on Gel Firmness of SPIae
Through pre-experiment we found that a SPIae concentration of 15%, 0.2 M NaCl at natural pH (6.93) was necessary to form a good gel. Therefore, we have used 15% protein to study the effect of pH on gelation properties. A salt concentration of 0.2 M was chosen to evaluate a wide pH range because this concentration is normal in emulsified meat products. The gel firmness was dependent on pH () and had increased values at both acidic and alkaline values, respectively. This is consistent with the observation of Egelandsdal,[Citation27] who also found stronger ovalbumin gels formed at acidic and alkaline pH values, respectively. From the above results it would appear that at acidic and alkaline pH values, heating conditions (95°C) were sufficient to achieve denaturation, resulting in increased protein-protein interaction and the higher gel firmness values. Renkema et al.[Citation3] indicated that the mechanism of gel formation seemed to be affected by pH and the disulphide bridge between the acidic and the basic polypeptide of glycinin was broken at pH 7.6 in contrast to pH 3.8 upon heating. Our result showed that the gel firmness was low at pH 8, which probably would attribute to the broken glycinin polypeptide between the acidic and basic subunits.
Effect of Soy Protein Concentrations on Gel Firmness of SPIae
The effect of protein concentration on gel firmness is shown in . It can be seen that the gel firmness rose with increased SPIae concentration (in the range of 7–19%), due to the increase in opportunities for cross-linking of protein molecules. It was concluded that higher SPIae concentrations induced the formation of stronger gels. Our result is in accordance with previous reports by Renkema and van Vliet,[Citation28] who also observed increased gel stiffness for soy protein isolate, purified glycinin, and a β-conglycinin-rich fraction with increasing protein concentration. Power law relationship between gel firmness and protein concentration was obtained for SPIae:
FIGURE 3 Relationship between soy protein concentration and gel firmness for heat induced networks, 0.2 M NaCl, natural pH 6.93. Error bars represent standard deviation. a–fDifferent letters indicate statistically significant differences among samples.
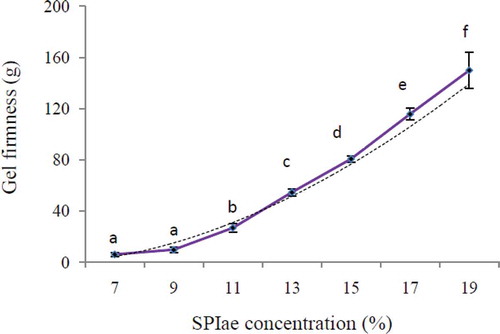
Effects of Different Reagents on Non-Covalent Bonds
Different molecular forces, including hydrogen bond, hydrophobic and electrostatic interactions, tend to be disrupted by most chaotropic agents, such as detergents, urea, or GuHCl.[Citation29] To investigate these non-covalent bonds that contribute to the gel formation, different reagents (GuHCl, PG, and urea) were added to SPIae dispersion containing 0.2 M NaCl prior to heat treatment.
Effect of urea
Zou et al.[Citation30] indicated that hydrophobic groups and hydrophilic groups are involved in the denaturation caused by urea and suggested that urea binds to amide groups through hydrogen bonds, reducing the hydrophobic effect through dehydration of the protein molecule. Walstra[Citation31] also indicated that by a dehydration of peptide bonds, which were bound by urea, also weakens hydrophobic interactions caused by the denaturing effect of urea. In , gel firmness decreased dramatically when urea was added and gel firmness was dropped to a very small value when urea concentration was greater than 5 M. It can be concluded that urea denatured soy protein by breaking down hydrogen bonds and hydrophobic interactions, preventing network formation, and this reduction in soy protein gel firmness confirms the involvement of hydrogen bonds and/or hydrophobic interactions in gel networks.
FIGURE 4 (a) Effect of urea on gel firmness of SPIae, 15% SPI (w/v), 0.2 M NaCl. (b) Effect of PG on gel firmness of SPIae, 15% SPI (w/v), 0.2 M NaCl. (c) Effect of 2-ME on gel firmness of SPIae, 15% SPI (w/v), 0.2 M NaCl. Error bars represent standard deviation. a–eDifferent letters indicate statistically significant differences among samples.
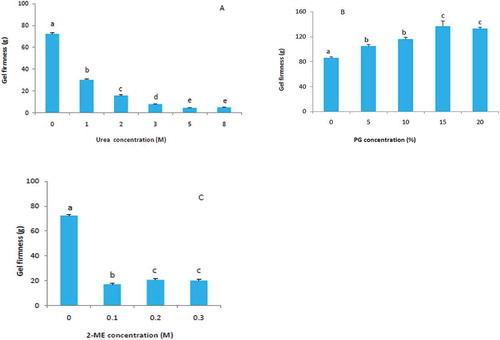
Tanford[Citation32] pointed out that urea is a strong denaturing agent, which can result in an extensively unfolded state, in which the protein molecule behaves like a random coil. The denatured states induced by other denaturants, for example, 2-ME or DTT, are “intermediate” states between native and urea denatured states.[Citation32] In the overall balance of attractive and repulsive forces contributing to soy protein gel network formation, hydrogen bonds, and hydrophobic and electrostatic interactions are all believed to be important. In a most recent study, Liu et al.[Citation33] found that weak gel network of soy β-conglycinin was susceptible to ionic strength, suggesting that electrostatic forces play an important role in the formation of weak gel network.
Effect of PG
PG may disrupt hydrophobic forces while enhancing hydrogen bonds and electrostatic interactions by lowering the dielectric constant of the solvent, and reducing the energy barrier to protein-protein interaction enough to enable structure formation.[Citation6] Our results showed that the gel firmness of SPIae increased dramatically when 5 and 10% PG was added, then significantly increased with the increasing amount of added PG to 15 and 20% (), which might reflect the effect of PG on disruption of the hydrophobic interaction, and increasing gel firmness by promoting protein-protein interactions through electrostatic interaction and hydrogen bonds. This would suggest that hydrophobic interaction, hydrogen bonds, and electrostatic interaction all participated in formation of SPIae gel. These results are consistent with the suggestion that electrostatic interactions are important in the formation of elastic gels, and suggest that hydrogen bonding complements electrostatic interactions in soy protein gels. Utsumi and Kinsella[Citation6] proposed that for molecular forces that were involved in soy protein gel formation and maintenance, mostly hydrogen bonds in 7S globulin gels and hydrogen bonds and hydrophobic interactions in soy protein isolate gels. Our results are in agreement with their findings.
Effect of 2-ME on covalent bonds
By competing for sulfhydryl group, mercaptoethanol can reduce disulfide bonds of protein.[Citation34] As a result, the effect of adding 2-ME reflects the contribution of disulfide bonds to soy protein gel network formation and maintenance. As seen in , gel firmness significantly decreased with addition of 2-ME. This phenomenon is consistent with the result of Utsumi and Kinsella,[Citation6] who suggested that disulfide bonds were involved in the formation of soybean 11S globulin gels.
TABLE 1 Effect of GuHCl on gel firmness of SPIae
TABLE 2 Comparison of gel firmness and thermal properties of SPIae and SPIc.*
Effect of GuHCl
Tanford[Citation31] indicated that GuHCl is a strong ionic denaturing agent, which weakens hydrophobic interactions and inhibits hydrogen and ionic bonds. He proposed that GuHCl gave the most extensively unfolded state, in which the protein molecules lose their native conformation and perform as random coils. GuHCl is a more effective denaturant than urea, unfolding proteins at two to three times lower concentrations than urea.[Citation35] There was a gradual decrease in the gel firmness values with increasing levels of GuHCl. At a concentration of 3.0 M GuHCl, gel formation was completely inhibited (). This evidence supports the need for hydrogen and ionic bonds in gel formation.
Comparison of SPIae and SPIc
In , results showed that there was a significant difference for gel firmness between SPIae and SPIc, where the gel firmness of SPIae was greater than that of SPIc. This indicated that alcohol extracted soy protein isolate could form stronger gel than the commercial soy protein isolate used here. A similar result was obtained by Ishino and Kudo[Citation36] and Hua et al.,[Citation37] who also observed improved gel firmness for alcohol extracted SPI than conventional SPI (isoelectric precipitation). The latter attributed this phenomenon to the effect of free radicals, which were generated from lipid oxidation on protein denaturation and aggregation. They indicated that this effect might have implications on the structure and hence, the aggregation behavior of SPIc, since at water extraction stage the reaction of lipid oxidation catalyzed by soybean lipoxygenase was much more significant for defatted soy flakes compared to alcohol washed soy flakes. For globular protein gelation, gel is formed through extensive cross-linking of aggregates. More interacting sites for cross-linking could be provided by protein aggregates with larger size and more extended structure, thus a stronger gel should form by SPIae. While for thermal properties of the two protein isolates, DSC curves showed a straight line for both of them, which indicated that these two proteins have undergone severe denaturation.
SDS–PAGE
The SPI prepared by alcohol extraction, isoelectric precipitation, and native soybean powder displayed similar electrophoretic patterns as shown in . Compared to soybean powder, the band densities of α-7S, α’-7S, β-amylase, β-7S, 11S, A1, A2, A3, and A4-11S subunits of SPIae and SPIc samples are almost the same. It seems like no significant differences exist for protein subunits between two extraction methods, and compared to soybean powder, some faint bands (for example, soybean agglutinin) indicate loss of certain components through protein extraction. This result is probably an indication of the fundamentally same gel firmness of the two extraction methods.
CONCLUSIONS
Our results indicate that salts and pH played important roles in gel formation of SPIae. The gel firmness of SPIae increased with increasing protein concentration, and a power law relationship between gel firmness and protein concentration was obtained. Disulfide bonds play a major role in soy protein gel firmness, while electrostatic interactions, hydrogen bonds, and/or hydrophobic interactions are also involved in gel formation. Thermal analysis indicated that both SPIae and SPIc have undergone severe denaturation, hence greatly reducing the gel firmness. However, the gel firmness of SPIae was significantly greater than that of SPIc. SDS-PAGE image showed that there was almost no difference for protein subunits among SPIae, SPIc, and soy powder. In general, as an environmental friendly extraction method, alcohol-extraction of soy protein isolate has great potential to replace the presently applied isoelectric precipitation method. Further study should focus on continuous improvement of gel firmness of SPIae.
REFERENCES
- Otte, J.; Schumacher, E.; Ipsen, R.; Ju, Z.; Qvist, K.B. Protease induced gelation of unheated and heated whey proteins: Effects of pH, temperature and concentration of proteins, enzyme and salts. International Dairy Journal 1999, 9, 801–812.
- Lakemond, C.M.M.; de Jongh, H.H.J.; Paques, M.; van Vliet, T.; Gruppen, H.; Voragen, A.G.J. Gelation of soy glycinin: Influence of pH and ionic strength on network structure in relation to protein conformation. Food Hydrocolloids 2003, 17, 365–377.
- Renkema, J.M.S.; Lakemond, C.M.M.; de Jongh, H.H.J.; Gruppen, H.; van Vliet, T. The effect of pH on heat denaturation and gel forming properties of soy proteins. Journal of Biotechnology 2000, 79, 223–230.
- Clark, A.H.; Judge, F.J.; Richards, B.; Stubbs, J.; Suggett, A. Electron microscopy of network structure in thermally induced globular protein gels. International Journal of Peptide and Protein Research 1981, 17, 380–392.
- Mulvihill, D.M.; Rector, D.; Ensella, J.E. Effect of structuring and destructuring anionic ions on the rheological properties of thermally induced β-lactoglobulin gels. Food Hydrocolloids 1990, 4, 267–276.
- Utsumi, S.; Kinsella, J.E. Forces involved in soy protein gelation: Effects of various reagents on the formation, hardness and solubility of heat-induced gels made from 7S, 11S and soy isolate. Journal of Food Science 1985, 50, 1278–1282.
- Hickson, D.W.; Dill, C.W.; Morgan, R.G.; Suter, D.A.; Carpenter, Z.L. A comparison of heat-induced gel strength of bovine plasma and egg albumen proteins. Journal of Animal Science 1980, 51, 69–73.
- Hermansson, A.M. Gel characteristics—Compression and penetration of blood plasma gels. Journal of Food Science 1982, 47, 1960–1964.
- Hermansson, A.M. Gel characteristics—Structure as related to texture and water binding of blood plasma gels. Journal of Food Science 1982, 47, 1965–1972.
- O’Riordan, D.; Kinsella, J.E.; Mulvihill, D.M.; Morrissey, P.A. Gelation of plasma proteins. Food Chemistry 1988, 33, 203–214.
- O’Riordan, D.; Mulvihill, D.M.; Kinsella, J.E.; Morrissey, P.A. The effect of salts on the rheological properties of plasma protein gels. Food Chemistry 1988, 34, 1–11.
- Zhang, Y.; Cremer, P.S. Interactions between macromolecules and ions: The Hofmeister series. Current Opinion in Chemical Biology 2006, 10, 658–663.
- Damodaran, S.; Kinsella, J.E. The effect of neutral salts on the stability of macromolecules. Journal of Biological Chemistry 1981, 256, 3394–3398.
- von Hippel, P.H.; Schleich, T. Effects of neutral salts on the structure and conformational stability of macromolecules in solution. In: Structure and Stability of Biological Macromolecules; Timasheff, S.N.; Fasman, G.D.; Eds.; Marcel Dekker: New York, 1969; 417.
- Utsumi, S.; Damodaran, S.; Kinsella, J.E. Heat-induced interactions between soybean proteins: Preferential association of 11S basic subunits and β subunits of 7S. Journal of Agricultural and Food Chemistry 1984, 32, 1406–1412.
- Wolf, W.J.; Briggs, D.R. Studies on the cold-insoluble fraction of the water-extractable soybean proteins. II. Factors influencing conformation changes in the 11S component. Archives of Biochemistry and Biophysics 1958, 76, 377–393.
- Wolf, W.J. Sulfhydryl content of glycinin: Effect of reducing agents. Journal of Agricultural and Food Chemistry 1993, 41, 168–176.
- McKlem, L.K. Investigation of molecular forces involved in gelation of commercially prepared soy protein isolates. MS Thesis, North Carolina State University: Raleigh, North Carolina, 2002.
- Wallqvist, A.; Covell, D.G.; Thirumalai, D. Hydrophobic interactions in aqueous urea solutions with implications for the mechanism of protein denaturation. Journal of American Chemist Society 1998, 120, 427–428.
- AACC. Approved Methods of the American Association of Cereal Chemists, 8th Ed.; AACC: St. Paul, MN, 1982.
- Sun, X.D.; Arntfield, S.D. Gelation properties of chicken myofibrillar protein induced by transglutaminase crosslinking. Journal of Food Engineering 2011, 107, 226–233.
- Sun, X.D.; Arntfield, S.D. Gelation properties of salt extracted pea protein induced by heat treatment. Food Research International 2010, 43, 509–515.
- Poysa, V.; Woodrow, L.; Yu, K. Effect of soy protein subunit composition on tofu quality. Food Research International 2006, 39, 309–317.
- Mulvihill, D.M.; Rector, D.; Kinsella, J.E. Mercaptoethanol, N-ethylmaleimide, propylene glycol and urea effects on rheological properties of thermally induced β-lactoglobulin gels at alkaline pH. Journal of Food Science 1991, 56, 1338–1341.
- Hermansson, A.M.; Akesson, C. Functional properties of added proteins correlated with the properties of model meat systems. Effect of salt on water binding properties of model meat systems. Journal of Food Science 1975, 40, 603–610.
- Hincha, D.K. Release of two peripheral proteins from chloroplast thylakoid membranes in the presence of a Hofmeister series of chaotropic anions. Archives of Biochemistry and Biophysics 1998, 358, 385–390.
- Egelandsdal, B. Heat-induced gelling in solutions of ovalbumin. Journal of Food Science 1980, 45, 570–574.
- Renkema, J.M.S.; van Vliet, T. Concentration dependence of dynamic moduli of heat-induced soy protein gels. Food Hydrocolloids 2004, 18, 483–487.
- Sood, S.M.; Slattery, C.W. The use of lithium chloride to study human milk micelles. Journal of Dairy Science 2003, 86, 78–85.
- Zou, Q.; Habermann-Rottinghaus, S.M.; Murphy, K.P. Urea effects on protein stability: Hydrogen bonding and the hydrophobic effect. Proteins: Structure, Function, and Genetics 1998, 31, 107–115.
- Walstra, P. Physical Chemistry of Foods; Marcel Dekker, Inc.: New York, 2003.
- Tanford, C. Protein denaturation. Advances in Protein Chemistry 1968, 23, 121–282.
- Liu, C.; Yang, X.Q.; Ahmad, I.; Tang, C.H.; Li, L.; Zhu, J.H.; Qi, J.R. Rheological properties of soybean β-conglycinin in aqueous dispersions: Effects of concentration, ionic strength and thermal treatment. International Journal of Food Properties 2011, 14, 264–279.
- Wang, C.H.; Damodaran, S. Thermal gelation of globular proteins: Weight average molecular weight dependence of gel strength. Journal of Agricultural and Food Chemistry 1990, 38, 1157–1164.
- Greene, Jr., R.F.; Pace, C.N. Urea and guanidine hydrochloride denaturation of ribonuclease, lysozyme, α-chymotrypsin, and β-lactoglobulin. Journal of Biological Chemistry 1974, 249, 5388–5393.
- Ishino, K.; Kudo, S. Nutritional evaluation of soya beans (Glycine max): Nitrogen balance and fractionation studies. Agricultural and Biological Chemistry 1980, 44, 537–543.
- Hua, Y.; Huang, Y.; Qiu, A.; Liu, X. Properties of soy protein isolate prepared from aqueous alcohol washed soy flakes. Food Research International 2005, 38, 273–279.