Abstract
Curcumin is a natural polyphenolic compound with anticancer, anti-inflammatory, and antioxidation properties. Low water solubility and rapid hydrolytic degradation are two challenges limiting use of curcumin. In this study, the role of the native/modified forms of bovine serum albumin and casein, as food-grade biopolymers and safe drug delivery systems and also protein chemical modification in stabilizing of curcumin were surveyed. Investigation on curcumin stability indicated that curcumin binding to the native bovine serum albumin is stronger than the modified bovine serum albumin, and hence, native protein could suppress water-mediated curcumin degradation. Drug competitive binding, fluorescence resonance energy transfer, and molecular docking studies suggested that the binding site for curcumin has not been changed upon albumin modification. Moreover, different observations were made for both the native and modified caseins. It appears from thermodynamic analyses that in “protein-curcumin” systems, water molecules are excluded from the vicinity of curcumin, so that some of them may provide novel tools to increase both food quality and the bioavailability of curcumin as a health promoting agent.
INTRODUCTION
According to the International Food Information Council (IFIC) “functional food” is defined as “food that has health promotion properties beyond basic nutrition.” In this way, different phytochemicals, especially polyphenols, have attracted the attention and extensive research has been carried out to devise novel methods to incorporate the functional ingredients into foods. Curcumin (1,7-bis(4-hydroxy-3-methoxyphenyl)-1,6-heptadiene-3,5-dione) is the best characterized plant-derived polyphenol showing chemopreventive and safety activities against malignancy.[Citation1] This yellow-orange natural compound, which is the main component of Curcuma longa rhizomes (),[Citation2] has been consumed as dye and food additive for a long time period in Asia. In the past decade, scientific studies have revealed other medicinal effects of curcumin, including antiproliferative, antiangiogenic, anti-inflammatory, anti-cystic fibrosis, antioxidant, and wound healing properties.[Citation3−Citation6] It is of special importance among multitarget components in cancer chemotherapy. Today, it is also used in curry as spice, and as a preservative and industrial food dye (E-100).
FIGURE 1 (Top, left) Curcuma longa with flower and rhizome plus dried turmeric. Turmeric (Curcuma longa L.), belonging to the family of Zingiberaceae, is a perennial herb native to India where its rhizome is used as a yellow colorant curry spice and traditional medicine. (Top, right) Chemical structure of curcumin. The active principle in turmeric was identified as a group of polyphenolic compounds, namely curcumin (74–78%), demethoxycurcumin (15–18%), and bisdemethoxycurcumin (4–6%) commonly referred to as “curcumin.” (Bottom, Left) One of the proposed representations of the model of casein micelles. (Bottom, Right) Ribbon representation of modeled BSA and the locations of domain-binding sites. The locations of hydrophobic binding sites (Sudlow I and Sudlow II) are indicated. The protein secondary structure is shown as ribbon with the sub-domains color coded as follows: IA, blue; IB, sky blue; IIA, green; IIB, yellow; IIIA, orange; IIIB, red. This color scheme is maintained throughout the article. Final conformation obtained after energy minimization has been prepared using PyMOL software. For interpretation of the references to color in this figure legend, the reader is referred to the web version of this article.
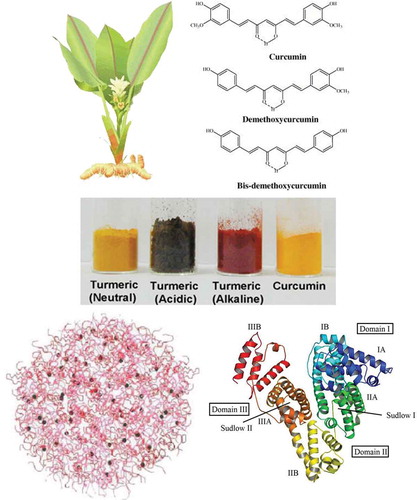
Curcumin is extremely safe even at very high doses of 8–12 g/day. But, from the chemical viewpoint, there are several challenges that must be overcome for curcumin to become a routine treatment agent. Despite the safety, efficacy, and well-tracked mechanisms of action, curcumin has a low aqueous solubility (approximately 11 ng/mL), which significantly limits its bioavailability in vivo.[Citation4] On the other hand, in aqueous medium, curcumin undergoes rapid degradation by hydrolysis (even at physiological pH) followed by molecular fragmentation within 30 min.[Citation7,Citation8] Study on the curcumin chemical degradation is an interesting research topic due to the effects of its degradation products on human health and also possible changes in the color and organoleptic properties of food products in which curcumin is used as an additive. In addition, poor solubility/bioavailability and also instability of curcumin have limited its application as health-promoting agent. Various approaches have been applied to increase water solubility, stability and bioavailability of curcumin (as well as its controlled delivery at or around cancer tissues) such as emulsification, chemical modification, and encapsulation in polymer nanoparticles, cyclodextrins, hydrogels, surfactants micelles, lipid bilayers, and vesicles and other delivery systems so that the water solubility of curcumin in cyclodextrin-based or chemically modified systems can reach about 2-200 mg/ml.[Citation9,Citation10] However, each delivery system may have cytotoxic effects on some normal tissues and cells and/or display limited influence on curcumin stability.
Drug delivery systems based on proteins hold much promise because of their high nutritional value and excellent functional properties, including emulsification, gelation, foaming and water binding capacity as well as their applications as ingredients in the food industry. Food protein networks have the ability to interact with a wide range of active compounds via functional groups on their polypeptide primary structure, thus offering a variety of possibilities for reversible binding of active molecules and for protecting them until their release at the desired site within the body. The main aim of the current study is to introduce albumin and casein () as food-grade amphiphilic materials to interact with curcumin, since serum albumins and caseins are commonly used transporting vehicles for proteins, hormones, drugs, and diagnostic agents.[Citation11] Casein micelles are relatively easy to prepare, biodegradable, and have potential for high drug loading capacity. Serum albumin is the most abundant of the proteins, circulated several times in the blood.[Citation12] Both casein micelles and albumin delivery systems have been previously employed for administration of several hydrophobic drugs.[Citation13,Citation14] In the literature, there are a few reports on the interaction of curcumin with casein and albumins[Citation2,Citation15] and its potential delivery to tumor cells,[Citation2] implying that these proteins have the ability to soulobilize and stabilize curcumin in aqueous medium.[Citation2,Citation4,Citation16,Citation17] This implication is consistent with the results by Wang et al.[Citation7] indicating that degradation of curcumin is suppressed in the presence of serum. Since proteins are generally amphiphilic polymers, we hypothesis that albumin and casein (micelle) are able to bind/encapsulate curcumin and improve its stability.
Chemical modification induces structural changes in proteins and exposure of previously buried hydrophobic patches or rendering groups prone to H-bond formation, affecting the binding of hydrophobic and hydrophilic compounds, respectively.[Citation18,Citation19] In this way, we characterized the responsible interactions of curcumin binding to the native and modified proteins. Since, half life is an important factor of curcumin efficacy, in this study; we also reported the mechanism of the enhanced stability of curcumin upon hydrophobic interaction with proteins. Furthermore, we demonstrated that stability enhancement of curcumin in the presence of proteins, originates in part from its stronger binding (high Kb) to the protein. Finally, bound, encapsulated (and stabilized) curcumin exhibited increased anti-cancer activity in vitro.
MATERIALS AND METHODS
Materials
Bovine serum albumin (essentially free fatty acids) fraction V was from Applichem, pure curcumin was from Merck (Darmstadt, Germany) and whole casein and 1-anilinonaphtalene-8-sulfonate (ANS) were purchased from Sigma Chemical Co. (St. Louis, Mo, USA). The other reagents were from analytical grade. All of the experiments were carried out in 50 mM sodium phosphate, pH 7.0 as the buffer in triplicate.
Bovine Serum Albumin (BSA) Modification with Acetic Anhydride
BSA solution (10% w/v) was made in distilled water at 30°C and the pH was adjusted to 7.5–8.5. Acetic anhydride was added to the solution at the level of 0.5 g/g of protein over 30–90 min.[Citation20] Then it was dialyzed against 50 mM phosphate buffer pH 7.0 for 24 h and the buffer was exchanged four times at 6 h intervals.
Casein Modification with Glyceraldehyde
Casein concentrate (150 μM) was incubated overnight at 37°C in glyceraldehyde aqueous solution (3 mM), which was buffered with 50 mM phosphate buffer pH 7.0. At the end, 100 μl of a 0.1 M NaBH4 solution in 0.1 N NaOH was added and the mixture was further incubated for 1 h. After cooling under room temperature condition, the modified proteins were dialyzed against 50 mM phosphate buffer pH 7.0 for 24 h and the buffer was exchanged four times at 6-h intervals.[Citation21] Free amino groups in both modified proteins were assessed by the TNBS method for determination of the lysine modification extent, as described elsewhere.[Citation22]
Curcumin Hydrolytic Stability Measurement
A solution of 1 mM curcumin in methanol was used as a stock solution, 30 μL of which was added to 3 mL of the phosphate buffer solution without proteins to give 10 μM curcumin concentration. In this concentration, aggregation of curcumin appeared to be negligible. Solutions of 1 mg/ml native and modified BSA and 0.33 mg/ml native and modified casein were also prepared in buffer solution. Curcumin stock solution (30 μL) was added to 3 mL of each of protein solutions to achieve ˜1:1.5 molar ratio of curcumin to protein. Absorbance spectra were recorded from 300 to 600 nm over 60 min at 5-min intervals using a PerkinElmer UV-vis spectrophotometer at 30°C. The hydrolytic stability of the curcumin was followed at the absorption maximum (420 nm) versus time. The rate of degradation is determined from the initial slope of the best-fit linear curve.
PSH Determination
Suitable probes, such as ANS, may be help to monitoring of changes in protein surface hydrophobicity.[Citation23] Changes in protein surface hydrophobicity (PSH) upon modifications can be detected by following the protein-ANS binding characteristics or extent of fluorescence enhancement through titration of protein solution with probe. ANS from 4 mM stock solution was added to a final concentration range from 0.25 to 35 (BSA) or 140 (casein) μM. The protein final concentration was 0.3 mg/ml in 3 ml quartz cuvette. The increment in the fluorescence emission with excitation at 380 nm was recorded from 400 to 600 nm until no further increase was observed in fluorescence. Also, the observed fluorescence should be correct for dilution. This correction normalizes the fluorescence to constant volume and protein concentration.
Intrinsic Steady-State Fluorescence Measurements
Fluorescence emission spectra of 0.067 mg/ml native/modified BSA and 0.02 mg/ml native/modified casein in the absence and presence of the various concentrations of curcumin were separately recorded on a Cary Eclipse (Varian) spectrofluorometer equipped with multicell holder and Peltier temperature control. The excitation and emission wavelengths were set at 290 and 300–450 nm, respectively.
Determination of Binding Constant and Number of Binding Sites
The modified Stern-Volmer equation can be used for the relationship explanation between the extent of fluorescence quenching of the macromolecule and the quencher concentration (Eq. 1). Since this equation is correct that small molecules bind independently to a set of equivalent sites on a fluorescent macromolecule:
Thermodynamic Analysis of the Binding Process
The thermodynamic parameters, enthalpy (ΔH°) and entropy (ΔS°), of reaction are prominent for illustrating binding mode. For obtaining these parameters, the temperature-dependence of the binding constant was surveyed. A plot of ln K versus 1/T gives a straight line according to the Van’t Hoff equation (Eq. 2):
Site-Specific Drug Competitive Displacement Experiments
To determine the binding site(s) of curcumin in proteins, site specific probes (drugs), indomethacin, furosemide, and phenylbutazone has been used, which are known to bind to drug site I of subdomain IIA of SA. The concentration of indomethacin, furosemide, and phenylbutazone were 35, 65, and 100 μM, respectively, which are further than their respective Km for BSA. After 10 min incubation of drug with BSA, curcumin (10 μM) was added and then degradation of curcumin was monitored by absorption at 420 nm and emission at 510 nm.
Fluorescence Resonance Energy Transfer (FRET)
Fluorescence emission was recorded at 25°C on a Cary Eclipse spectrofluorometer. The bandwidth of both excitation and emission slits were 5 nm. The fluorescence quenching experiments were carried out by adding curcumin to BSA solution to 1:1 molar ratio in phosphate buffer. Also, the BSA emission and curcumin absorption spectrum was separately recorded at equal concentrations. When the fluorescence emission spectrum of the donor and absorption spectrum of the acceptor have suitable overlap and the donor and the acceptor are within the characteristic Förster distance, energy transfer occurs. The energy transfer efficacy, E, is assessed by Eq. (4):[Citation24]
Molecular Docking and Accessible Surface Area Calculations
The crystal structure of native BSA (PDB 3V03) was downloaded from the protein data bank (PDB) (www.pdb.org) as a template for modeling the modified BSA. An accessible residue will have a relatively fast modification rate, whereas a buried residue will have a relatively slow modification rate. For this study, we chose lysine surface residues, which have >30% accessible surface area assessed in Swiss-PDB Viewer (version 4.0.1).[Citation26] Theoretical modification and the atomic coordinates of curcumin was built using Hyperchem program (version 8.0) in PDB format.[Citation27,Citation28] The native and modified proteins were selected for docking process to study the sildenafil interaction. The optimized structure of ligand was used as input of Auto Dock Tools and the partial charges of atoms were calculated using Gasteiger–Marsili procedure. Using AutoGrid tools, the grid maps were generated adequately large to include the binding site(s) of protein as well as significant regions of the surrounding surface. In all cases, a grid of 100 × 82 × 90 points in the x-, y-, and z-axis directions and a grid spacing of 0.397 Å was applied in each Cartesian direction. Then, the most suitable structure for the flexible ligand molecule was optimized by the rotation of all single bonds in the ligand molecule. The grid parameter file and the docking parameter file were set up by the AutoDock Tools program. Population size was 256 and a maximum number of energy evaluations of 2,000,000 were used. Default settings were used for all other parameters.[Citation29] The ligand molecule for the docking calculations was obtained from Drugbank database.[Citation30] Docking calculations were carried out with the rigid native and modified protein and a flexible ligand using a Lamarckian genetic algorithm.[Citation31] At the end of the docking simulation, the BSA-curcumin complex with the most stable energy was adopted as the most favorable structure of the complex for native and modified BSA. Among all energy minima conformers, the global minimum of conformers were transferred into Python Molecular Viewer (version 1.5.4) program package and Solvent Accessible Surface (SAS) and Solvent Excluded Surface (SES) calculations were performed using Python Molecular Viewer program with the help of the MSMS package (probe radius of 1.5 Å).[Citation32] Molecular graphics were prepared with PyMOL version 0.99 beta06 (http://www.pymol.org) and molecular surface representation was calculated with the MSMS package running from Chimera.[Citation33]
RESULTS AND DISCUSSION
Curcumin, with considerable pharmaceutical activity, is a hydrophobic polyphenolic compound with low water solubility and stability.[Citation1,Citation7] Hence, curcumin requires a convenient carrier system to deliver to different parts of the body. Recently, various systems, such as encapsulation in nanoparticles, cyclodextrins, micelles, and proteins, are being expanded for drug delivery investigations.[Citation4,Citation9] In this investigation, the interaction of curcumin with the native and modified forms of two important proteins, serum albumin and milk casein, (and their effects on curcumin stability and anticancer property) have been studied.
UV-Visible Absorption and Fluorescence Spectra of Curcumin
Curcumin shows weak absorption in the UV-vis region (in phosphate buffer) with a maximum at ˜412 nm, as shown in (solid curves). Absorption intensifies in the presence of proteins and λmax shifted to 418, 428, 427, 422, and 420 nm in the case of lipid transfer protein (LTP), native and modified BSA, native and modified casein solutions, respectively. Furthermore, the UV-vis absorption spectra of curcumin in the presence of LTP and modified casein displayed a shoulder around 355 nm (as indicated by solid arrows in ). These results imply that curcumin is present in the aqueous phase,[Citation4,Citation34] and this is further supported by other experimental results, as will be shown below. As a preliminary conclusion, it appears that LTP and modified casein could not protect curcumin from water-mediated degradation. However, the absence of shoulder in the cases of the native and modified BSA and native casein, suggests that curcumin experiencing different environment (with decreased water-curcumin interaction), which is supported by the next results (on degradation of curcumin), as follows.
FIGURE 2 UV-vis absorption (solid lines) and fluorescence (dashed lines) spectra of curcumin in (a) 50 mM sodium phosphate buffer pH 7.0 plus (b) LTP, (c) BSA, (d) modified BSA, (e) casein, and (f) modified casein. The concentrations of proteins and curcumin were 15 and 10 μM, respectively.
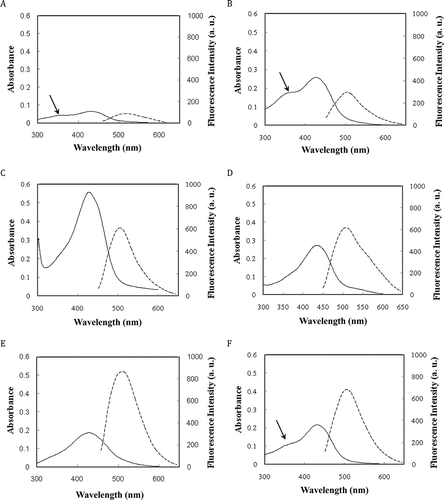
Free curcumin also exhibits weak fluorescence emission at 522 nm in aqueous solutions after excitation at 420 nm (). The fluorescence of curcumin displayed ˜20 nm blue shift in the presence of LTP, native and modified BSA and casein (, dashed curves). Such a significant spectroscopic blue shift can be attributed to the presence of a new local hydrophobic environment generated by the proteins and is generally believed to be due to decrease in the internal relaxation of the fluorophore (curcumin) in a spatially restricted environment.[Citation24] The fluorescence intensity of curcumin in LTP () is similar to that of curcumin in phosphate buffer (), further supporting that curcumin is present largely in the aqueous phase and implying that it is only weakly associated with LTP. The fluorescence intensity of curcumin in phosphate buffer and LTP is less than half of that in BSA and casein, as shown in (dashed curves). Previous studies have shown that the fluorescence yield of curcumin is substantially lower in the polar aqueous environment than in nonpolar surroundings. On the other hand, the high fluorescence yields in BSA and casein imply that curcumin is segregated from the aqueous solvent. The Stokes shifts of curcumin in the presence of proteins also exist in the spectrum of curcumin encapsulated in the ionic micelle, as shown in previous studies, suggesting the local environment of curcumin in “curcumin-protein” systems, similar to the palisade layer of the micelle, where curcumin is located upon encapsulation.[Citation34]
Hydrolytic Degradation of Curcumin
Some of the major challenges in drug delivery to target tissues are their insolubility, instability and biodegradation at physiological pH and temperature.[Citation35] As shown previously, alkaline hydrolysis is the main process in the degradation of curcumin in buffer solution. In addition, the curcumin molecule is unstable at temperatures higher than 30°C (data not shown). It appears that curcumin is partially deprotonated initially, which is followed by fragmentation into trans-6-(40-hydroxy-30-methoxyphenyl)-2,4-dioxo-5-hexanal, as determined by HPLC and mass spectrometry. This product is then further decomposed into smaller molecules such as vanillin, feruloyl methane, and ferulic acid. In contrast to intact curcumin, these molecules contribute negligibly to the absorption of 420 nm light.[Citation4] In addition, we have also established that proteins exhibit negligible absorption at this wavelength. Therefore, decrement in absorbance at 420 nm is mainly reminiscent of decrease in curcumin concentration, solely. In current study, the kinetic of curcumin degradation was investigated in phosphate buffer alone, buffer plus native and modified BSA, buffer plus native and modified casein, and buffer plus LTP. In phosphate buffer solution, the degradation is accompanied by a substantial decrease in the UV-vis absorption intensity that was also confirmed by fluorescence analyses (data not shown). shows the decrease at the absorption maximum, which decays to approximately 30.77% of the initial value in 60 min (in phosphate buffer pH 7 at 30°C). This progressive decrease of the 420 nm absorption intensity is accompanied by the intensification of absorption at 355 nm. In agreement with and , a considerable degradation of curcumin is also present in the LTP solution (), where the absorption at maximum decreases to roughly ˜47% of its original value. It is noteworthy that LTP contains a hydrophobic tunnel, and has been recently considered as a possible drug delivery vehicle. In contrast, the curcumin decays are negligible in the presence of native and modified BSA and native casein, as shown in , , and , respectively. These results clearly highlight the intrinsic ability of BSA and casein to stabilize curcumin.
FIGURE 3 Decreasing UV-vis absorption spectra of curcumin in (a) 50 mM sodium phosphate buffer pH 7.0, and in the presence of (b) LTP, (c) BSA, (d) modified BSA, (e) casein, and (f) modified casein. The concentrations of proteins and curcumin were 15 and 10 μM, respectively. The insets show the decay of curcumin at the absorption maximum (420 nm) vs. time. Each data point was the mean of three independent observations and their S.D. was in the range of 0.82–3.74%.
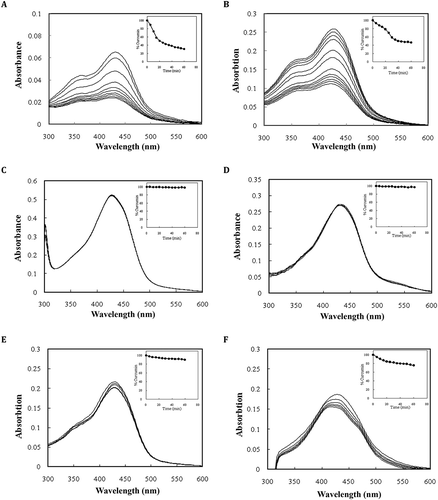
Insets of illustrate the time dependent decays of curcumin in the buffer and protein solutions, which shows linear behavior and hence fits to a pseudo-zero-order model, in agreement with previous reports.[Citation4,Citation34] At pH 7.0, curcumin degrades initially at a rapid rate of 2.562% min−1 for the first 20 min in phosphate buffer solution and then the rate of degradation decreases drastically, (which is) showing a biphasic behavior. In the presence of the native and modified BSA and native and modified casein, however, the rates of degradation are suppressed to 0.045, 0.109, 0.306, and 0.848% min−1, respectively. The time dependent decay kinetics for LTP is similar to this for curcumin in buffer solution, (which is) exhibiting a biphasic characteristic with a fast decay followed by a slow degradation. The fastest decay rate belongs to LTP (1.087% min−1).
The ratio of degradation rates in phosphate buffer and protein solutions indicates that the rate of curcumin degradation decreases in the presence of proteins. The relative reductions in degradation rates were approximately 56.93, 23.51, 8.37, 3.02, and 2.36 in the presence of the native BSA, modified BSA, native casein, modified casein, and LTP, respectively. Suppression of the curcumin degradation by the native and modified BSA was reported as 98.24 and 95.75%, respectively. Also, the suppression yields of degradation by the native and modified casein are 88.06 and 66.90%, respectively. The inhibition of the rapid degradation of curcumin at physiological pH results in an important question: What is the primary reason for the potency of BSA and casein in suppressing of the curcumin degradation? In addition to “hydrophobicity”, there is a possibility that this protection originates from strong binding of the curcumin to the protein vehicles. To test this possibility surface hydrophobicity of proteins and the stability of curcumin-protein complexes were evaluated.
Protein Surface Hydrophobicity Changes upon Protein Modification
Since ideal drug carriers are expected to have protective effects against water-mediated degradation, native, and modified forms of albumin/casein were selected to study in detail and LTP was excluded from further studies. There are several methods for determining of the proteins surface hydrophobicity. ANS is a widely used hydrophobic probe for proteins.[Citation23,Citation35] The fluorescence quantum yield of free ANS is low in water, when it places in hydrophobic medium its fluorescence intensity increases accompanying with a blue shift.[Citation25,Citation34] Thus, ANS can be applied for following of the changes in protein surface hydrophobicity which was induced by ligand binding, chemical modification, mutation, and etc. At a fixed concentration of native/modified proteins, fluorescence intensity of increasing concentration of ANS was measured (). ANS has fixed maximum fluorescence intensity at 470 nm in the presence of the native and modified BSA, but equal concentration of ANS has about 4-fold higher fluorescence intensity in the presence of native BSA than modified form ( and ). This means that surface hydrophobic patches for ANS binding decreases in the modified BSA. The calculated PSH and KbANS values (data not shown) are in full agreement with the above results.
FIGURE 4 Titration of (a) native BSA, (b) modified BSA, (c) native casein, and (d) modified casein with increasing concentration of ANS dye in 50 mM sodium phosphate buffer pH 7.0. ANS was excited at 380 nm and the increase in its fluorescence emission was recorded at 470 nm. Data shown are representative of three similar independent experiments.
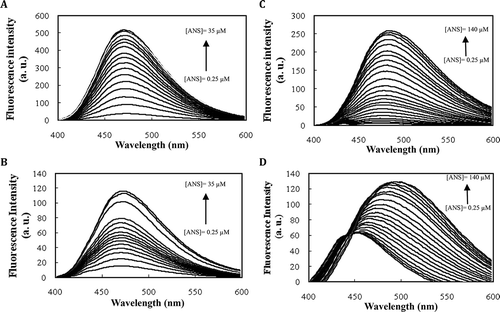
In the presence of native casein, ANS has fixed λmax at 480 nm, whereas, λmax of ANS red shifted from 445 to 495 nm by increasing ANS concentration ( and ). This illustrated that ANS binds to modified casein at multiple dependent binding sites as binding of one ANS causes structural change that affects the nature of other binding sites and results in increased polarity of them. However, fluorescence intensity of identical concentration of ANS in the presence of the native casein is two-fold higher than that of the modified form, which demonstrates decrement of surface hydrophobicity of casein upon modification ( and ).
Hydrophobic interaction is believed to play a crucial role in curcumin-native BSA interaction. There is the possibility that increased protein hydrophobicity affects albumin-curcumin interaction. To test this possibility, charge modification of lysine residues was achieved so that positive charges of the lysine ϵ-amino groups were changed to more apolar moieties by acetylation. Therefore, we decided to modify accessible lysine side chains of the protein by acetic anhydride. Contrary to the expectation, the surface hydrophobicity of BSA is drastically was decreased upon lysine acetylation. Furthermore, according to results of this investigation, the essence of curcumin-BSA interaction has not been altered upon modification, which may also corroborate this assumption that curcumin binding sites are the same in the native and modified BSAs.
There is a linear relationship between the surface areas of amino acid residues and free energy changes related to the transfer of the amino acids from water into protein interior (an apolar environment).[Citation36] The degree of flexibility of the protein structure can be quantitatively estimated by calculating solvent accessible surface area (SASA). As depicted illustratively in , significant SASA decrement, upon BSA modification, is in agreement with PSH data and shows induction of some degree of compactness in the protein conformation.
FIGURE 5 The hydrophobic surfaces of (a) native and (b) modified BSA. The surface hydrophobicity was shown with colors ranging from dodger blue for the most hydrophilic to white at 0.0 and orange red for the most hydrophobic; the images are made with UCSF Chimera and the surface was calculated with the MSMS package. PDB ID: 3v03. Since there is no crystallographic data on casein 3D structure, we brought only the BSA data in this figure.
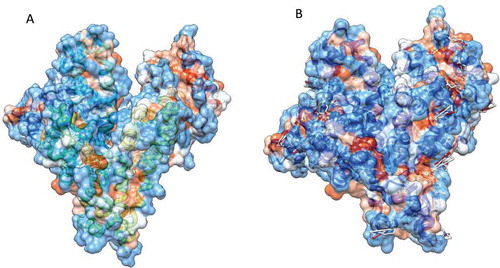
Interaction between Curcumin and Proteins
Fluorescence quenching of (macro-)molecules gives useful details about ligand binding to specific sites on macromolecule.[Citation37] So, fluorescence quenching experiments of native/modified proteins were carried out at 293, 298, 303, and 308 K. shows the fluorescence quenching spectra of proteins in the presence of curcumin at 293 K. The native and modified proteins exhibit a strong fluorescence emission at ˜345 nm upon excitation at 290 nm. Since curcumin contains hydroxylic functional groups () and its interaction with polar side chains on proteins is expected, we decided to modify casein lysine side chains with a hydroxyl-containing modifier, such as glyceraldehyde. The modification of casein with glyceraldehyde led to formation of new fluorophores with significant fluorescence between 400 and 500 nm (may be due to formation of chromogenic end products).[Citation25] Addition of curcumin to the protein solutions then caused quenching of fluorescence intensity with the formation of a new peak at 510 nm (data not shown). This indicates an interaction between the ligand and the proteins and it is also suggested that curcumin is located at the vicinity of the tryptophanyl side chains. This may be attributed to a Foerster type energy transfer from the fluorophores (Trps) to the quencher (curcumin). The appearance of a new isosbestic point also demonstrates a simple equilibrium binding system (data not shown).[Citation38]
FIGURE 6 Curcumin-mediated quenching of the native BSA (a), modified BSA (b), native casein (c), and modified casein (d) fluorescenc at 293 K in 50 mM sodium phosphate buffer pH 7.0. Each data point was the mean of three independent determinations and their S.D. was in the range of 1.28–4.24%.
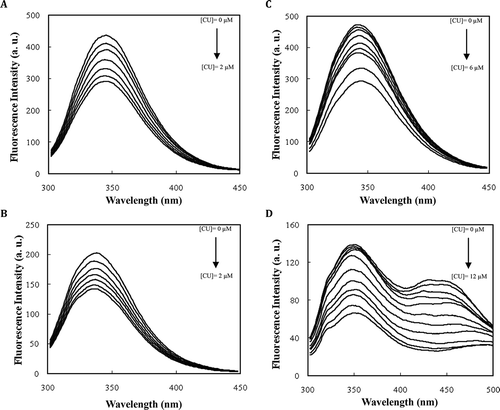
Fluorescence measurements can be used to assess the binding constant (Kb) and average number of binding sites (n) of the curcumin-protein complex by using modified Stern-Volmer equation.[Citation24,Citation29] Plot of log[(F0−F)/F] versus log[Q] gives the values of Kb and n (). The value of Kb is essential for estimation of efficiency of the drug carrier systems, since weak binding may result in a short half-life or poor distribution, while strong binding can decrease the concentrations of free drug in body fluids.[Citation39] The proteins exhibit high binding constants on the order of 106 M−1 (). The values of the binding constants for the association of curcumin to the native forms of BSA and casein agree with the obtained values from the previous studies.[Citation2,Citation15] The n values at different temperatures are kept around unity, which shows the existence of only one binding site both on the native and modified albumin.
TABLE 1 Average number of binding site (n) and binding constants (Kb) for interaction of curcumin with native and modified forms of BSA and casein at 293 K
FIGURE 7 Double-logarithmic plot of the curcumin quenching effect on the native (•) and modified (○) forms of BSA (a) and casein (b) fluorescence at 293 K in 50 mM sodium phosphate buffer pH 7.0. Kb and n values are obtained from the y-intercepts and slopes of the plots, respectively.
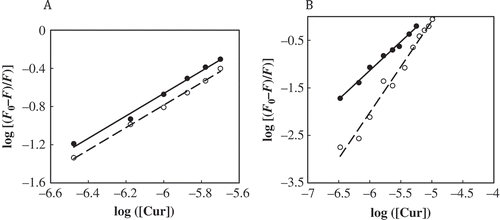
As it can be observed (), Kb decreases upon modification indicating lower stability of curcumin-modified BSA complex, in agreement with PSH data. Reciprocally, different observations were made in the case of casein. Taking the above observations into account, it is reasonable to assume that loose binding of the ligand to the modified BSA plays at least partially a causative role in the increased rate of curcumin degradation, which confirms that the modified BSA is not good drug delivery system because of its lower protective effect against hydrolytic curcumin degradation.
Mohammadi et al.[Citation15] have suggested that hydroxyl phenolic groups have an important role in the curcumin binding process. Hence, modified casein (with novel hydroxyl groups) is prone to H-bond formation with hydroxyl phenolic groups of curcumin. Despite a decrease of casein surface hydrophobicity upon glycation-like modification, increased Kb value suggests that the stability of curcumin-casein complex has increased upon modification. The results indicated that not only Kb of “curcumin-casein” system but also n values increase in the case of the modified casein. Nevertheless, extreme increment in Kb and n is associated with decreased protection ability of casein against curcumin degradation. More importantly, it possibly seems that protective effects of casein against hydrolytic degradation of curcumin originate from factors other than stability of “drug-albumin” complex. These findings illustrated that the native casein as a natural biopolymer can be a good matrix for increasing of the curcumin stability in water, whereas the modified casein may not be a suitable drug carrier.
Competition between Curcumin and Site-Marker Drugs
In order to further substantiate curcumin binding site on BSA, site-specific competitive experiments were scrutinized using drugs, which specifically bind to a known site or domain. Serum albumin is composed of three homologous domains (I, II, and III); each domain in turn is divided into two sub-domains, A and B abundantl.[Citation40] Albumins (BSA, HSA) possess two main drug-binding sites designed as Sudlow site I and Sudlow site II. They are located in two hydrophobic cavities of subdomains IIA and IIIA, respectively. It was shown that Sudlow site I prefers large heterocyclic and negatively charged compounds, while Sudlow site II is the preferred site for small aromatic carboxylic acids.[Citation41] Other highly specific binding sites are a binding site for polycyclic aromatic hydrocarbon epoxides, in subdomain IB, and as many as six sites for fatty acids.[Citation42] In addition, there are a number of minor binding sites allowing simultaneous binding of multiple drugs on HSA.
In this work, site specific competitor probes (drugs), including indomethacin, furosemide, and phenylbutazone, have been used to investigate the curcumin binding site(s) in native/modified BSA. Indomethacin, furosemide, and phenylbutazone are known site markers that mainly bind to drug site I, in subdomain IIA.[Citation43] As can be deduced from , the rate of curcumin degradation increases in the presence of indomethacin confirming a relative competition between indomethacin and curcumin so that indomethacin displaces curcumin both in the native and modified BSAs. Furosemide partially competes with curcumin, whereas phenylbutazone has no effect on curcumin degradation. Phenylbutazone and furosemide binding site are located in subdomain IIA (drug binding site I), while indomethacin has either a secondary binding site in subdomain IB.[Citation25] These results may corroborate the idea that curcumin competes with indomethacin for only its secondary binding site (located in subdomain IB), which has been previously proposed by docking studies[Citation44] or for its main specific binding site (indomethacin subsite)[Citation25] not AZT subsite and SAL subsite in Sudlow site I.
FIGURE 8 Degradation of curcumin in the absence (•) and the presence of phenylbutasone (◊), furosemide (▴), and indomethacin (○) for the native (a) and modified (b) BSA studied by UV-vis technique in 50 mM sodium phosphate buffer pH 7.0. Each data point is the mean of three independent observations and their S.D. was in the range of 1.12–4.81%.
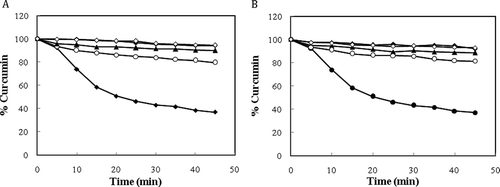
Fluorescence Energy Transfer between Proteins and Curcumin
Quenching of the BSA and casein fluorescence upon their binding to curcumin leads to formation of a new peak at 510 nm, which shows the occurrence of energy transfer between ligand and proteins. Efficiency of energy transfer is determined by the expanse of overlap between the emission spectrum of the donor (protein) and the absorption spectrum of the acceptor (ligand), and the relative orientation and distance of the donor and acceptor transition dipoles.[Citation45] Therefore, to accurately determine the binding site of curcumin, fluorescence resonance energy transfer experiment was used. As observed in , there was a good overlap between the emission spectra of equimolar concentrations of native/modified BSA and the UV absorption spectrum of curcumin. The J values were calculated by integrating the overlap area of the spectrum from 302 to 500 nm and were found to be 4.897 × 10−14 M−1 cm3 and 4.914 × 10−14 M−1 cm3 for the native and modified BSA, respectively. Using, κ2 = 2/3, n = 1.33, and QD = 0.15,[Citation46] R0 were 2.551 and 2.560 nm for the native and modified BSA, respectively. The Förster distance (R0) and the distance between the donor and the acceptor (r0) obtained are well in accordance with previous studies.[Citation47] Insignificant changes in obtained “r” values for the native and modified BSA were similar (2.040 and 2.191 nm, respectively, using E = 0.738 and E = 0.607 calculated from fluorescence data), and confirm that the curcumin binding site on BSA has not changed upon modification.
FIGURE 9 Spectral overlap between the fluorescence emission spectrum of protein (solid curves) and absorption spectrum of curcumin (dashed curves). (a) Native BSA and (b) modified BSA in 50 mM sodium phosphate buffer pH 7.0. [Curcumin]:[BSA] = 5 μM; λex = 290 nm.
![FIGURE 9 Spectral overlap between the fluorescence emission spectrum of protein (solid curves) and absorption spectrum of curcumin (dashed curves). (a) Native BSA and (b) modified BSA in 50 mM sodium phosphate buffer pH 7.0. [Curcumin]:[BSA] = 5 μM; λex = 290 nm.](/cms/asset/82f17b79-5dee-448f-a88c-4a9ee44825d7/ljfp_a_853185_f0009_b.gif)
The relative smaller “r” values as well as larger “E” values suggested the significant interaction between this natural compound and BSA. In other words, the donor-to-acceptor distance lies within the limits of 0.5 R0 to 2 R0 revealing effective energy transfer[Citation24] and implies that one or two Trp residues are in close proximity to curcumin. It is revealed that Trp214 is found in a hydrophobic cavity on subdomain IIA whereas Trp134 is placed near the surface of the molecule in subdomain IB.[Citation12,Citation47] Docking simulations carried out by Kudva et al.[Citation44] indicated the presence of a single curcumin binding site on HSA, located away from Trp214, on domain IB.
Mode of Curcumin Binding to BSA and Casein
Essentially, there are four types of non-covalent interactions that could play a key role in drug binding to proteins, including hydrogen bonds and van der Waals interactions, electrostatic forces, and hydrophobic interactions. In order to elucidate the binding mode, binding constant (Kb) thermodynamic parameters, ΔH° (enthalpy change) and ΔS° (entropy change), are often obtained. Ross and Subramanian[Citation48] have characterized the sign of the thermodynamic parameters associated with various types of interactions.
The temperature-dependent experiments were done to obtain the thermodynamic parameters of curcumin-protein interactions. The values of ΔH° and ΔS° are raised from the slope and y-intercept of the Van’t Hoff plots, respectively, and are listed in . A negative value of ΔG° for all complexes supports the spontaneous nature of the curcumin binding process.
TABLE 2 Thermodynamic parameters for binding of curcumin to native and modified proteins at 293 K
When we applied above analyses to the binding systems of curcumin-albumin, the positive values of ΔH° and ΔS° were obtained, indicating that hydrophobic interactions are the major forces in binding of curcumin to both native and modified albumins.[Citation48] Moreover, although the results of drug displacement, FRET, and binding force determination suggest that the curcumin binding sites on both native and modified BSA are the same, but it seems that the reduced PSH leads to weaker binding of curcumin to the modified BSA.
On the other hand, based on obtained positive values for both ΔH° and ΔS°, hydrophobic interactions are the main forces in binding of curcumin to the native casein, whereas, according to ΔH° < 0, curcumin binding to the modified casein is enthalpy driven and the major binding forces are hydrogen bounds and/or van der Waals interactions.[Citation48,Citation49] There is the possibility that rigorous (glyceraldehyde-mediated) modification of casein disrupt its micellular structure. At these conditions, water molecules can penetrate into the modified casein structure, therefore leading to decrement of half-life of bound curcumin molecules via water-mediated degradation processes (see ). To investigate this objective, curcumin degradation rate has been evaluated in the presence of two polarity reducing compounds, ethylene glycol (EG) and polyethylene glycol 6000 (PEG). The results showed that these two compounds have similar protective effects so that they reduced curcumin degradation rate by almost 10-fold (86 and 98%, respectively). This is probably due to the weak and dynamic hydrophobic interactions between the exposed hydrophobic regions of polarity reducing agents and curcumin (data not shown).
Docking Studies of Curcumin-BSA Complexes
In order to reconfirm experimental observations, molecular docking studies of the native/modified BSA-curcumin complexes were conducted. The docking results of curcumin with the native and modified BSA are shown in , indicating that the primary binding site for curcumin in BSA is located in subdomains IB, closer to the tryptophan residue 134, in agreement with the results of the competitive displacement experiments. However, it appears that hydrophobic interaction plays a major role in the curcumin-BSA binding reaction (see thermodynamic analyses, ). Additionally, the calculated binding distances between curcumin and Trp134 in the native and modified BSA were 2.43 and 2.81 nm, respectively, which is similar to the apparent (mean) “r” values reported earlier (2.040 and 2.191 nm for native and modified BSA, respectively).
FIGURE 10 Binding of curcumin to subdomain IB of (a) native (PDB: 3V03) and (b) modified BSA. The protein backbone is shown in the “cartoon” representation, and three histidines and Trp residues in the active site are shown in the “stick” representation. Final conformation obtained after energy minimization and docking has been prepared using Hyperchem, Autodock, and PyMOL software, respectively.
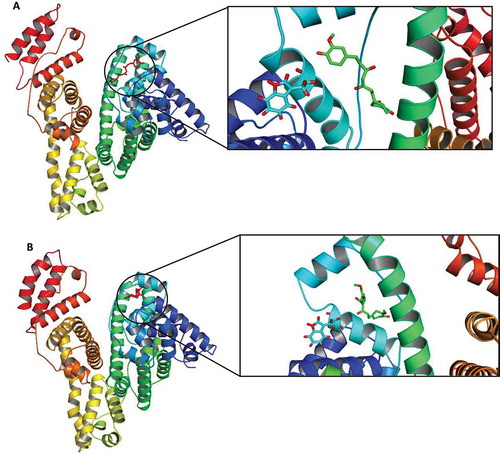
The interaction of curcumin with different biomacromolecules could have strong consequences on its activity in biological systems.[Citation50] According to previous reports on cytotoxicity effects of curcumin on cancer cells,[Citation2,Citation50,Citation51] we also evaluated the efficacy of protein delivery systems on two cancerous cell lines of human origin (MCF7, and SKNMC plus a normal cell, HUVEC, data not shown). In agreement with stability data, curcumin in the presence of native (not modified) proteins displayed higher cell-killing activity indicating that control of degradation rate of curcumin may play an important role in its cytotoxic properties. Additionally, increased curcumin degradation rate in the presence of the modified casein (with additional HO- groups) may originate from water-mediated hydrogen bonds between protein and curcumin.
The increased stability enhances lifetime and gives an additional opportunity to intact/functional curcumin to be up taken by target cells. Moreover, binding of curcumin to proteins may lead to its controlled release. This can affect the distribution and pharmacokinetics of curcumin. In a phase I trial, the concentrations of curcumin in plasma and target tissues were as low as 11.1 nM and 1.3 μM, respectively, even with an oral administration of 3.6 g/day.[Citation8,Citation52] This poor bioavailability may be due to degradation of curcumin in gastrointestinal tract and plasma. This laboratory suggest that mixing of curcumin with milk or its consumption as ethylene glycol containing capsules may lead to its enhanced stability and bioavailability. Finally, enhanced permeation and retention (ESR) of albumin within tumor cells may highlight its application as an important drug delivery system.
In conclusion, although curcumin has potential for extensive use in disease treatment, there are two major challenges that limit this target: (a) Curcumin has low solubility in aqueous solution, which poses a severe limitation on the achievable concentration in biological systems. (b) The water soluble portion undergoes rapid (water-mediated) hydrolytic degradation followed by molecular fragmentation, even at physiological pH. Therefore, due to low stability, ingested curcumin may degrade under physiological conditions, in vivo. The current work shows that serum albumin/native casein as carriers for curcumin has the ability to stabilize curcumin against (water-mediated) chemical degradation of curcumin. Moreover, possible mechanisms which are responsible for stabilizing curcumin against hydrolytic degradation were discussed. Based on some published data,[Citation53,Citation54] there is still a debate on biological activity/efficiency of functional foods and a large segment of the population is reluctant to use them due to ambiguities related to their health claims. But, this article may highlight the role of processed/modified functional foods against various physiological threats.
ABBREVIATIONS
SA: serum albumin; BSA: bovine serum albumin; LTP: lipid transfer protein; TNBS: 2,4,6-trinitrobenzenesulfonic acid; PSH: protein surface hydrophobicity; ANS: 1-anilinonaphthalene-8-sulfonate; FRET: fluorescence resonance energy transfer; PDB: protein data bank; SASA: solvent accessible surface area; LDH: lactate dehydrogenase; MCF7: a breast cancer cell line; SKNMC: a human neuronal epithelioma cell line; HUVEC: human umbilical vein endothelial cell.
ACKNOWLEDGMENT
This work was performed in partial fulfillment of the requirements for Pharm. D. of M. M. Nadi, in the Faculty of Pharmacy, Kermanshah University of Medical Sciences, Kermanshah, Iran.
FUNDING
This work is sponsored by the Research Council of Kermanshah University of Medical Sciences, Kermanshah, Iran.
REFERENCES
- Aggarwal, B.B.; Kumar, A.; Bharti, A.C. Anticancer potential of curcumin: Preclinical and clinical studies. Anticancer Research 2003, 23 (1A), 363–398.
- Sahu, A.; Kasoju, N.; Bora, U. Fluorescence study of the curcumin-casein micelle complexation and its application as a drug nanocarrier to cancer cells. Biomacromolecules 2008, 9 (10), 2905–2912.
- Aggarwal, B.B.; Bhatt, I.D.; Ichikawa, H.; Ahn, K.S.; Sethi, G.; Sandur, S.K.; Sundaram, C.; Seeram, N.; Shishodia, S. Curcumin: Biological and medicinal properties. In: Turmeric: The Genus Curcuma. Taylor and Francis Press: London, United Kingdom, 2007.
- Leung, M.H.; Kee, T.W. Effective stabilization of curcumin by association to plasma proteins: Human serum albumin and fibrinogen. Langmuir 2009, 25 (10), 5773–5777.
- Hsu, C.H.; Cheng, A.L. Clinical studies with curcumin. Advances in Experimental Medicine and Biology 2007, 595, 471–480.
- Cheng, A.L.; Hsu, C.H.; Lin, J.K.; Hsu, M.M.; Ho, Y.F.; Shen, T.S.; Ko, J.Y.; Lin, J.T.; Lin, B.R.; Ming-Shiang, W.; Yu, H.S.; Jee, S.H.; Chen, G.S.; Chen, T.M.; Chen, C.A.; Lai, M.K.; Pu, Y.S.; Pan, M.H.; Wang, Y.J.; Tsai, C.C.; Hsieh, C.Y. Phase I clinical trial of curcumin, a chemopreventive agent, in patients with high-risk or pre-malignant lesions. Anticancer Research 2001, 21 (4B), 2895–2900.
- Wang, Y.J.; Pan, M.H.; Cheng, A.L.; Lin, L.I.; Ho, Y.S.; Hsieh, C.Y.; Lin, J.K. Stability of curcumin in buffer solutions and characterization of its degradation products. Journal of Pharmaceutical and Biomedical Analysis 1997, 15 (12), 1867–1876.
- Liang, G.; Shao, L.; Wang, Y.; Zhao, C.; Chu, Y.; Xiao, J.; Zhao, Y.; Li, X.; Yang, S. Exploration and synthesis of curcumin analogues with improved structural stability both in vitro and in vivo as cytotoxic agents. Bioorganic & Medicinal Chemistry 2009, 17 (6), 2623–2631.
- Canamares, M.V.; Garcia-Ramos, J.V.; Sanchez-Cortes, S. Degradation of curcumin dye in aqueous solution and on ag nanoparticles studied by ultraviolet-visible absorption and surface-enhanced Raman spectroscopy. Applied Spectroscopy 2006, 60 (12), 1386–1391.
- Mohanty, C.; Sahoo, S.K. The in vitro stability and in vivo pharmacokinetics of curcumin prepared as an aqueous nanoparticulate formulation. Biomaterials 2010, 31 (25), 6597–6611.
- Kunwar, A.; Barik, A.; Pandey, R.; Priyadarsini, K.I. Transport of liposomal and albumin loaded curcumin to living cells: An absorption and fluorescence spectroscopic study. Biochim. Biophys. Acta 2006, 1760 (10), 1513–1520.
- Peters, Jr., T., Serum albumin. Advances in Protein Chemistry 1985, 37, 161–245.
- Zamboni, W.C. Liposomal, nanoparticle, and conjugated formulations of anticancer agents. Clinical Cancer Research 2005, 11 (23), 8230–8234.
- Portnaya, I.; Cogan, U.; Livney, Y.D.; Ramon, O.; Shimoni, K.; Rosenberg, M.; Danino, D. Micellization of bovine beta-casein studied by isothermal titration microcalorimetry and cryogenic transmission electron microscopy. Journal of Agricultural and Food Chemistry 2006, 54 (15), 5555–5561.
- Mohammadi, F.; Bordbar, A.K.; Divsalar, A.; Mohammadi, K.; Saboury, A.A. Analysis of binding interaction of curcumin and diacetylcurcumin with human and bovine serum albumin using fluorescence and circular dichroism spectroscopy. Protein Journal 2009, 28 (3–4), 189–196.
- Mitra, S.P. Binding and stability of curcumin in presence of bovine serum albumin. Journal of Surface Science and Technology 2007, 23, 91–110.
- Esmaili, M.; Ghaffari, S.M.; Moosavi-Movahedi, Z.; Atri, M.S.; Sharifizadeh, A.; Farhadi, M.; Yousefi, R.; Chobert, J.M.; Haertlé, T.; Moosavi-Movahedi, A.A. Beta casein-micelle as a nano vehicle for solubility enhancement of curcumin: Food industry application. LWT–Food Science and Technology 2011, 44, 2166–2172.
- Kulmyrzaev, A.A.; Levieux, D.; Dufour, E. Front-face fluorescence spectroscopy allows the characterization of mild heat treatments applied to milk. Relations with the denaturation of milk proteins. Journal of Agricultural and Food Chemistry 2005, 53 (3), 502–507.
- Tayyab, S.; Haq, S.K.; Sabeeha; Aziz, M.A.; Khan, M.M.; Muzammil, S. Effect of lysine modification on the conformation and indomethacin binding properties of human serum albumin. International Journal of Biological Macromolecules 1999, 26 (2–3), 173–180.
- Lawal, O.S.; Adebowale, K.O. The acylated protein derivatives of Canavalia ensiformis (jack bean): A study of functional characteristics. LWT–Food Science and Technology 2006, 39, 918–929.
- Fenaille, F.; Guy, P.A.; Tabet, J.C. Study of protein modification by 4-hydroxy-2-nonenal and other short chain aldehydes analyzed by electrospray ionization tandem mass spectrometry. Journal of the American Society for Mass Spectrometry 2003, 14 (3), 215–226.
- Snyder, S.L.; Sobocinski, P.Z. An improved 2,4,6-trinitrobenzenesulfonic acid method for the determination of amines. Analytical Biochemistry 1975, 64 (1), 284–288.
- Cardamone, M.; Puri, N.K. Spectrofluorimetric assessment of the surface hydrophobicity of proteins. Biochemical Journal 1992, 282 (Pt 2), 589–593.
- Lakowicz, J.R. Principles of Fluorescence Spectroscopy. Kluwer Academic/Plenum: New York, 1999.
- Khodarahmi, R.; Karimi, S.A.; Ashrafi Kooshk, M.R.; Ghadami, S.A.; Ghobadi, S.; Amani, M. Comparative spectroscopic studies on drug binding characteristics and protein surface hydrophobicity of native and modified forms of bovine serum albumin: Possible relevance to change in protein structure/function upon non-enzymatic glycation. Spectrochimica Acta A Molecular and Biomolecular Spectroscopy 2012, 89, 177–186.
- Schwede, T.; Kopp, J.; Guex, N.; Peitsch, M.C. SWISS-MODEL: An automated protein homology-modeling server. Nucleic Acids Research. 2003, 31 (13), 3381–3385.
- Froimowitz, M. HyperChem: A software package for computational chemistry and molecular modeling. Biotechniques 1993, 14 (6), 1010–1013.
- Ghadami, S.A.; Khodarahmi, R.; Ghobadi, S.; Ghasemi, M.; Pirmoradi, S. Amyloid fibril formation by native and modified bovine beta-lactoglobulins proceeds through unfolded form of proteins: A comparative study. Biophysical Chemistry 2011, 159 (2–3), 311–320.
- Goodsell, D.S.; Olson, A.J. Automated docking of substrates to proteins by simulated annealing. Proteins 1990, 8 (3), 195–202.
- Wishart, D.S. DrugBank and its relevance to pharmacogenomics. Pharmacogenomics 2008, 9 (8), 1155–1162.
- Morris, G.M.; Goodsell, D.S.; Halliday, R.S.; Huey, R.; Hart, W.E.; Belew, R.K.; Olson, A.J. Automated docking using a Lamarckian genetic algorithm and an empirical binding free energy function. Journal of Computational Chemistry 1998, 19 (14), 1639–1662.
- Can, T.; Chen, C.I.; Wang, Y.F. Efficient molecular surface generation using level-set methods. Journal of Molecular Graphics and Modelling 2006, 25 (4), 442–454.
- Pettersen, E.F.; Goddard, T.D.; Huang, C.C.; Couch, G.S.; Greenblatt, D.M.; Meng, E.C.; Ferrin, T.E. UCSF chimera—A visualization system for exploratory research and analysis. Journal of Computational Chemistry 2004, 25 (13), 1605–1612.
- Leung, M.H.M.; Colangelo, H.; Kee, T.W. Encapsulation of curcumin in cationic micelles suppresses alkaline hydrolysis. Langmuir 2008, 24, 5672–5675.
- Jarho, P.; Urtti, A.; Jarvinen, T. Hydroxypropyl-beta-cyclodextrin increases the aqueous solubility and stability of pilocarpine prodrugs. Pharmaceutical Research 1995, 12 (9), 1371–1375.
- Samanta, U.; Bahadur, R.P.; Chakrabarti, P. Quantifying the accessible surface area of protein residues in their local environment. Protein Engineering 2002, 15 (8), 659–667.
- Möller, M.; Denicola, A. Study of protein-ligand binding by fluorescence. Biochemistry and Molecular Biology Education 2002, 30, 309–312.
- Sahoo, B.K.; Ghosh, K.S.; Dasgupta, S. Molecular interactions of isoxazolcurcumin with human serum albumin: Spectroscopic and molecular modeling studies. Biopolymers 2009, 91 (2), 108–119.
- Chen, T.; Cao, H.; Zhu, S.; Lu, Y.; Shang, Y.; Wang, M.; Tang, Y.; Zhu, L. Investigation of the binding of Salvianolic acid B to human serum albumin and the effect of metal ions on the binding. Spectrochimica Acta A Molecular and Biomolecular Spectroscopy 2011, 81 (1), 645–652.
- Mikusinska-Planner, A.; Surma, M. X-ray diffraction study of human serum. Spectrochimica Acta A Molecular and Biomolecular Spectroscopy 2000, 56A (9), 1835–1841.
- Sudlow, G.; Birkett, D.J.; Wade, D.N. Further characterization of specific drug binding sites on human serum albumin. Molecular Pharmacology 1976, 12 (6), 1052–1061.
- Brunmark, P.; Harriman, S.; Skipper, P.L.; Wishnok, J.S.; Amin, S.; Tannenbaum, S.R. Identification of subdomain IB in human serum albumin as a major binding site for polycyclic aromatic hydrocarbon epoxides. Chemical Research in Toxicology 1997, 10 (8), 880–886.
- Ascenzi, P.; Bocedi, A.; Notari, S.; Menegatti, E.; Fasano, M. Heme impairs allosterically drug binding to human serum albumin Sudlow’s site I. Biochemical and Biophysical Research Communications 2005, 334 (2), 481–486.
- Kudva, A.K.; Manoj, M.N.; Swamy, B.M.; Ramadoss, C.S. Complexation of amphotericin B and curcumin with serum albumins: Solubility and effect on erythrocyte membrane damage. Journal of Experimental Pharmacology 2011, 3, 1–6.
- Gonzalez, G. Determination of distances between chromophores in proteins. A resonance-energy transfer experiment. Biochemical Education 1994, 22, 150–151.
- Cui, F.L.; Fan, J.; Li, J.P.; Hu, Z.D. Interactions between 1-benzoyl-4-p-chlorophenyl thiosemicarbazide and serum albumin: Investigation by fluorescence spectroscopy. Bioorganic & Medicinal Chemistry 2004, 12 (1), 151–157.
- Barik, A.; Mishra, B.; Kunwar, A.; Priyadarsini, K.I. Interaction of curcumin with humanserum albumin: Thermodynamic properties, fluorescence energy transfer and denaturation effects. Chemical Physics Letters 2007, 436, 239–243.
- Ross, P.D.; Subramanian, S. Thermodynamics of protein association reactions: Forces contributing to stability. Biochemistry 1981, 20 (11), 3096–3102.
- Hua, T.Y.; Liua, C.L.; Chena, J.Y.; Hu, M.L. Curcumin ameliorates methylglyoxal-induced alterations of cellular morphology and hyperpermeability in human umbilical vein endothelial cells. Journal of Functional Foods 2013, 5, 745–754.
- Blasius, R.; Duvoix, A.; Morceau, F.; Schnekenburger, M.; Delhalle, S.; Henry, E.; Diederich, M. Curcumin stability and its effect on glutathione S-transferase P1-1 mRNA expression in K562 cells. Annals of the New York Academy of Sciences 2004, 1030, 442–448.
- Ima, K.; Ravia, A.; Kumara, D.; Kuttanb, R.; Maliakela, B. An enhanced bioavailable formulation of curcumin using fenugreek-derived soluble dietary fibre. Journal of Functional Foods 2012, 4 (1), 348–357.
- Nishikawa, H.; Jinsyo, T.; Kitani, S. Anti-inflammatory and anti-oxidative effect of curcumin in connective tissue type mast cell. Journal of Functional Foods 2013, 5, 763–772.
- Sadiq Butt, M.; Sultan, M.T. Selected functional foods for potential in disease treatment and their regulatory issues. Journal of Functional Foods, 2013 16 (2), 397–415.
- Tlili, N.; Elfalleh, W.; Hannachi, H.; Yahia, Y.; Khaldi, A.; Ferchichi, A.; Nasri, N. Screening of natural antioxidants from selected medicinal plants. Journal of Functional Foods 2013, 16 (5), 1117–1126.