Abstract
Cyanidin-3-glucoside is an anthocyanin that is abundant in blueberry. Lauric acid was used as the acyl donor in the acylation of cyanidin-3-glucoside. Preparative high performance liquid chromatography was used to separate and purify the acylated cyanidin-3-glucoside, the acylated rate was 30.78%. Fourier transform infrared spectroscopy and liquid chromatography mass spectrometry were used to confirm the basic structure of cyanidin-3-glucoside. Lauric acid was combined with cyanidin-3-glucoside. Lauric acid reacted with the glucoside’s primary hydroxyl group and removed a molecule of water, thereby resulting in the obtained structure. The compositions of the unacylated cyanidin-3-glucoside and acylated cyanidin-3-glucoside were compared under different temperatures and under illumination. The effects of different concentrations of additive compounds on the stability of acylated cyanidin-3-glucoside were compared. Stability of acylated cyanidin-3-glucoside was obviously higher than that of the unacylated cyanidin-3-glucoside because of the higher stability of the ester group than the hydroxyl group. The primary hydroxyl esterification of glucoside improved cyanidin-3-glucoside.
INTRODUCTION
Anthocyanins are bioactive flavonoid compounds that demonstrate a high potential for use as natural colorants because of their attractive color and usually are incorporated into aqueous food systems.[Citation1,Citation2] Blueberry is a popular fruit because of its taste and high anthocyanin concentration. This fruit contains a higher amount of anthocyanins compared with other fruits. Anthocyanins have good antioxidant properties and may protect against many chronic diseases because of their anti-inflammation, liver protection, and antithrombotic, antitumor, and immunity-enhancing activities.[Citation3–Citation5] Blueberry cv. “Patriot” contains a high concentration of the anthocyanin Cyanidin-3-glucoside (C3G; 75%).[Citation6] This cultivar also contains other kinds of anthocyanins, such as pelargonidin, delphinidin, peonidin, petunidin, and malvidin, at low amounts. These anthocyanins differ in terms of hydroxyl number, methylation, glycosylation number, glycoside number, and connecting position.[Citation7,Citation8] Various anthocyanins present different colors in diverse environments.[Citation9,Citation10] Anthocyanins have positive and protective effects on human health. Compared with other colorants, anthocyanins have better antioxidant activity and may help prevent many chronic diseases. However, similar to unstable colorants, anthocyanin shows limited stability and solubility in oils.[Citation11,Citation12]
Various unacylated anthocyanins can be pH indicators. At low pH, unacylated anthocyanins show a deep red color. At neutral pH, nattier blue color is presented. Thus, the susceptibility of anthocyanins to pH, temperature, lighting, and metal ions significantly limits the application of these compounds.[Citation13,Citation14] C3G can be an obvious research target and example for investigating such color changes.
Acylated anthocyanins are superior to unacylated anthocyanins in two aspects, namely, good stability and lipotropic resistance. A previous study reported the occurrence of many kinds of naturally acylated anthocyanins. Willlams extracted 3-malonyl-D-glucopyranose-7-mustard acid-cyanidin and studied the ramifications of removing its malonyl group.[Citation15] Shimizu also extracted an acylated pelargonidin compound.[Citation16] In acidic solution, four indicators were found in anthocyanins, namely, quinoid alkali, huang yang salt-positive ion, pseudo-base, and chalcone. At pH levels lower than 2, anthocyanins mostly present a red color because of the huang yang salt positive ion. Increasing pH resulted in blue colored anthocyanins because of the presence of the quinoid alkali compound. Huang yang salt positive ion formed a colorless pseudo-base. Such pseudo-base maintained the balance to colorless chalcone at slow speed.[Citation17]
Acylation can effectively inhibit the transformation of these four chemical structures.[Citation18] Lauric acid, which is used in acylation experiments, can largely affect the stability of anthocyanins. Certain scholars have described anthocyanins’ “sandwich” structure,[Citation19] which indicated that there were acyl groups around the anthocyanin, and they were added in the glycosyl, and the carbohydrate could be folded and rotated. The accumulating form can resist the water’s nucleophilic attack and other degradation, so it could improve the stabilities of anthocyanin. Clydesdale[Citation20] reported that cinnamic acid residue is accumulated parallel to nuclear anthocyanins to protect the chromophore from the nucleophilic attack of water molecules. Other scholars used 2D NMR technology to analyze the radish pigment’s 2-phenyl-benzopyrene, which is spatially close to the acyl group’s hydrogen atom. These findings prove that anthocyanins are folded into a “sandwich” structure.[Citation21] The anthocyanin tertiary structure’s discoloration is due to C2 combining with water and yielding a colorless acetal compound. When other molecules are used to cover C2 or to avoid hydration, stability may increase. In the presence of an acyl group, the anthocyanin fails to turn into a colorless chalcone or blue quinine ketone and retains its red color. Dangles,[Citation22] Saito,[Citation23] and Yoshida[Citation24] proved that acylated anthocyanins had better stability and deeper color than unacylated anthocyanins, and this phenomenon is due to the “sandwich” structure accumulation. The second positive aspect of anthocyanins is lipotropism. Flavonoid compounds such as anthocyanins are directly extracted from natural plants and generally exist in free states and as glucoside. The molecular structure of anthocyanins contains many hydroxyls, including phenolic and alcoholic hydroxyl groups. The benzene ring in the flavonoid compounds has hydrophobic grouping, and hydroxyl belongs to the hydrophilic group. Thus, anthocyanins are both hydrophilic and hydrophobic. Given the many hydroxyls in flavonoid compounds, many anthocyanins have low lipotropism.[Citation25–Citation27]
Currently, improving lipotropism is the main research objective in the molecular modification of flavonoid compounds. The different kinds, amounts, and connecting locations of glucoside are available. Thus, anthocyanins as a flavonoid compound nearly exist in a glycosidic state, connecting with O-glycosidic and C-glycosidic bends. C3G is one of these compounds. Some kinds of flavonoid compounds, such as aglycone and glucoside, have different bioavailabilities. Németh found that aglycone has good bioavailability.[Citation28] Acylated modification of glucoside can retain the main activity and improve lipotropism, thereby promoting fat and lipid metabolism. A previous study showed that acylated anthocyanins are more stable than unacylated anthocyanins.[Citation25] To improve anthocyanin stability and solubility in oils and to broaden its future application in the food industry, using a chemical method to acylate anthocyanins is an optimal choice. Given that C3G is the main anthocyanin in blueberry cv. “Patriot,” we used this compound as the reaction subject. Maused lauric and tetradecanoic acids to acylate bamboo leaf flavonoid and found that lauric acid showed better effects on glucoside in an acylation reaction.[Citation29] Thus, we selected lauric acid as the acylating agent. Lauric acid is a long-chain fatty acid that can effectively connect primary hydroxyl groups in glucoside. In the reaction process, the main structure of lauric acid cannot be destroyed. We aimed to investigate the interaction between lauric acid and anthocyanin, the formation properties of acylated C3G, and the effect of such properties on the stability of anthocyanins.
MATERIALS AND METHODS
Materials and Reagents
C3G was obtained from blueberry according to the manufacturer’s instructions (Sigma, USA). This extract consisted of 80% w/w C3G. The following reagents were also used: lauric acid (Sinopharm Chemical Reagent, China), 1-(3-Dimethylaminopropyl)-3-ethylcarbodiimi-de, N-hydroxybenzotriazole (HOBt; Aladdin, China), dimethylformamide (DMF; Aladdin, China), trifluoroacetic acid (TFA; Aladdin, China), methyl alcohol, acetonitrile (ACN; Fisher, USA), citric acid, sodium citrate, vitamin C, sucrose, and glucose. Solvents and general chemicals were of analytical grade (Beijing Chemical Reagent Co., China).
Chemical Acylation Procedure
C3G (20 mg) was combined with two molar equivalents of acyl donor substrates using DMF as solvents. The samples were placed in a round bottom flask using argon as protective gas. The reaction was continuously stirred from 0 h to 48 h at 4°C. Samples (10 μL) were obtained every 12 h, diluted with methanol, and analyzed by high performance liquid chromatography-mass spectrometry (HPLC-MS) to monitor the extent of reaction. Acyl donor substrates included one molar equivalent of lauric acid compared with C3G, one and a half molar equivalents of 1-(3-dimethylaminopropy)-3-ethycarbodiimide, and one molar equivalent of HOBt. The hybrid reaction was performed at 20°C and proceeded for 30 min. When the reaction was completed, methanol was used to stop the reaction.
Preparative High Performance Liquid Chromatography (PHPLC) Separation and Purification Procedure
The acylation product was purified and prepared using PHPLC. The preparative liquid chromatography equipment used was the Waters preparation liquid chromatography system, and the detector was 2998. X-Bridge C18 preparative column (5 μm; 19 mm × 150 mm) was used with gradient elution to generate the preparative liquid. The solvents included (A) ACN and (B) H2O + 0.1% TFA. The mobile phase was H2O (0.1% TFA)/ACN (40 to 95% in 10 min). The flow rate was 15 mL/min. The sample injection volume was 1000 μL each time, UV detection wavelength was 280 nm, and column temperature was 20°C. The sample of the peak should be manually selected at a retention time of 6.24 min. After repeated preparations, the emerging elute was transformed by rotary evaporation into a dry powder. After weighing, Fourier transform infrared (FTIR) spectroscopy and liquid chromatography mass spectrometry (LC-MS) were performed for analysis.
FTIR Analytical Procedure
NEXUS 670 FTIR (Thermo Fisher Nicolet) and deuterated triglycerine sulfate detector were used; spectral region was 4000 to 400 cm−1. The resolution ratio was 2 cm−1. The scanning signal cumulative frequency was 32×. Upon scanning, interference of H2O and CO2 was excluded. C3G and acylated C3G was obtained separately to combine with KBr by grinding evenly. FTIR was used for analysis.
LC-MS Analytical Procedure
LC-MS was performed, and ICQ Deca ion trap mass spectrometer was fitted with an electrospray ionization (ESI) interface (Thermo Quest, Finnigan, San Jose. CA, USA) and coupled to a SurveyTM or HPLC and PDA detector. The HPLC column used was X-Bridge C18 (5 μm, 4.6 mm × 150 mm) analytical column maintained at 20°C. Solvents were (A) ACN and (B) H2O + 0.1% TFA. The mobile phase was H2O (0.1% TFA)/ACN (40 to 95% in 10 min). The flow rate was 1 mL/min, and sample injection volume was 20 μL. UV-vis detection absorbance was at 200 nm to 600 nm. MS data were acquired in the positive mode using a data-dependent LC-MS3 method with dynamic exclusion enabled and a repeat count of two. The ESI voltage, capillary temperature, sheath gas pressure, and auxiliary gas were set at 27 V, 300°C, and 45 and 10 psi, respectively.
Stability Analysis of Acylated C3G
Citric acid–sodium citrate solution (pH 3.0) was used to dilute unacylated and acylated C3G 50 times before stability analysis. Three parts of 30 mL of diluted unacylated C3G were separately obtained and placed into 80, 100, and 120°C water bath at regular intervals (2, 4, 6, 8, and 10 h) to determine concentration changes. The same method was used for acylated C3G. Water bath kettle was used to process heating. The two targets were compared. Up to 50 mL of diluted unacylated and acylated C3G compounds were separately placed in white reagent bottles and stored under natural lighting. The content change was observed every 3 d. Finally, the two targets were compared. Three parts of 30 mL of diluted acylated C3G solution were combined with different concentrations of (5R)-[(1S)-1,2-Dihydroxyethyl]-3,4-dihydroxyfuran-2(5H)-one (vitamin C), sucrose, and glucose. The control group was combined with 30 mL distilled water. All the samples were left standing for 2 h, and the content change was then detected. Dual-wavelength pH-differential method was used to figure out the retention rate, using UV detector to detect. Take 1 mL diluted sample, add 9 mL buffer solution which pH is 1.0 and 4.5 respectively, and then detect the absorbance in 280 and 540 nm after a water bath for 40 min. The following formula is used:
Statistical Analysis
Experiments were performed at repeating three times, and the results were expressed as means standard deviation. Statistical analysis was performed using SPSS 16.0. One-way analysis of variance was used to assess the differences in biochemical indices. Differences between groups were considered significant at P<0.05.
RESULTS AND DISCUSSION
PHPLC Preparation Situation and Acylated Degree Determination
Peak 1 () with a preferred retention time of 6.44 showed acylated C3G. Considering the nucleoplasmic relationship is 631, the sample was manually selected from this range. Using the integration, peak 1 can occupy approximately 48.02% of the entire area. Using ACN and water (TFA) prepared by rotary evaporation, we can obtain the solid-powder product, and the detected weight of the powder was 8.65 mg. Using the following formula, we can obtain the acylated rate of 30.78%.
FIGURE 1 PHPLC chromatogram of products with lauric acids as acyl donor. Note: The peak1 was target acylated product, the peak 2, 3 and others were unknown and unrelated by-products.
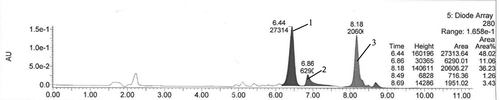
A is acylated rate, M2 is detected weight, M1 is theoretically acylated product weight, M0 is material weight, C0 is material MW, C1 is acylated product MW.
FTIR Analysis Comparison with Unacylated and Acylated C3G
C3G FTIR spectra had strong absorption peak at 3423 cm−1 ( line.a). The wide and strong absorption peak corresponded to many hydroxide radical stretching vibration peaks of aromatic ring and glycosyl in the structure of C3G. Considering that these hydroxide radicals formed a hydrogen bond association, the absorption peak’s wave number shifted to the low frequencies, and the waveform widened. Correspondingly, the absorption peak at 2924 cm−1 in the spectrogram was caused by methylene’s stretching vibration in the glycosyl. The absorption peaks at 1638 and 1444 cm−1 corresponded to the aromatic and heterocyclic rings’ skeletal vibration in the chromene of C3G. A series of absorption peaks between 1329 to 1074 cm−1 corresponded to the stretching vibration of C–O in glycosyl.
FIGURE 2 FTIR spectra of C3G and acylated C3G. Note: The line of a was the FTIR spectrum of C3G which was unacylated, the line of b was the FTIR spectrum of acylated C3G.
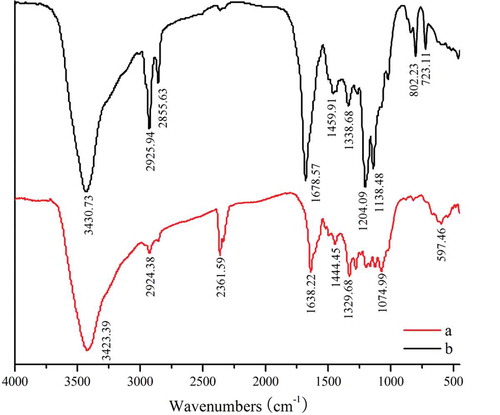
FIGURE 3 Total ion chromatograms of acylated C3G. Note: The liguid chromatogram was the total ion chromatogram of acylated C3G, the peak is what we wanted, called “acylated C3G” which retention time is 6.43.M.
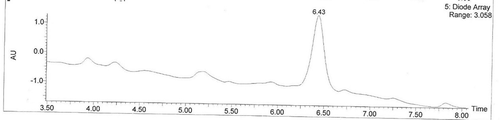
The FTIR spectrogram of acylated C3G is shown in line b. The spectrograms of the unacylated and acylated C3G were compared, the absorption peaks were at 2925 and 2855 cm−1, respectively, thereby revealing an obviously high absorption. The absorption peak at 2361cm−1 showed movement in the left direction. In general, saturated hydrocarbon, C–H, stretching vibrations were under and close to the absorption of 3000 cm−1. Thus, the long-chain saturated hydrocarbon’s addition can move the peak of 2361 cm−1 in the left direction. Therefore, we can preliminary conclude that lauric acid’s long aliphatic chain was already present in the structure of the anthocyanin. We failed to find the absorption peak of 1710 to 1715 cm−1, but the range surrounding these values had obvious absorption (). The phenolic hydroxyl group was not initially acylated. Under the lack of change of the skeleton pattern of normal chromene, the absorption peak at 1204 cm−1 had an obviously high intensity. The high absorption peak at 1204 cm−1 corresponded to the stretching vibration of C–O–C perssad, which belonged to the saturated fat spectra band. Generally, the ester groups’ existence was based on the stretching vibration of C–O–C, which can be the primary characteristic of absorption. Therefore, C3G was acylated, and lauric acid was added to the glucoside primary hydroxyl group.
LC-MS Analysis of Acylated C3G Structure
LC-MS analysis was performed to confirm the identity of the product. By HPLC analysis () at the retention time of 6.43, we showed that the product had a strong characteristic absorption. The structure analysis was performed by ESI. With the full scan process () at the positive ion mode, the acylated product can produce MS signals. In the environment of the solution, C3G usually exists in the form of O+ with a positive charge. We can further authenticate the structure of the synthetic product by tandem mass spectrometry.
FIGURE 4 Mass spectrogram of acylated C3G. Note: This was the mass spectrogram of acylated C3G, the m/z was 631, m/z + 1 = 632, m/z + 2 = 633.
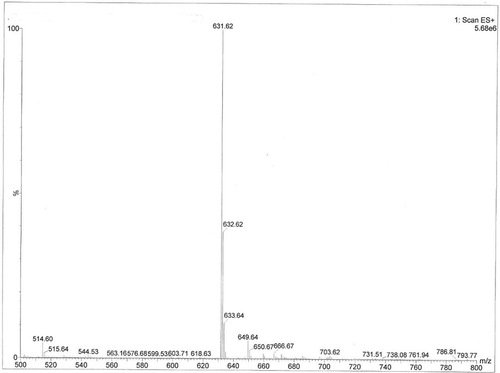
The synthetic product showed a single acyl esterification, as illustrated in . The molecular mass of C3G is 449, the molecular mass of lauric acid is 200, and both compounds were involved in the esterification reaction, which required the removal of a molecule of water. After the reaction, a product with a molecular mass of 631 was obtained. From the peak, m/z = 631 consist with the theoretically acylated C3G [M-H+], whereas m/z + 1 = 632, m/z + 2 = 633, the m/z of 632 and 633 are both the target products combined with one or two positive charges. Therefore, the acylated C3G is a single acyl compound. The primary hydroxyl group had a higher flexibility than the other position’s hydroxide radical in the structure of C3G. Adding the data from the FTIR analysis, we can obtain the reaction formula. The synthetic route and our target product are shown in . The synthetic principle states that the formation of mixed ester can enhance the combination of lauric acid with the primary hydroxyl group in the C3G, thereby demonstrating good selectivity.
Comparison of Stability between C3G and Acylated C3G
Effect comparison of stability at different temperatures
With increasing temperature and extension of heating time, the retention rates of acylated and unacylated C3G decreased ( to ). After heating from 0 h to 2 h at temperatures of 80, 100, and 120°C, retention rate of acyl and unacylated C3G showed no obvious differences. However, when the heating time was longer than 4 h, the decrease in retention rate of unacylated C3G was obviously higher than that of acylated C3G. Thus, acylated C3G has higher heat resistance than the unacylated C3G. Retention rate of acylated C3G after heating for 10 h at 80, 100, and 120°C are 83.24, 74.17, and 62.17%, respectively, and these rates were obviously higher than those of unacylated C3G. The acylation ability of C3G improved significantly (p < 0.05). C3G has 4 hydroxyl groups in the cyclo-chromene and four hydroxyl groups in the 6’glucoside. Thus, the hydroxyl groups make C3G unstable. Acylation of the ester can improve C3G’s stability. The acylated C3G combined with lauric acid to transform into a certain lipid. The ester group was more stable than hydroxide radicals, and a long-chain aliphatic hydrocarbon can make C3G more stable. Therefore, the acylated product from which the acyl group is added in the glucoside’s primary hydroxyl group can reduce the hydroxide radical’s activities.
Effect Comparison of Stability on Different Lighting Times
With prolonged lighting time, the stability of acylated and unacylated C3G decreased. The stability of acylated C3G was significantly higher than that of unacylated C3G (). When lighting was extended for 6 d, the retention rate of acylated C3G remained higher than 80%, whereas, that of unacylated C3G was 64.37%. The light stability and tolerance of acylated C3G were significantly higher (p < 0.05) than those of unacylated C3G.
Effect of Additive on Stability of Acylated C3G
Effect of vitamin C on stability
With the addition of Vitamin C, the retention rate increased (). Compared with the control group, different concentrations of Vitamin C had increased the retention rate of acylated C3G, and the retention rate of acylated C3G was approximately 90% on average after 10 d. By ANOVA, we determined that the different concentrations of vitamin C were not significantly different (p > 0.05) in terms of improving the stability of acylated C3G. However, vitamin C treatment significantly improved the retention rate of acylated C3G compared with no-vitamin C treatment (p < 0.05). Vitamin C addition can improve acylated C3G’s stability.
Effect of sucrose on stability
Sucrose increased the retention rate of acylated C3G compared with the control (). When the added amount reached 30%, the retention rate of acylated C3G remained above 85% after 10 h. ANOVA results showed that different sucrose concentrations failed to significantly differ (p > 0.05) in terms of improving the stability of acylated C3G. However, when sucrose was added at up to 30%, acylated C3G’s stability was significantly (p < 0.05) higher than that of acylated C3G with no sucrose addition. Adding an appropriate amount of sucrose improves the stability of acylated C3G.
Effect of glucose on stability
Compared with the control group, glucose-treated acylated C3G showed increased retention rate. When glucose was added up to 30%, the retention rate of acylated C3G remained above 80% after 10 h (). ANOVA results showed that varying concentrations of glucose failed to significantly differ (p > 0.05) in terms of effects on the stability of acylated C3G. However, when the glucose concentration was higher than 10%, the stability of acylated C3G showed a significant improvement (p < 0.05) compared with that of glucose-untreated acylated C3G. Glucose, similar to sucrose, can improve the stability of acylated C3G.
CONCLUSION
In the structure of C3G, the primary hydroxyl group is highly flexible and selective. Lauric acid and C3G were reacted in a mixed ester environment with alkaline DMF as a solvent. Preparation was performed by PHPLC, after which the solution was rotated to dry and weighed. The obtained acylation rate was 30.78%. After FTIR and LC-MS analyses, the ultimate product is a single ester compound. The acylated C3G had higher stability than unacylated C3G at different temperatures, lighting, times, and in the presence of various food additives, such as vitamin C, sucrose, and glucose. In the acylation reaction process, the color of acylated C3G was deeper than that of unacylated C3G. This reaction can lead to pigment color change. We can research this aspect of C3G in future experiments. Acylated anthocyanins can expand the application of such compounds in the food industry.
FUNDING
This research was supported by “the Fundamental Research Funds for the Central Universities (No. BLX2013020),” “the National Natural Science Funds of China (No. 31271981),” “the National Natural Science Base Fund (No. J1103516),” and “the National Key Technology Research and Development Program of China for the 12th five-year plan (Grant No. 2012BAD31B05).”
Additional information
Funding
REFERENCES
- Mazza, G.; Brouillard, R. Recent developments in the Stabilization of Anthocyanins in Food Products. Food Chemistry 1987, 25, 207–225.
- Mazza, G.; Miniati, E. Anthocyanins in Fruits, Vegetables, and Grains; CRC Press: London, 1993; 362 p.
- Gabrielska, J.; Oszmianski, J.; Komorowska, M.; Langner, M. Anthocyanin Extracts with Antioxidant and Radical Scavenging Effect. Journal of Biosciences 1999, 54, 319–324.
- Ghiselli, A.; Nardini, M.; Baldi, A.; Scaccini, C. Antioxidant Activity of Different Phenolic Fractions Separated from An Italian Red Wine. Journal of Agricultural and Food Chemistry 1998, 46, 361–367.
- Kopjar, M.; Orsolic, M.; Pilizota, V. Anthocyanins, Phenols, and Antioxidant Activity of Sour Cherry Puree Extracts and Their Stability During Storage. International Journal of Food Properties 2014, 17, 1393–1405.
- Jian, C. Research on Antagonistic Chemical Liver Injury and Its Mechanisms in Blueberry Anthocyanins; Beijing Forestry University, 2013.
- Elisia, I.; Hu, C.; Popovich, D.G.; Kitts, D.D. Antioxidant Assessment of a Anthocyanin-Enriched Blackberry Extract. Food Chemistry 2007, 101, 1052–1058.
- Chen, J.; Tao, X.Y.; Sun, A.D.; Wang, Y.; Liao, X.J.; Li, L.N.; Zhang, S. Influence of Pulsed Electric Field and Thermal Treatments on the Quality of Blueberry Juice. International Journal of Food Properties 2014, 17, 1419–1427.
- Legua, P.; Melgarejo, P.; Martinez, J.J.; Martinez, R.; Hernandez, F. Evaluation of Spanish Pomegranate Juices: Organic Acids, Sugars, and Anthocyanins. International Journal of Food Properties 2012, 15, 481–494.
- Jakobek, L.; Seruga, M. Influence of Anthocyanins, Flavonols, and Phenolic Acids on the Antiradical Activity of Berries and Small Fruits. International Journal of Food Properties 2012, 15, 122–133.
- Bakowska-Barczak, A.M.; Marianchuk, M.; Kolodziejczyk, P. Survey of bioactive Components in Western Canadian Berries. Canadian Journal of Physiology and Pharmacology 2007, 85, 1139–1152.
- Banerjee, A.; De, B. Comparative Study of Antioxidant Activity of the Food Flowers of West Bengal, India. International Journal of Food Properties 2013, 16, 193–204.
- Rice-Evans, C.A.; Miller, N.J.; Paganga, G. Structure-Antioxidant Activity Relationships of Flavonoids and Phenolic Acids. Free Radical Biology and Medicine 1996, 20, 933–956.
- Giusti, M.M.; Wrolstad, R.E. Acylated Anthocyanins from Edible Sources and Their Applications in Food Systems. Biochemical Engineering Journal 2003, 14, 217–225.
- Williams, C.; Greenham, J.; Harborne, J.; Kong, J.M.; Chia, L.S.; Goh, N.K. Acylated Anthocyanins and Flavonols from Purple Flowers of Dendrobium cv.‘Pompadour’. Biochemical Systematics and Ecology 2002, 30, 667–675.
- Shimizu, T.; Ichi, T.; Iwabuchi, H.; Kato, Y.; Goda, Y., Structure of Diacylated Anthocyanins from Red Radish (Raphanus sativus L.). Japanese Journal of Food Chemistry 1996, 3, 5–9.
- Wrolstad, R.E.; Giusti, M.M.; Rodriguez-Saona, L.E.; Durst, R.W. Anthocyanins from Radishes and Red-Fleshed Potatoes. Chemistry and Physiology of Selected Food Colorants 2001, 5, 66–89.
- Dong, L.; Jian-ya, Q.; Xiao-hui, Z. The Research Development of Acylated Anthocyanins. Guangzhou Food Science and Technology 2005, 19, 101–104.
- Bkowska-Barczak, A. Acylated Anthocyanins As Stable, Natural Food Colorants—A Review. Polish Journal of Food and Nutrition Sciences 2005, 14, 107–116.
- Clydesdale, F.; Main, J.; Francis, F. Roselle (Hibiscus sabdariffa L.) anthocyanins As Colorants for Beverages and Gelatin Desserts. Journal of Food Protection (USA) 1979, 42,165–169.
- Giusti, M.M.; Ghanadan, H.; Wrolstad, R.E. Elucidation of the Structure and Conformation of Red Radish (Raphanus Sativus) Anthocyanins Using One-and Two-Dimensional Nuclear Magnetic Resonance Techniques. Journal of Agricultural and Food Chemistry 1998, 46, 4858–4863.
- Dangles, O.; Saito, N.; Brouillard, R. Anthocyanin Intramolecular Copigment Effect. Phytochemistry 1993, 34, 119–124.
- Saito, N.; Tatsuzawa, F.; Yoda, K.; Yokoi, M.; Kasahara, K.; Iida, S. Acylated Cyanidin Glycosides in the Violet-Blue Flowers of Ipomoea Purpurea. Phytochemistry 1995, 40, 1283–1289.
- Yoshida, K.; Kondo, T.; Goto, T. Unusually Stable Monoacylated Anthocyanin from Purple Yam Dioscorea Alata. Tetrahedron Letters 1991, 32, 5579–5580.
- Ishihara, K.; Nakajima, N. Structural Aspects of Acylated Plant Pigments: Stabilization of Flavonoid Glucosides and Interpretation of Their Functions. Journal of Molecular Catalysis B: Enzymatic 2003, 23, 411–417.
- Moon, Y.H.; Lee, J.H.; Jhon, D.Y.; Jun, W.J.; Kang, S.S.; Sim, J. Synthesis and Characterization of Novel Quercetin-α-d-Glucopyranosides Using Glucansucrase from Leuconostoc Mesenteroides. Enzyme and Microbial Technology 2007, 40, 1124–1129.
- Tommasini, S.; Raneri, D.; Ficarra, R.; Calabrò, M.L.; Stancanelli, R.; Ficarra, P. Improvement in solubility and Dissolution Rate of Flavonoids by Complexation with β-Cyclodextrin. Journal of Pharmaceutical and Biomedical Analysis 2004, 35, 379–387.
- Németh, K.; Plumb, G.W.; Berrin, J.G.; Juge, N.; Jacob, R.; Naim, H. Y. Deglycosylation by Small Intestinal Epithelial Cell β-Glucosidases Is a Critical Step in the Absorption and Metabolism of Dietary Flavonoid Glycosides in Humans. European Journal of Nutrition 2003, 42, 29–42.
- Ma, X.; Yan, R.; Yu, S.; Lu, Y.; Li, Z.; Lu, H. Enzymatic Acylation of Isoorientin and Isovitexin from Bamboo-Leaf Extracts with Fatty Acids and Antiradical Activity of the Acylated Derivatives. Journal of Agricultural Food Chemistry 2012, 60, 10844–10849.