Abstract
The objective of this study was to investigate the effects of the acid- and alkali-aided processes on bighead carp (Aristichthys nobilis) muscle protein as compared with the conventional washing method. Acid- and alkali-aided processes resulted in higher protein recoveries than conventional washing method. In textural profile analyses, the highest hardness and cohesiveness were observed in the gels prepared by conventional washing and alkali-aided processing (p < 0.05). No endothermic peak was found in the differential scanning calorimetry thermogram of protein isolates after acid- and alkali-aided processes. The hydrophobic interactions and disulfide bonds were the main chemical bonds to maintain the stability of the gel matrix, while the sulfhydryl group in bighead carp muscle was apt to be activated during alkali-aided processing. The α-helical content of actomyosin decreased remarkably after the acid- and alkali-aided processes (p < 0.05). Overall, there is a potential to apply the alkali-aided processing to extract proteins from bighead carp muscle for the production of surimi-based seafoods.
INTRODUCTION
Numerous efforts are made to utilize fish by-products and low value fish species, due to the increasing awareness of the gap between the decreasing marine resources and the increasing demand for fish products.[Citation1,Citation2] Bighead carp (Aristichthys nobilis), one of the main freshwater fish species in China, can improve water quality by suppressing excess propagation of zooplankton in the surrounding water.[Citation3] The global production of farmed bighead carp has reached close to 3 million metric tons in 2012, while it ranks the 5th among all cultured freshwater fish.[Citation4] However, bighead carp flesh is usually recognized as an underutilized material due to its muddy smell, tiny bones, and coarse texture. Only the head of bighead carp is considered as a highly sought-after culinary item, while its flesh is sold at a very low price, especially in China. Hence, it is considered as an effective utilization to convert bighead carp flesh into surimi and further manufacture surimi-based products, such as kamaboko, fish ball, and shellfish substitutes, to satisfy the demand of consumers and produce value-added products.
Conventional surimi process involves several washing steps with chilled water (5–10°C) until most of the water-soluble compounds are removed.[Citation2,Citation5] The washing procedure plays an important role in improving gel-forming ability of surimi by removing fat and undesirable materials, such as bone, pigment, blood. and fat, and concentrating myofibrillar proteins.[Citation5] However, the loss of water-soluble proteins during water washing reduces the total protein yield in surimi production.[Citation6] Besides the conventional washing (CW), the pH-shifting process is an alternative for recovering protein efficiently.[Citation7] It often involves several steps: (1) homogenization fish mince with water in the ratio of 1:5~9; (2) solubilization fish protein at extreme acid or alkaline condition; (3) recovery of fish protein at the isoelectric point (PI). Currently, the pH-shifting process was applied to some fish species, such as Atlantic croaker,[Citation8] tilapia,[Citation5,Citation9] and silver carp.[Citation1,Citation10]
Until now, no study has been reported documenting the utilization of pH-shifting process on the production of bighead carp muscle protein isolate. Thus, the objective of this study was to investigate the effects of the acid- and alkali-aided (AL) processes on bighead carp muscle proteins as compared with the CW for surimi manufacture.
MATERIAL AND METHODS
Materials
Three live bighead carps (Aristichthys nobilis; body length: 35.6 ± 1.5 cm; weight: 1.05 ± 0.2 kg) were purchased from a local supermarket (Wuhan, Hubei, China) and transferred to the laboratory within 30 min. On arrival, bighead carps were stunned and then immediately headed, eviscerated, and washed with 4°C water. Fish mince was prepared by passing the gutted fish through a grinder (YY90L4, Hong Kong Fuxiang machinery Co., Ltd., Hong Kong, China) with perforations of 2 mm diameter. Analytical grade chemicals were used. Preparation of all samples was performed on ice to maintain the temperature below 5°C.
Preparation of Conventional Washed Surimi
Ground fish muscle was mixed with cold (4°C) distilled water (1:5, w/w) and allowed to stand for 15 min after 3 min stirring with a plastic spatula. The slurry was strained with double-layer cheesecloth, while the excess water was manually squeezed out. The washing and straining process was repeated three times, with the last washing containing 0.5% NaCl in the distilled water to facilitate the dewatering step. Washed surimi was then mixed with cryoprotectants (4% sucrose, 4% sorbitol, and 0.3% sodium tripolyphosphate). After adjusted to 78% moisture content by adding the cold (4°C) distilled water, surimi was vacuum-packed and stored at –20°C prior to use. The moisture content of sample was determined according to the method of Ingadottir and Kristinsson[Citation9] and the final pH value of surimi was 7.0.
Preparation of Protein Isolates
Ground fish muscle was homogenized with cold (4°C) distilled water (1:6, w/w) in a homogenizer (FJ200-SH, Shanghai Specimen and Model Factory, Shanghai, China) for 10 min at 15,000 r.p.m. Our previous study demonstrated that bighead carp muscle proteins were solubilized maximally at pH 2.0 and 12.0 and its PI was at pH 5.5. Thus, pH 2.0 and 12.0 were selected for solubilizing bighead carp muscle proteins, while pH 5.5 was used to recover protein isolates. The homogenate was adjusted from about 7.0 to pH 3.0 using 2 M cold (4°C) HCl or to pH 12.0 using 2 M cold (4°C) NaOH to solubilize protein for 10 min. Subsequently, the homogenates were then centrifuged (Avanti J-E, Backman, California, USA) at 10,000 × g for 20 min at 4°C to separate the insoluble substance. After centrifugation, the upper layer (neutral lipid) and the bottom sediment (connective tissue, insoluble lipid membranes, and skin) in the centrifuge tube were discarded, while the middle layer (solubilized protein) was filtered through four-layer cheesecloth and then adjusted to pH 5.5 with 2 M cold (4°C) NaOH or 2 M cold (4°C) HCl. Acid- or alkali-treated protein isolates were collected by centrifugation at 10,000 × g for 20 min at 4°C. After mixing with the same cryoprotectants as mentioned above, the protein isolates were subsequently vacuum-packed and stored at –20°C. The moisture content of protein isolates was adjusted to 78% by adding cold (4°C) distilled water, while their pH values were adjusted to 7.0 with 2 M cold (4°C) NaOH.[Citation9]
Protein Recovery
Protein content of each of the fish pastes was determined by the Kjeldahl method,[Citation11] while the recoveries of proteins from different treatments were calculated according to the method of Kim et al.[Citation12] with a slight modification. Protein recovery (% yield) was expressed as the weight of recovered protein divided by the weight of minced fish muscle (at the same moisture content). After CW as well as acid- and AL processes, moisture contents of surimi and protein isolates were equally adjusted to 81% moisture (the initial moisture content of fish muscle).[Citation9] The protein recovery was calculated with the following equation:
Gel Preparation
After frozen samples were thawed at 4°C for 12 h in advance, fish paste was prepared by chopping washed muscle/protein isolate in a Mini Chopper (HR7625, Philips, Hong Kong, China). During homogenization, cold distilled water (4°C) was added into the paste for adjusting the moisture content to 78% and preventing the protein denaturation. After chopping for 1 min, 2% dry NaCl was added to the paste and blended for another 1 min. The paste was then manually stuffed into plastic casing (25 cm in length, 20 mm in diameter) and both ends of the casing were sealed tightly. The paste was cooked for 30 min at 90°C in a water bath (HH-4, Changzhou Guohua Electric Co., Ltd., Jintan, Jiangsu, China) and then cooled in iced water for 20 min. Gels were held at 4°C overnight prior to analysis.
Instrumental Texture Profile Analyses (TPA)
The TPA was performed using a TA.XTPlus Texture Analyzer (Texture Technologies Corp., Scarsdale, NY, USA) equipped with a cylindrical steel plunger (P/36R, diameter 35 mm). Gels were equilibrated to room temperature for 2 h followed by cutting into 20 mm long samples prior to the TPA. The samples were placed on the flat plate and vertically compressed twice by the plunger at a speed of 1 mm/s. The compression rate was 0.4 of the original height for keeping from cracking the gel. Hardness, springiness, cohesiveness, and chewiness were obtained from the TPA curve.[Citation13]
Differential Scanning Calorimetry (DSC)
Samples (18–20 mg) were weighed and sealed in the hermetical aluminum pans, with a contact between the sample and the pan bottom. DSC was performed using a differential scanning calorimeter (NETZSCH-Gerätebau GmbH, Selb, Germany). All samples were scanned at 10°C/min from 15 to 95°C in triplicates. Helium gas was used as the protective gas during the measurement.
Protein Solubility
Determination of protein solubility for paste and gel was carried out according to the method of Gómez-Guillén et al.[Citation14] with a slight modification. The sample (2 g) was homogenized in a homogenizer (FJ200-SH, Shanghai Specimen and Model Factory, Shanghai, China) for 2 min with 20 mL of 0.05 M NaCl (SA), 0.6 M NaCl (SB), 0.6 M NaCl + 1.5 M urea (SC), 0.6 M NaCl + 8 M urea (SD), respectively. The homogenates were centrifuged at 10,000 × g for 15 min. Protein content in supernatants was determined using the Bradford method.[Citation15] The difference in solubilized protein content between two adjacent reagents was used to determine the existence of ionic bond (difference in solubilized protein content between SB and SA), hydrogen bond (difference in solubilized protein content between SC and SB), and hydrophobic interaction (difference in solubilized protein content between SD and in SC).
Total Sulfhydryl Content
The total sulphydryl (SH) content of the protein paste and gel was determined by using the method of Ingadottir and Kristinsson[Citation9] with slight modifications. Paste or gel sample (1 g) was homogenized with 9 mL of 0.2 M Tris-HCl buffer (pH 7.1, containing 2% SDS, 10 mM EDTA and 8 M urea). The homogenate was heated at 100°C for 5 min in a water bath (HH-4, Changzhou Guohua Electric Co., Ltd., Jintan, Jiangsu, China) and then centrifuged at 10,000 × g for 15 min. After diluted 20 times, the supernatant (1 mL) was mixed with 0.01 mL of 10 mM Ellman’s reagent (5, 5′-dithiobis (2-nitrobenzoic acid), DNTB) and then incubated at 40°C for 25 min. The absorbance of the mixture was determined at 420 nm using a ultraviolet-visible (UV-VIS) spectrophotometer (UV-1750, SHIMADZU, Beijing, China). Total SH content was calculated using a molar extinction coefficient of 13,600 mol/cm.
Circular Dichroism (CD) Spectrum
The CD spectrum was measured using a CD spectrometer (J-1500, Jasco Co. Ltd., Tokyo, Japan). The actomyosin of surimi or protein isolate was prepared according to the method of Kim et al.[Citation12] The actomyosin solutions were diluted to 0.08 mg/mL using 20 mM Tris-HCl (pH 7.0, containing 0.6 M KCl) and then transferred to a 0.1 cm path length quartz cell. Molecular ellipticity was measured in the range from 200 to 250 nm with a scan rate of 100 nm/min. The spectra were corrected for the solvent signal and averaged over three scans. Molar ellipticity of actomyosin was determined using a mean residue weight of 110 g/mol. The content of α-helix structures was determined using the protein secondary structure analysis program (Yang’s method) provided by Jasco Co. Ltd. (Tokyo, Japan).
Statistical Analysis
All experiments were conducted in triplicates. Analysis of variance (ANOVA) was tested and performed using a SPSS package (SPSS 17.0 for Windows, SPSS Inc., Chicago, IL, USA). Differences among the mean values of various treatments were measured by Duncan’s multiple range test (p < 0.05).
RESULTS AND DISCUSSION
Protein Recovery
Acid-aided (AC) and AL processes exhibited remarkably higher protein recoveries (74.8 and 54.7%, respectively; p < 0.05) than CW (45.5%; ). Undeland et al.[Citation16] reported that 74 and 68% herring light muscle protein were recovered for AC and AL processes, respectively. AC and AL processes of channel catfish muscle showed higher protein recoveries than conventional surimi processing.[Citation6] The high recoveries achieved by AC and AL processes were probably due to the recovery of sarcoplasmic proteins during processing.[Citation16] In the CW, sarcoplasmic proteins are soluble and then can be removed during water washing. In addition, some myofibrillar proteins can also become soluble and then lose during water washing.[Citation5] In contrast, most sarcoplasmic proteins are retained during AC and AL processes, resulting in a higher yield as compared to CW. In the present study, the highest protein recovery was found in AC processing (p < 0.05). A higher yield was recovered using AC processing on Pacific whiting compared with CW,[Citation17] while AC processing led to about 14% higher recovery of croaker proteins than AL processing.[Citation18] As compared with AC processing, AL processing resulted in less protein denaturation and thus, less protein co-aggregation at PI and more soluble proteins were still left in the supernatant after the second centrifugation during AL processing.[Citation18] It led to less overall recovery for AL processing. Therefore, AC and AL processes produced much more fish protein from bighead carp muscle than CW, while AC processing could contribute to the highest protein yields.
Instrumental TPA
The TPA involves compressing the test sample at least twice and quantifying the mechanical parameters from the recorded TPA curves.[Citation19] In , there was no significant difference in springiness (p > 0.05). Thus, the result indicated that AC and AL processes could give gels the similar characterization of the extent structure broken down by the initial compression as compared with CW. In addition, the gel prepared by CW showed remarkably higher hardness than those prepared by pH shift processing (p < 0.05; ). It was attributed to the removal of sarcoplasmic proteins during water washing, resulting in concentrating myofibrillar proteins and enhancing gel hardness.[Citation5] In addition, the integrity of myofibrillar proteins is a key factor affecting gel properties of surimi,[Citation20] while acid or alkaline solubilization can lead to the substantial changes of myofibrillar proteins, which make a detrimental effect on gel properties of protein isolates.[Citation21] Moreover, AL processing led to the highest gel cohesiveness (p < 0.05), while AC processing resulted in the lowest TPA values except the springiness (). It implied that AC processing led to the weaker gel structure, comparing to CW and AL processing. The trout proteins recovered from AL processing also showed higher cohesiveness than those from AC processing.[Citation22] Generally, fish protein is easily denatured to form large aggregate under acid condition, while such aggregate cannot form a well ordered three dimensional structure and thus lead to a weaker gel.[Citation9] Moreover, the hydrolysis of the myosin heavy chains, usually observed in AC protein isolate, could partly reduce the gel forming ability of AC protein isolate.[Citation18] Overall, the protein isolate prepared by AC processing showed the weaker gel texture as compared with AL processing.
Table 1 Texture profile analysis of gels prepared by the conventional washing method, acid-, and alkali-aided processes
DSC
shows the DSC thermograms of CW surimi, AC, and AL protein isolates. While the temperature of endothermic peaks in CW surimi were found at 36.4 and 65.5°C, which were probably denaturation temperatures for myosin and actin of bighead carp muscles.[Citation23] In contrast, no endothermic peak was found in the thermograms of AC and AL protein isolates (). The similar pattern was also found in AC and AL protein isolates from rockfish muscles.[Citation24] It indicated that myosin and actin were denatured during AC and AL processes, since there was less thermal energy required for denaturing myosin and actin in protein isolates. Moreover, the protein aggregation also leads to the disappearance of endothermic peaks because the aggregation is an exothermic reaction, which compensates the energy needed for protein denaturation.[Citation24] Thus, there was no endothermic peak found in the DSC thermogram of AC or AL protein isolates.
Protein Solubility
In , the ionic bond, hydrogen bond, and hydrophobic interaction are presented as the protein solubility of fish pastes and gels in the different solutions. During grinding, myofibrillar proteins would solubilize and hydrate with the addition of salt, leading to the formation of several chemical bonds. However, sarcoplasmic proteins would not be soluble in the same system.[Citation25] The CW surimi showed a predominance of ionic and hydrogen bonds ( and ). The added NaCl in the homogenizing process of CW surimi preparation led to the solubility of myofibrillar proteins and then the proteins were hydrated with water, resulting in the formation of more ionic and hydrogen bonds as compared to protein isolates.[Citation25]
FIGURE 3 Chemical interactions in minced bighead carp muscles and their thermal gels (30 min at 90°C). A: Ionic bond; B: Hydrogen bond; C: Hydrophobic interaction; D: Total sulphydryl (SH) content. Means in columns with different letters were significantly different (p < 0.05). CW: conventional washing method, AC: acid-aided processing, AL: alkali-aided processing.
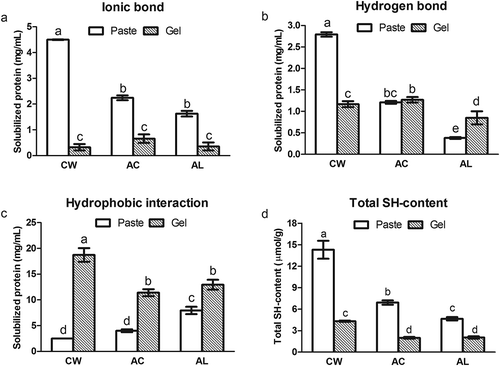
In contrast, protein isolates showed less ionic and hydrogen bonds but more hydrophobic interaction than CW surimi (, , and ). The formation of hydrophobic interaction may be correlated with the changes in the conformation of myosin tail.[Citation26] Thus, more hydrophobic residues in myosin tail are exposed after pH shift processing, while the exposed hydrophobic residues of denatured proteins lead to the formation of hydrophobic interactions.[Citation27] The exposure of the hydrophobic residues minimizes the hydration of proteins with water due to thermo-dynamically unfavorable decrease in entropy,[Citation28] resulting in the decrease of ionic and hydrogen bonds.
After heating, there was no significant increase in the hydrogen bond for gel samples compared with their pastes (p > 0.05), except AL protein isolate (). The ionic bond was significantly reduced (p < 0.05; ), while the hydrophobic interaction was sharply increased (p < 0.05; ) as compared to the pastes. Thus, the result demonstrated that hydrophobic interaction was the main bond for maintaining the stability of gel matrix and myofibrillar proteins had a stronger tendency to crosslink via hydrophobic interaction. During heating, proteins undergo a process of denaturation, which leads to the exposure of hydrophobic group in proteins, and aggregation, which results from hydrophobic interaction.[Citation14] Therefore, hydrophobic interaction plays a vital role in the formation of thermal gels.
Changes in total SH-content and disulfide (S-S) bonds are often associated with gelation of muscle proteins.[Citation9] In the present study, the total SH-content of fish pastes was obviously lower than that of gels (; p < 0.05). Compared with the pastes, a sharp decrease of the total SH-content in the gel samples usually indicates the formation of S-S bond during the thermal gelation.[Citation29] Thus, the results demonstrated that the S-S bond was another chemical interaction contributed to the formation of gel network. The gel prepared from alkali-treated protein isolate of rockfish showed the lowest content of SH-group, resulting from the highest formation of S-S bond and SH/S-S interchange reaction.[Citation24] Additionally, the total SH-content of protein isolates was considerably lower than that of CW surimi (p < 0.05), which was probably due to the fact that the oxidation of SH and SH/S-S interchange reaction could occur during AC and AL processes.[Citation24] Moreover, the AL processing resulted in the lower SH-content of protein isolate than AC processing (; p < 0.05), suggesting that the SH-group in bighead carp muscle protein became more reactive after AL processing. AL protein isolate from Pacific whiting also showed a lower total SH content than AC protein isolate.[Citation12] Usually, disulfide interchange reaction occurs at alkaline pH but is inhibited at acidic pH.[Citation30] Fish muscle proteins often probably carried negative charges that could produce strong repulsive force, so they underwent extensive unfolding in the alkaline condition. In addition, the SH group was likely to converted into the monovalent ion (S−) at about pH 8.3, facilitating the formation of S-S bond or the appearance of SH/S-S interchange reaction.[Citation24] Overall, the disulfide bond also played an important role in gel formation, while the sulfhydryl group in bighead carp muscle was apt to be activated during the AL processing.
CD
The CD spectrum is a useful technique for investigating the changes in the secondary structure of protein in diluted solutions.[Citation31] In , the CD spectrum of the CW surimi exhibits two valleys at around 208 and 222 nm, indicating the existence for α-helix structure of myosin.[Citation32] However, the negative molar ellipticity values sharply decreased in the CD spectra of protein isolates (), implying that there was a loss of α-helix structure after AC or AL processing. In , the α-helix content of actomyosin from washed surimi was 45.1% but decreased to 27.1 and 21.4% for AC and AL protein isolates, respectively (p < 0.05). Myosin is composed of two globular head regions and a rod-like tail portion.[Citation33] The rod-like tail portion is almost 100% helical, while the globular head region has less than 50% α-helix. Thus, the rod-like tail portion is considered to represent the great major of the secondary structure of myosin.[Citation21] As the pH of fish muscle solution moved to acidic or alkaline range, the increase of electrostatic repulsion led to the unfolding of myosin. The unfolded myosin could partly refold as the pH returned to neutral.[Citation21] In the present study, the results for the CD spectra of protein isolates demonstrated that the rod-like tail portion of myosin could not completely refold after the pH returned to neutral, eventually resulting in the reduction of α-helix content in the CD spectra (). In addition, the α-helix structure is mainly stabilized by hydrogen bond between carbonyl oxygen (-CO) and amino hydrogen (NH-) of a poly-peptide chain.[Citation34,Citation35] Besides, electrostatic interaction among amino acids is also contributable to the stability of secondary structures.[Citation36] There were decreases in hydrogen and ionic bonds of protein isolates after AC or AL processing (). Hence, the decrease of α-helix content also indicated that the breakage of hydrogen bond and electrostatic interaction in protein isolates during AC or AL processing. Overall, the result of CD spectra demonstrated that the rod-like tail portion of bighead carp myosin was sensitive to extreme acid and alkaline conditions.
CONCLUSIONS
AC and AL processes produced higher protein recoveries than CW, while AL processing resulted in higher gel cohesiveness as compared to CW. However, the results of DSC thermogram, protein solubility and CD spectrum demonstrated that chemical bonds and structure of bighead carp muscle proteins were modified after AC and AL processes. Further experimentation is needed to investigate the explicit structure changes of bighead carp muscle proteins induced by AC and AL processes. Overall, comparing with AC processing, there is a potential to apply AL processing to extract proteins from bighead carp muscle for the production of surimi-based seafood.
FUNDING
The authors would like to thank the Ministry of Scientific and Technology China (Grant No. 2012BAD28B06) and the Fundamental Research Funds for the Central Universities (Project 2013PY096) for the financial support.
Additional information
Funding
REFERENCES
- Azadian, M.; Moosavi-Nasab, M.; Abedi, E. Comparison of Functional Properties and SDS-PAGE Patterns Between Fish Protein Isolate and Surimi Produced from Silver Carp. European Food Research and Technology 2012, 235 (1), 83–90.
- Nolsøe, H.; Undeland, I. The Acid and Alkaline Solubilization Process for the Isolation of Muscle Proteins: State of the Art. Food and Bioprocess Technology 2008, 2 (1), 1–27.
- Liu, Z.Y.; et al. Fermentation of Bighead Carp (Aristichthys Nobilis) Surimi and the Characteristics of Fermented Bighead Carp Surimi Products. Journal of the Science of Food and Agriculture 2009, 89 (3), 511–516.
- FAO. Cultured Aquatic Species Information Programme Hypophthalmichthys nobilis (Richardson, 1845), 2014.
- Rawdkuen, S.; et al. Biochemical and Gelling Properties of Tilapia Surimi and Protein Recovered Using An Acid-Alkaline Process. Food Chemistry 2009, 112 (1), 112–119.
- Kristinsson, H.G.; et al. A Comparative Study Between Acid- and Alkali-Aided Processing and Surimi Processing for the Recovery of Proteins from Channel Catfish Muscle. Journal of Food Science 2005, 70 (4), C298–C306.
- Hultin, H.O.; Kelleher, S.D. Surimi Processing from Dark Muscle Fish. In Surimi and Surimi Seafood; Park, J.W.; Ed.; Marcel Dekker: New York, NY, 2000.
- Kristinsson, H.G.; Liang, Y. Effect of pH-Shift Processing and Surimi Processing on Atlantic Croaker (Micropogonias Undulates) Muscle Proteins. Journal of Food Science 2006, 71 (5), C304–C312.
- Ingadottir, B.; Kristinsson, H.G. Gelation of Protein Isolates Extracted from Tilapia Light Muscle by pH Shift Processing. Food Chemistry 2010, 118 (3), 789–798.
- Taskaya, L.; Chen, Y.-C.; Jaczynski, J. Color Improvement by Titanium Dioxide and Its Effect on Gelation and Texture of Proteins Recovered from Whole Fish Using Isoelectric Solubilization/Precipitation. LWT–Food Science and Technology 2010, 43 (3), 401–408.
- AOAC. Official Methods of Analysis, 17th Ed. ; Association of Official Analytical Chemists: Washington, DC, 2000.
- Kim, Y.S.; Park, J.W.; Choi, Y.J. New Approaches for the Effective Recovery of Fish Proteins and Their Physicochemical Characteristics. Fisheries Science 2003, 69 (6), 1231–1239.
- Pons, M. Instrumental Texture Profile Analysis with Particular Reference to Gelled Systems. Journal of Texture Studies 1996, 27 (6), 597–624.
- Gómez-Guillén, M.C.; Borderı́as, A.J.; Montero, P. Chemical Interactions of Nonmuscle Proteins in the Network of Sardine (Sardina Pilchardus) Muscle Gels. LWT–Food Science and Technology 1997, 30 (6), 602–608.
- Kruger, N.J. The Bradford Method for Protein Quantitation. Methods in Molecular Biology 1994, 32, 9–15.
- Undeland, I.; Kelleher, S.D.; Hultin, H.O. Recovery of Functional Proteins from Herring (Clupea Harengus) Light Muscle by An Acid Or Alkaline Solubilization Process. Journal of Agricultural and Food Chemistry 2002, 50 (25), 7371–7379.
- Choi, Y.J.; Park, J.W. Acid-Aided Protein Recovery from Enzyme-Rich Pacific Whiting. Journal of Food Science 2002, 67 (8), 2962–2967.
- Kristinsson, H.G.; Ingadottir, B. Recovery and Properties of Muscle Proteins Extracted from Tilapia (Oreochromis Niloticus) Light Muscle by pH Shift Processing. Journal of Food Science 2006, 71 (3), E132–E141.
- Szczesniak, A.S. Texture Is a Sensory Property. Food Quality and Preference 2002, 13 (4), 215–225.
- An, H.; Peters, M.Y.; Seymour, T.A. Roles of Endogenous Enzymes in Surimi Gelation. Trends in Food Science and Technology 1996, 7, 321–327.
- Kristinsson, H.G.; Hultin, H.O. Changes in Conformation and Subunit Assembly of Cod Myosin at Low and High pH and After Subsequent Refolding. Journal of Agricultural and Food Chemistry 2003, 51 (24), 7187–7196.
- Chen, Y.C.; Jaczynski, J. Protein Recovery from Rainbow Trout (Oncorhynchus Mykiss) Processing Byproducts Via Isoelectric Swolubilization/Precipitation and Its Gelation Properties As Affected by Functional Additives. Journal of Agricultural and Food Chemistry 2007, 55 (22), 9079–9088.
- Beas, V.E.; et al. Thermal Denaturation in Fish Muscle Proteins During Gelling: Effect of Spawning Condition. Journal of Food Science 1991, 56 (2), 281–284.
- Yongsawatdigul, J.; Park, J.W. Effects of Alkali and Acid Solubilization on Gelation Characteristics of Rockfish Muscle Proteins. Journal of Food Science 2004, 69 (7), C499–C505.
- Hwang, J.S.; Lai, K.M.; Hsu, K.C. Changes in Textural and Rheological Properties of Gels from Tilapia Muscle Proteins Induced by High Pressure and Setting. Food Chemistry 2007, 104 (2), 746–753.
- Yamamoto, K.; Miura, T.; Yasui, T. Gelation of Myosin Filament Under High Hydrostatic Pressure. Food Structure 1990, 9, 269–277.
- Chaijan, M.; et al. Physicochemical Properties, Gel-Forming Ability, and Myoglobin Content of Sardine (Sardinella Gibbosa) and Mackerel (Rastrelliger Kanagurta) Surimi Produced by Conventional Method and Alkaline Solubilisation Process. European Food Research and Technology 2005, 222(1–2), 58–63.
- Park, J.W. Surimi and Surimi Seafood, 2nd Ed; CRC Press: Boca Raton, FL, 2005.
- Lanier, T.C. Surimi Gelation Chemistry. In Surimi and Surimi Seafood; Park, J.W.; Ed.; Marcel Dekker: New York, NY, 2000; 237–265.
- Monahan, F.J.; German, J.B.; Kinsella, J.E. Effect of pH and Temperature on Protein Unfolding and Thiol/Disulfide Interchange Reactions During Heat-Induced Gelation of Whey Proteins. Journal of Agricultural and Food Chemistry 1995, 43, 46–52.
- Choi, S.M.; Ma, C.Y. Structural Characterization of Globulin from Common Buckwheat (Fagopyrum Esculentum Moench) Using Circular Dichroism and Raman Spectroscopy. Food Chemistry 2007, 102 (1), 150–160.
- Greenfield, N.J. Applications of Circular Dichroism in Protein and Peptide Analysis. Trends in Analytical Chemistry 1999, 18 (4), 236–244.
- Harrington, W.F.; Rodgers, M.E. Myosin. Annual Review of Biochemistry 1984, 53, 35–73.
- Damodaran, S. Aminoacids, Peptides, and Proteins. In Food Chemistry; Fennema, O.R.; Ed.; Marcel Dekker: New York, NY, 1996; 321–429.
- Liu, R.; et al. Effect of pH on the Gel Properties and Secondary Structure of Fish Myosin. Food Chemistry 2010, 121 (1), 196–202.
- Yuan, C.; et al. Structural Stability of Myosin Rod from Silver Carp As Affected by Season. Journal of Food Science 2011, 76 (5), C686–C693.