ABSTRACT
Grass carp skin pieces were homogenized in water and hydrolyzed by Alcalase®, collagenase, proteinase K, and/or trypsin at their optimum conditions. Samples were taken at various degrees of hydrolysis and were evaluated for antioxidant, antimicrobial, and angiotensin-converting enzyme inhibitory activities. Alcalase and collagenase completely hydrolyzed the skin with different rates, and released peptides with antioxidant and angiotensin-converting enzyme-inhibitory activity. These activities increased linearly with increasing degrees of hydrolysis. Subsequent incubation of the collagenase hydrolysates with trypsin slightly increased the antioxidant activity. Proteinase K, although only partially hydrolyzing the skin, also catalyzed the release of peptides with antioxidant and angiotensin-converting enzyme-inhibitory activities. These results show that skin by-products from grass carp can be a source of bioactive peptides produced by a one-step reaction. Such hydrolysates may be applied in food products to prolong shelf life and provide beneficial effects on blood pressure.
Introduction
The production of cultured fresh water fish has been increasing in China over the past decades, amounting to 26.47 million metric tons in 2013.[Citation1] Grass carp (Ctenopharyngodon idella) is one of the three most cultivated fresh water fish species worldwide, and the increased manufacturing of processed products (mainly fillet) from this species, in response to consumer demands, has resulted in a significant accumulation of by-products, such as viscera, heads, bone, and skin, which present an environmental burden and are of economic concern for the fish industry. Therefore, there is an increasing need to transform these by-products into value-added products. Grass carp skin is a rich source of high quality protein,[Citation2] which may potentially be used for human nutrition.
The major component of fish skin is collagen, which can be converted into gelatine by breaking down some crosslinkages during an extraction process.[Citation3] Several studies have investigated the extraction of gelatine from grass carp skin[Citation4–Citation6] and it’s emulsification and water binding capacity.[Citation2] In general, gelatines from fish skin have much lower gelling strength and lower melting and gelling temperature compared to gelatines from conventional sources, such as pig skin and bovine bone, which limits their application as gelling agents.[Citation3]
It is also possible to exploit protein-rich by-products from industrial food production by a more extensive enzymatic degradation into hydrolysates containing bioactive peptides with antioxidant, antimicrobial, anticancer, and/or blood-pressure lowering activity.[Citation7–Citation11] A number of studies have addressed the enzymatic release of bioactive peptides from marine fish skin samples, for example, peptides with antioxidant activity[Citation7,Citation8,Citation12,Citation13] and angiotensin-I converting enzyme inhibitory (ACE-I) activity.[Citation8,Citation14–Citation17] To our knowledge, no studies on the bioactivity of grass carp skin hydrolysates have been performed.
In several studies, fish skin protein hydrolysates made with trypsin or Alcalase® have shown better antioxidant activity than those made with other enzymes such as pepsin, α-chymotrypsin, or papain.[Citation7,Citation12,Citation18,Citation19] Alcalase has also been shown to efficiently catalyze the release of peptides with high ACE-I activity from Alaska pollack skin and other fish and collagen sources.[Citation14,Citation20–Citation23] In our previous study,[Citation22] proteinase K hydrolysates of bovine collagen also showed very high ACE-I activity despite of quite low degrees of hydrolysis (DH). Therefore, enzymes such as Alcalase, collagenase, trypsin, and proteinase K seem well-suited for catalyzing the release of bioactive peptides from fish skin.
In most of the studies mentioned previously, gelatine was first extracted from the skin and then hydrolyzed. However, it has been shown that some proteases such as collagenases and Alcalase have the ability to act on collagen directly.[Citation2,Citation22,Citation24] Direct use of the fish skin as substrate for the enzymatic release of bioactive peptides would be beneficial, since it would save energy, decrease the use of chemicals, and shorten the time needed for the extraction process.
The purpose of this study was (1) to assess whether four different enzymatic treatments could release bioactive peptides directly from grass carp skin, and (2) to determine the influence of the degree of hydrolysis on the bioactivity and possibly relate it to the peptide profiles of the hydrolysates. The bioactivities evaluated were the ACE-I activity, the antioxidant activity (by three different assays), and the antimicrobial activity.
Materials and methods
Materials
Frozen skin of grass carp fish (with scales removed) were provided by Huzhou Nanxun Xinya Produce Company, Zhejiang, China. The frozen skin samples were freeze-dried, and kept cool and dry. The gross composition of the freeze-dried skin was 65.8% protein, 34.4% lipid, and 3.67% water. The amino acid composition of the freeze-dried skin was analyzed following the Chinese national standard GB/T 5009.124-2003. Approximately 25 mg of freeze-dried skin was hydrolyzed in 6 M HCl at 110°C for 24 h, and the amino acids were quantified using a Hitachi L-8800 amino acid analyzer (Tokyo, Japan).
Proteinase K (from Engyodontium album, P6556), Alcalase (from Bacillus licheniformis P4860), trypsin (from bovine pancreas, T9201), ACE (EC 3.4.15.1, rabbit lung, 0.25 units), as well as ABTS (2,29-azinobis-(3-ethylbenzothiazoline-6-sulfonic acid), Ferrozine™ (3-(2-Pyridyl)-5,6-diphenyl-1,2,4-triazine-p,p´-disulfonic acid), L-α-phosphatidylcholine, and MTT (3-(4,5-Dimethyl-2-thiazolyl)-2,5-diphenyl-2H-tetrazolium bromide) were purchased from Sigma Chemical Co., St. Louis, MO, USA. Collagenase (type II from Clostridium histolyticum, 17101) was obtained from Invitrogen Corporation A/S, Carlsbad, CA, and o-aminobenzoylglycyl-p-nitro-L-phenylalanyl-proline (ABZ-Gly-Phe(NO2)-Pro) was obtained from Bachem (Bubendorf, Switzerland).
Production of hydrolysates and determination of degree of hydrolysis
The Grass carp skin was cut into small pieces (approximately 3 × 10 mm), washed with de-ionized water, and dried with filter paper before use. Seven portions (1.25 g each) of the freeze-dried skin pieces were rehydrated in water (at 1% w/v) for 2 h, and then homogenized with an Ultra-Turrax homogenizer for 5 min (at 25,000 rpm). The pH and temperature of each slurry was adjusted to the optimum conditions for the enzyme used (), and the enzyme was added at an enzyme-substrate ratio of 1% (w/w). One portion was hydrolyzed with Alcalase at 60°C and pH 8.0 to different DH (A1-A4), another portion was hydrolyzed with proteinase K at 37°C and pH 8.0 (P1-P3), and two portions with collagenase at 37°C and pH 7.5 (C1-C3). One of the C3 samples was subsequently subjected to hydrolysis with trypsin (after adjustment of the pH to 8.0) for approximately 1 and 3 h (C3T1 and C3T2). The remaining three portions were incubated without enzymes at 37°C for 10 h (B1) and at 60°C for 2 and 5 h, respectively (B2 and B3). The pH of the reaction was kept constant by addition of 0.1 N NaOH to the reaction medium using a pH-stat (718 STAT Titrino [Methrohm Ltd., Herisan, Switzerland]). Sample aliquots (3 mL) were taken during hydrolysis. The amount of alkali added during the hydrolysis was recorded and used to calculate the degree of hydrolysis. Collected samples were heated at 95°C for 15 min to inactivate the enzyme, cooled on ice, and centrifuged at 10,000 × g for 15 min (4°C). The upper layer (oil) was carefully removed with a pipette, and the clear supernatant was collected and frozen (–25°C) until analysis. Hydrolysates were analysed by liquid chromatography-mass spectrometry (LC-MS) and suitably diluted samples were assayed for bioactivity as described below.
Table 1. Enzymes and conditions used for hydrolysis of fish skin; and resulting degree of hydrolysis (DH).
Determination of peptide profiles and peptide masses
The hydrolysates were thawed and centrifuged (10,000 × g for 10 min) and samples were taken for LC-MS analysis. The blank samples B2 and B3 were still turbid and were not analyzed. LC-MS analysis was performed using an Agilent LC-MSD Trap as described by de Gobba et al.[Citation25] with a few modifications. From each sample, a volume of 25 μL was injected and separated using a gradient consisting of 0% B for 5 min followed by a linear increase to 40% B in 70 min. MS spectra were recorded from 50 to 2200 m/z with the target set to 1521 m/z.
Determination of ACE-I activity
The ACE-I activity of the grass carp skin hydrolysates was determined using the internally quenched fluorescent substrate o-amino-benzoylglycyl-p-nitro-L-phenylalanyl-proline (ABZ-Gly-Phe(NO2)-Pro) as described by De Gobba et al.[Citation25] The hydrolysates were diluted 10 times before measurement and 50 μL was subject to triplicate determinations. The inhibition of ACE was calculated using the slope of the control as 100% enzyme activity according to the following equation,
where Slopeinhib is the slope of the line for the sample and Slopecontrol is the slope for the control sample without hydrolysate.
Determination of antioxidant activity
The antioxidant activity of the hydrolysates was determined by three different assays testing radical scavenging and iron chelation properties as well as, inhibition of lipid oxidation in a model system.
ABTS radical scavenging assay
This assay, which is based on the decolorization due to reduction of the radical form of ABTS (2;29-azinobis-(3-ethylbenzothiazoline-6-sulfonic acid)); was performed as described in the article from De Gobba et al.[Citation25] The hydrolysate was diluted (40 or 50×) before analysis and 50 µL were used in the assay. The control sample consisted of ABTS•+ working solution (200 µL) and 50 µL of water. All samples were assayed in triplicate. The final readings were used for calculating the ABTS radical scavenging activity as outlined in the formula:
where Actrl is the absorbance of ABTS•+ in the control sample without hydrolysate, Asample is the absorbance of the ABTS•+ after addition of hydrolysate sample.
Iron chelation capacity
This assay, which is based on the complexation of iron with Ferrozine™ (3-(2-pyridyl)-5,6-diphenyl-1,2,4-triazine-p,p′-disulfonic acid) forming a purple complex, was performed as described by De Gobba et al.[Citation25] Briefly, in a transparent microtiter plate, 25 µL of hydrolysate (5× dilution) was mixed with 100 µL of a FeSO4 solution (75 µM in water) and incubated for 10 min at room temperature. Then, 100 µL of Ferrozine solution (500 µM in water) was added and the absorbance measured at 562 nm using a Multiskan EX plate reader (Labsystems Oy, Helsinki, Finland). The iron chelation ability was calculated as the inhibition (%) of the formation of the FeCitation2+-Ferrozine complex using the formula,
where Actrl is the absorbance of the Fe-Ferrozine complex in the control sample, and Asample is the absorbance of the Fe-Ferrozine complex in the presence of hydrolysate sample. All measurements were performed in triplicate.
Prevention of lipid oxidation in liposomes
The prevention of formation of secondary oxidation products in L-α-phosphatidyl choline liposomes initiated with 25 µM FeCl3 was detected fluorometrically as described by De Gobba et al.[Citation25] In each well, 10 µL of liposomes and 50 µL of diluted (5×) hydrolysate (or water) were added together with the glycine ascorbate buffer and the oxidizing reagent, and the fluorescence was monitored for 4 h at 37°C. The inhibition of lipid oxidation (in %) was calculated from the areas under the curve (AUC) using the formula:
where AUCsample is the AUC of the sample with hydrolysate and AUCctrl is the AUC of the control (with water added instead of hydrolysate).
Determination of antimicrobial activity
The antimicrobial activity was tested against two gram-negative and two gram-positive pathogenic bacterial species and a fungus using a modified radial diffusion assay as described by De Gobba et al.[Citation25] The species and strains used were Eschericia coli (1873 and JM109), Pseudomonas aeruginosa (PaO1), Staphylococcus aureus (8325), Listeria monocytogenes (EGDe), and Candida albicans (cbs 8758). Ten microliters of each hydrolysate were placed in the wells and the plates were incubated and stained as described. The anti-microbial activity is seen as a non-colored area around the well containing the hydrolysate.
Statistical analysis
Differences between samples (and enzymatic treatment) with respect to bioactivity were tested with the general linear model procedure, with blanks (antioxidant activities) or without blanks included (ACE-I), and significant differences between means were tested with Duncan’s Multiple range test using SAS 9.3 for windows (SAS Institute Inc.). Correlations were found using SigmaPlot for windows version 11.0 (©2008 Systat Software, Inc.).
Results and discussion
Characterization of hydrolysates
An overview of the fish skin hydrolysates and the DH obtained with each enzymatic treatment is given in . The blank samples incubated at 37°C (B1) and 60°C (B2 and B3), respectively, without added enzyme reached only DH values around 1%, which is lower than the DH values obtained in the presence of enzymes. The limited hydrolysis obtained in the blank samples might be due to the action of endogenous enzymes in the skin samples as reported for Pacific hake,[Citation19] or to a thermally induced helix-to-coil transition of fish collagen which could perhaps lead to release of some gelatine molecules. The thermal transition of collagen is normally achieved by heating to above 45°C,[Citation3,Citation26] but could probably occur over time at 37°C in fish collagen, which has a more labile crosslinking[Citation3] and thus might have a lower transition temperature.
As expected, the increase of DH of the hydrolysates over hydrolysis time varied with the enzyme used. Alcalase was the most effective enzyme in hydrolysing collagen, followed by collagenase and then proteinase K. This was shown both by the speed of increase in DH and by visual inspection of the reaction mixtures. After hydrolysis with Alcalase at 60°C for only 1.6 h, the DH of the hydrolysate was 11.9% (A4 in ), much higher than the DH obtained with the any of the other enzymes within 2 h (which were used at 37°C). A similar result was reported by Wasswa et al.,[Citation2] who obtained a DH of 10.4% after 110 min hydrolysis of grass carp skin with Alcalase. After prolonged hydrolysis of fish skin with Alcalase even higher DH values may be obtained.[Citation17] In our study, with collagenase, a similar DH as that obtained with Alcalase was reached; however, only after prolonged hydrolysis (19 h at 37°C, C3 in ). This is in accordance with the results obtained by Zhang et al.[Citation22] on the ability of these two enzymes to extensively hydrolyze bovine collagen, but at different rates. Visually, it could be seen that the small pieces of grass carp skin incubated with Alcalase at 60°C quickly dissolved and the initial slurry became a clear solution, while collagenase broke down the skin pieces only gradually. The efficiency of Alcalase in hydrolyzing fish skin collagen could partly be due to the higher incubation temperature at which the collagen was transformed from triple helix into random coil which made it more accessible to the enzyme. When the sample hydrolyzed with collagenase (C3) was further hydrolyzed by trypsin for 1 to 3 h, the DH increased to more than 13% (C3T1 and C3T2 in ).
After more than 10 h of hydrolysis with proteinase K, the DH of skin hydrolysates was only 3.4%. This is similar to the DH obtained after 4 h of hydrolysis of bovine collagen with the same enzyme (about 3%).[Citation22] The low DH obtained was consistent with the presence of big skin pieces at the end of the hydrolysis, The reason for the limited hydrolysis obtained with this enzyme could be that the native triple-helical structure of collagen is not accessible for proteinase K, or that the primary structure of collagen molecules has very few peptide bonds susceptible to cleavage by this enzyme. Proteinase K has a broad specificity with preferred cleavage of peptide bonds with aliphatic and aromatic amino acids at the P1 position (http.//web.expasy.org/peptide_cutter/peptidecutter_enzymes.html). The amino acid composition of the skin from grass carp used in the present study is shown in . Although the total amino acid content (36%) was much lower than the crude protein content (64%), it can be seen that the amount of hydrophilic amino acids of native grass carp skin protein significantly outweighed the amount of aliphatic and aromatic amino acids (about 3:1). This result, together with the similar results obtained by Wasswa et al.[Citation2] on native grass carp skin and by Zhang et al.[Citation5] on extracted collagen from grass carp skin, shows that the hydrophobic amino acids contribute up to 35–36% of total amino acids. In extracted gelatine from grass carp skin (which should be more accessible to proteolytic enzymes) these amino acids make up even less of the total protein (24%),[Citation4] which strongly indicates that the low DH obtained with proteinase K is mainly due to the low accessibility to the susceptible peptide bonds in the skin.
Table 2. Amino acid composition (g/100 g) of the freeze-dried skin from grass carp. Results are given as mean ± std (n = 5).
The peptide profiles of the fish skin hydrolysates ( and ) confirmed the superiority of the Alcalase enzyme in degrading the fish skin, since more material was solubilized in the hydrolysates made with this enzyme (; A1-A4). The action of this enzyme led to release of many peptides with medium retention times (15–45 min) in addition to some very hydrophilic peptides or free amino acids (retention time 2–5 min). With increasing time of hydrolysis and DH a slight right-to-left shift in the profile is seen due to larger peptides being degraded into shorter peptides. This was confirmed by the average mass spectra of these samples (not shown) showing that the major peptides were found in the mass range from 1000 to 2000 Da, and that with increasing hydrolysis time less peptides were seen in this mass range, in accordance with further degradation. A similar trend has been reported by Wasswa et al.[Citation2] for grass carp skin hydrolysates made with Alcalase.
Figure 1. Peptide profiles (LC with absorbance measurement at 210 nm) of grass carp skin hydrolyzed to various degrees with Alcalase (A1-A4), collagenase (C1-C3), and collagenase followed by trypsin (C3T1 and C3T2). Detailed hydrolytic conditions and resulting DH are given in .
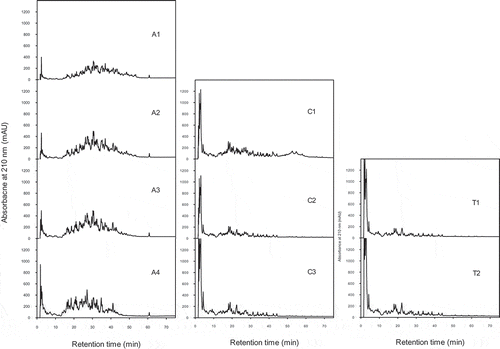
Figure 2. Peptide profiles (LC with absorbance measurement at 210 nm) of grass carp skin hydrolysates made with proteinase K (P1-P3) and a blank sample (B1) without enzyme but incubated under the same conditions as P3. Detailed hydrolytic conditions and DH are given in .
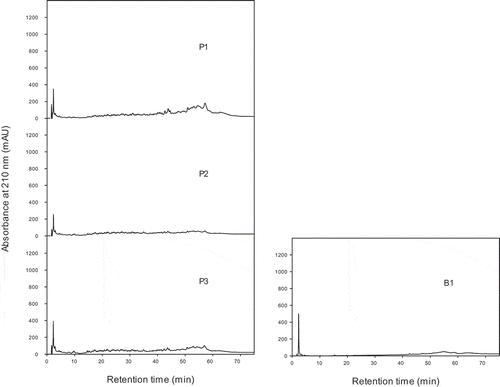
During collagenase catalyzed fish skin hydrolysis, large peptides were released first, which were subsequently further hydrolyzed (; C1-C3). Although the final DH was similar to that obtained with Alcalase, less medium-sized peptides and more small/hydrophilic peptides and/or amino acids were present (; C1-C3). Further hydrolysis with trypsin did not change the peptide profile (; C3T1 and C3T2), indicating that trypsin did not cleave the major peptides released by collagenase. The small increase in DH obtained during trypsin hydrolysis may be due to the release of more free amino acids or very small, hydrophilic peptides from minor peptides (retention time 2–5 min). During hydrolysis of the fish skin with proteinase K, primarily large peptides were released (; P1) and only a limited amount of medium-sized and small peptides were visible in the peptide profiles (; P1-P3), which is consistent with the low DH obtained (). In accordance with the low DH value of the blank sample (B1) a small amount of large peptides (retention time 45–70 min) had been released during incubation of the fish skin at 37°C (; B1). The blank samples incubated for longer time and at higher temperature contained even more polypeptidic material, which made them turbid to an extent that they could not be analyzed by LC-MS.
Bioactivity of hydrolysates
ACE-inhibitory activity
The unhydrolyzed samples did not show any ACE-I activity (not shown), in accordance with the fact that no small peptides had been liberated during incubation without enzymes (; B1). However, all hydrolysates obtained by enzymatic treatment exhibited a quite high inhibition of ACE (), showing that all enzymes used were able to release peptides from protein in fish skin which could inhibit ACE. With Alcalase there was a clear effect of hydrolysis, since the ACE-I activity increased with increasing hydrolysis time and DH (; A1-A4). A similar trend was seen with the other enzymatic treatments, and for all samples taken together a linear relationship between DH and ACE-I activity of hydrolysates was obtained (RCitation2 = 0.43). From the peptide profiles shown in and , it can be seen that the increased inhibitory activity with increasing DH is most likely due to further degradation of larger peptides into small peptides and free amino acids (retention time 2–5 min) during hydrolysis than to release of more material from the insoluble skin pieces. This is in line with other studies showing increased ACE-I activity with increasing DH (time of hydrolysis) or with fractions enriched in low molecular weight peptides.[Citation14,Citation15,Citation17,Citation25,Citation27,Citation28] This is probably due to release of a larger number of peptides (increased molar concentration) available for inhibition of ACE, but might also result from formation of smaller and more potent peptides. Indeed, many di-, tri- and oligopeptides from collagen, meat, fish, and other food proteins, have been shown to have strong ACE-I activity.[Citation3,Citation9]
Figure 3. ACE-inhibitory activity of fish skin hydrolysates obtained with Alcalase (A1-A4), collagenase (C1-C3), collagenase and trypsin (C3T1 and C3T2), and proteinase K (P1-P3). Hydrolysis conditions and resulting DH are given in . Samples were diluted (10×) before analysis. Data represent mean ± std (n = 3). Samples with different letters have significantly different activity (p < 0.05).
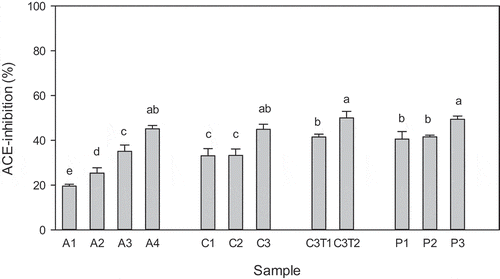
All the final hydrolysates, irrespective of the enzyme(s) used, showed ACE-I activities close to 50%. Assuming that the dried skin contains about 66% of protein and that the fish skin was completely hydrolyzed, which seems to be the case for the final Alcalase hydrolysate, the peptide concentration in this hydrolysate should be close to 7 mg mL−Citation1. Considering the dilutions, the maximum peptide concentration in the sample used was 0.7 mg mL−Citation1, corresponding to 0.12 mg mL−Citation1 in the final assay. The ACE-I values for the final hydrolysates being close to 50% show that the IC50 for the Alcalase hydrolysate is approximately 0.12 mg mL−Citation1, and even lower for the hydrolysates made with the other enzymes which did not completely dissolve all protein from the skin. These activities are quite good in comparison to those obtained for crude hydrolysates made by Alcalase hydrolysis of other fish skin sources[Citation14,Citation15,Citation17] presenting IC50 values ranging from 0.7 to 5 mg mL−Citation1. Hydrolysates of grass carp scales, sea bream scales, and sardinelle heads and viscera also showed lower ACE-I activity, presenting IC50 values of 1.66,[Citation29] 0.57,[Citation30] and 1.2 mg mL−Citation1,[Citation31] respectively. Only enriched fractions from grass carp scale hydrolysates showed a similar activity (IC50 = 0.13 mg mL−Citation1)[Citation29] and hydrolysates of salmon and cod skin, performed as simulated gastro-intestinal digestion, showed a slightly higher activity (IC50 = 0.10 mg mL−Citation1).[Citation32] The generally higher activity of hydrolysates from fish skin and scales in comparison to heads and viscera might be related to their high content of collagen. Collagen is rich in Pro residues which, when present in the C-terminal sequence of released peptides, are associated with high ACE-I activity.[Citation22,Citation33,Citation34]
Surprisingly, the hydrolysates obtained with proteinase K also had high ACE-I activity even though the DH obtained () and the amount of material present () were much lower than in the other hydrolysates. Blank samples incubated with proteinase K for various times did not show significant ACE-I activity (not shown), excluding that low molecular weight compounds in the enzyme preparation could contribute to the high ACE-I activity of these hydrolysates. Other studies have also found proteinase K to be one of the best enzymes for release of ACE-I peptides.[Citation22,Citation33,Citation35] This can be explained by the specificity of the enzyme which, as mentioned above, would lead to the release of peptides containing hydrophobic C-terminal amino acid residues, either aromatic (Trp, Tyr, Phe) or aliphatic residues (Ala, Ile, Leu, Pro, Val). These residues, when present at the C-terminal of peptides, have been shown to promote binding to ACE, and such peptides thus represent efficient inhibitors in vitro.[Citation3,Citation9,Citation22,Citation28,Citation36,Citation37] Furthermore, it has been reported that di- and tri-peptides with C-terminal proline or hydroxyproline are generally resistant to degradation by digestive enzymes.[Citation37,Citation38] Thus, grass carp collagen hydrolysates made by proteinase K should have a high content of peptides with terminal proline and hydroxyproline residues and should accordingly be stable toward gastro-intestinal digestion and may also demonstrate good ACE-inhibitory activity in vivo.
Antioxidant activity
The antioxidant activity of the fish skin hydrolysates tested by different mechanisms of reaction is shown in . All enzymatic hydrolysates were able to scavenge ABTS radicals () to a higher extent than the control samples incubated without enzymes (B2 and B3 as controls for A1-A4 samples and B1 for the other samples). However, the effect of the enzymatic treatments was not correlated to the DH of the resulting samples (RCitation2 = 0.18 for all samples). The highest ABTS radical scavenging activity, considering all hydrolysis times, was obtained with Alcalase and with a combination of collagenase and trypsin (p > 0.05). Similar results have been reported by Alemán et al.[Citation7] showing that the Alcalase hydrolysates of tuna and squid gelatine showed the highest ABTS radical scavenging activity, followed by trypsin and/or collagenase hydrolysates.
Figure 4. Antioxidant activity of fish skin hydrolysates as assayed by A: ABTS radical scavenging, B: iron chelation, and C: inhibition of lipid oxidation in liposomes. Hydrolysates were obtained with Alcalase (A1-A4), collagenase (C1-C3), collagenase and trypsin (C3T1 and C3T2), and proteinase K (P1-P3), respectively; as well as without enzyme (B1-B3). Hydrolysis conditions and resulting DH are given in . Samples were diluted 50× before the radical scavenging assay (a), and 5× before the chelation and liposome assays (b and c). Data represent mean ± std (n = 3). Samples with different letters have significantly different activity (p < 0.05).
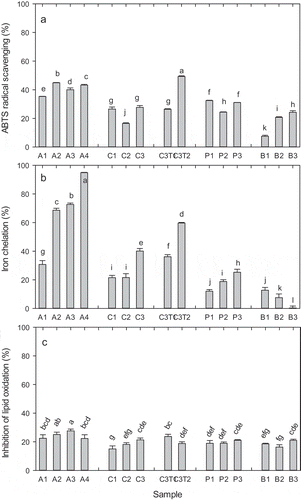
In this study, the Alcalase hydrolysate with intermediate hydrolysis degree (DH = 6.5%, A2) showed the highest ABTS radical scavenging activity. Other authors investigating the antioxidant activity of Alcalase hydrolysates of fish sources found that the ability to scavenge DPPH and/or ABTS increased with DH up to 30 or 40%,[Citation7,Citation39,Citation40] whereas the study of Klompong et al.[Citation41] shows no increase in the DPPH radical scavenging activity for Alcalase hydrolysates of yellow stripe trevally meat with increasing DH from 5 to 15%. The latter study is in agreement with the results of this study, and indicates that peptides of intermediate size have higher radical scavenging activity than small peptides, which could be due to the peptide bonds playing a role in delocalization of the radical formed in the peptide upon donation of a hydrogen/electron to the ABTS/DPPH radical.
Interestingly, the hydrolysate obtained by hydrolysis with collagenase followed by trypsin (C3T2) showed the highest ABTS radical scavenging activity of all samples (). This indicates that peptides resulting from trypsin hydrolysis, containing Lys or Arg residues at the C-terminal, improve the radical scavenging activity. These results are consistent with the fact that the peptide Ala-His-Lys and other peptides with C-terminal Lys have been shown to be potent antioxidant peptides.[Citation21]
The release of peptides with Trp or Tyr at the C-terminus confering strong radical scavenging activity[Citation21] despite their very low DH () is probably also the reason why the proteinase K hydrolysates showed significantly higher ABTS radical scavenging activity than the hydrolysates made with collagenase alone.
The highest ABTS radical scavenging activity in the present study was 50% (exerted by the C3T2 hydrolysate). Others have investigated the ABTS radical scavenging activity of fish gelatine and meat hydrolysates,[Citation7,Citation13,Citation19,Citation39,Citation40,Citation42,Citation43] however, they have all reported the results as Trolox equivalents or ascorbic acid equivalents per gram of gelatine, and their results are thus not directly comparable to our results. As mentioned previously, the maximum protein concentration possible in the hydrolysate is 7 mg mL−Citation1, and considering the dilutions, the maximum IC50 of this sample (C3T2) is 28 μg mL−Citation1 in the reaction mixture. This is probably in the same range as the activity obtained by Cheung et al.,[Citation19] who evaluated the antioxidant activity of pacific hake fillet hydrolysates produced with a number of commercial enzymes, among them an Alcalase hydrolysate with 80% ABTS radical scavenging at 67 μg mL−Citation1. Gomez-Guillen et al.[Citation44] showed by multivariate data analysis that the antioxidant activity of fractions from tuna and squid skin hydrolysates (made with Alcalase) correlated with the presence of the amino acids Thr, Leu, Ile, Hyl, Tyr, and Arg (in that order), while larger contents of Pro, Gly, and Ala correlated with a decreased activity. This could also explain why skin hydrolysates had slightly lower activity than casein hydrolysates as obtained with cold-active microbial enzymes (80% ABTS scavenging at 12 μg mL−Citation1).[Citation25]
The ability to scavenge radicals is only one of the mechanisms by which protein hydrolysates could contribute to inhibit lipid oxidation in food systems. Another way to prevent lipid oxidation in food and generation of unwanted secondary lipid oxidation products is by chelating iron ions since complex-binding of catalytic transition metal ions blocks one-electron cleavage of hydroperoxides, the primary lipid oxidation products.[Citation45]
All enzymatic hydrolysates from fish skin showed iron chelation capacity (), with the Alcalase hydrolysates showing proportionately higher activity, reaching almost 100% in the A4 hydrolysate. Dong et al.[Citation46] also found a high iron chelation activity (80–90%) in an Alcalase hydrolysate of silver carp protein, which was higher than the activity obtained with the Flavourzyme hydrolysate (up to 60%). Yarnpakdee et al.[Citation40] also found the highest iron chelating activity in the Alcalase hydrolysates of Nile tilapia protein, except at high DH (30–40%). In contrast, Klompong et al.[Citation41] and Cheung et al.[Citation19] found a slightly higher activity of the Flavourzyme hydrolysates than of the Alcalase hydrolysates of yellow stripe trevally and Pacific hake fillet, respectively. This is probably due to the varying amino acid composition and sequences of the fish substrates used in the various studies. The high activity obtained after hydrolysis with Alcalase in the present study, is probably due to release of a higher amount of peptides (), as well as to the type of the peptides released by Alcalase, having a sequence and conformation that favour binding of iron. Alcalase is a serine endoprotease with a broad specificity. The preferred cleavage sites in whey proteins have been shown to be Leu-X followed by Ala-X, Phe-X, Ser-X, Glu-X, and Lys-X bonds.[Citation47] It has been reported that the carboxyl and amino groups in the side chains of acidic and basic amino acids are thought to play an important role in chelating metal ions.[Citation48] This could explain why both the collagenase hydrolysate post-treated with trypsin and the Alcalase hydrolysates exhibited higher ion chelating activity than the other hydrolysates, since the former enzymes have specificities to release such amino acid residues at the terminals.
Overall, the iron chelation capacity increased with DH (RCitation2 = 0.43 for all samples). Other authors assessing the iron chelating capacity of fish protein hydrolysates have also found a linear relationship between iron chelation activity and DH up to 30% in Alcalase hydrolysates of yellow stripe trevally, silver carp, and Nile tilapia protein, respectively,[Citation40,Citation41,Citation46] as well as for other hydrolysates of fish protein.[Citation13,Citation39] This is probably due to a combination of more material being released from the skin during hydrolysis and the smaller size of peptides () generating more carboxylic groups (C-terminals) which might aid in chelating the iron ions.
The highest iron chelation capacity (95%) was obtained with the grass carp skin hydrolyzed with Alcalase to the highest DH (12%). Assuming complete hydrolysis of all protein in the fish skin and taking into account the dilutions, the protein concentration in this sample could maximally be 2.8 mg mL−Citation1 (0.28 mg mL−Citation1 in the assay). Other studies reporting iron chelation activities of fish protein hydrolysates mostly report their results as ethylenediaminetetraacetic acid equivalents,[Citation13,Citation40,Citation42] which does not allow direct comparison of results. Dong et al.[Citation46] reported a similar high iron chelation activity (80–90%) in samples of silver carp muscle protein hydrolyzed with Alcalase for more than 30 min when assayed at a sample concentration of 5 mg mL−Citation1. Cheung et al.[Citation19] reported the iron chelating activity of Alcalase hydrolysates of pacific hake fillet to be 51.5% when assayed at 3 mg mL−Citation1. The comparison with the previously mentioned studies clearly demonstrates the high metal chelating activity of the grass carp skin hydrolysates obtained in the present study.
Despite the high radical scavenging and iron chelation activities, the fish skin hydrolysates were able to inhibit lipid oxidation in a liposome model system to a limited extent only (20–30%, ), even if there was a significant effect of the enzymatic treatment (p < 0.001). Overall, the inhibitory activity was not linearly dependent on DH (RCitation2 = 0.08 for all samples), as also indicated by the blank samples incubated without proteolytic enzymes being as efficient as most of the enzymatically hydrolyzed samples. A possible reason is that, in food emulsion systems, proteins and peptides in protein hydrolysates can locate at the oil–water interface due to their surface active properties and form a physical barrier to minimize the contact of lipids with oxidizing agents in the aqueous phase, and in this way also contribute to reducing lipid peroxidation.[Citation21] Accordingly, hydrophobic amino acid residues, such as Val, Leu, and Pro can increase the presence of peptides at the water–lipid interface and, therefore, facilitate scavenging of free radicals generated in the lipid.[Citation10,Citation21] Furthermore, the Ala residue of Ala-His and Ala-His-Lys peptides has been shown to be critical for their antioxidant activity.[Citation21] Collagen is rich in Pro and Ala, and, therefore, peptides released, even in the absence of enzymes, are expected to be able to interact with the liposomes.
The Alcalase hydrolysates gave rise to higher inhibition than the other enzyme hydrolysates, including the blank samples (p < 0.05). This is consistent with the higher radical scavenging and iron chelating activities of Alcalase hydrolysates compared to the samples made with the other enzymes (except C3T2, ), which all could be related to the higher amounts of peptides released with this enzyme (), or to the release of some peptides with higher antioxidant activity. Alcalase has also been found in other studies to be among the best enzymes for release of peptides from fish skin gelatine and fish meat that can inhibit lipid oxidation in other model systems.[Citation18,Citation19,Citation23,Citation39,Citation40,Citation46,Citation49,Citation50]
With each enzymatic treatment there was a tendency for increasing activity with increasing DH (), and only for the samples with the highest DH (A4 with DH≈12% and C3T2 with DH≈14%, ), the antioxidative effect was diminished. The Alcalase hydrolysates A2 and A3 were the only samples that inhibited lipid oxidation to a greater extent than the blank samples, especially the A3 hydrolysate with DH 8.1 (27% inhibition of lipid oxidation). This could indicate that in the A4 sample some of the intermediary active peptides had been cleaved into small peptides and/or free amino acids with less protecting activity. Further cleavage of peptides will remove hydrophobic amino acid residues and, therefore, reduce the hydrophobicity and interaction with liposomes. Dong et al.[Citation46] investigating the antioxidant activity of Alcalase hydrolysates of silver carp muscle also found that the maximal inhibition of linoleic acid oxidation occurred after limited hydrolysis (1.5 h, DH not given). This is also consistent with results from other studies on cod protein hydrolysates (enzyme not indicated) where the highest inhibition of lipid oxidation in a liposome model was obtained with peptides of intermediate size (3–5 kDa)[Citation51] and on casein hydrolysates, where limited hydrolysis led to the highest inhibition.[Citation25]
The values for inhibition of lipid oxidation (<30%) obtained in the present study with the liposome model system are similar to the results obtained for casein hydrolysates using the same assay.[Citation25] However, in other studies on the antioxidant properties of various fish protein hydrolysates, using linoleic acid model systems, the inhibitory effect on lipid oxidation has been reported to be around 50%[Citation50] or higher, [Citation12,Citation23,Citation52,Citation53] which might be related primarily to a higher peptide concentration used in those studies, although many of them do not report the protein concentration.[Citation12,Citation46,Citation54] In our study, the fish skin hydrolysates obtained with Alcalase contained maximum 7 mg of protein mL−Citation1 and these were diluted 5 times resulting in 1.4 mg mL−Citation1 in the sample and 0.37 mg mL−Citation1 in the assay. This is lower than concentrations used by Jridi et al.,[Citation23] 5 mg mL−Citation1 in the samples (1.9 mg mL−Citation1 in the reaction mixture). Ren et al.[Citation18] reported that the IC50 for an Alcalase hydrolysate of grass carp muscle for inhibition of lipid peroxidation in a liver homogenate was 4.6 mg mL−Citation1 in the sample, which could explain the inhibitory values below 50% in our study.
Due to the ability of such hydrolysates from fish skin and other sources to delay lipid oxidation in model systems kept for several days, and also in model food systems,[Citation13,Citation23] they might have a potential for use as natural antioxidants in foods, for instance fish oil-enriched foods. Furthermore, several studies have shown that simulated gastrointestinal digestion improves the antioxidant activity, indicating that possible health benefits upon ingestion may occur.
Antimicrobial activity
Organisms produce antimicrobial peptides as components of the non-specific host-defence system, predominantly in tissues which are most likely to come in contact with microorganisms, such as skin, eyes, and lungs.[Citation55] Moreover, a number of hydrolysates of milk proteins and other bovine protein sources have shown antimicrobial activity against gram-negative and gram-positive bacteria.[Citation10] It was, therefore, surprising that, in the present study, no antimicrobial activity was detected in any of the fish skin hydrolysates against the microorganisms used, i.e., strains of L. monocytogenes, S. aureus, P. aeruginosa, E. coli 1873, and E.coli JM109. In fact, very few studies have reported antimicrobial activity of hydrolysates from marine sources,[Citation10] and to the best of our knowledge only one study has reported antimicrobial activity of hydrolysates from fish collagen or gelatine sources. Gomez-Guillen et al.[Citation44] found that fractions (<3kDa and 1–10 kDa) from Alcalase hydrolysates of skin from squid and tuna exerted antimicrobial activity against a range of G+ and G− microorganisms at 2 mg mL−Citation1 (2 mL spotted on the agar plate). However, no or only a weak effect was observed against the strains used from E. coli and P. aeroginosa and against Listeria innocua, which were closest to the strains used in the present study. In contrast, good antimicrobial activity was observed against S. putrefaciens, even at 0.2 mg mL−Citation1. In our study, the hydrolysates with maximum 7 mg protein mL−Citation1 were directly spotted in the wells in the agar plate (10 μL per well) and, therefore, the concentrations used in the present study were similar to those used by Gomez-Guillen et al.[Citation44] Probably the microbial strains used in the present study were not very sensitive to small amounts of antimicrobial peptides which might occur in the hydrolysate and may be masked by other peptides present. De Gobba et al.[Citation25] using the same method and the same strains also did not detect any antimicrobial activity of hydrolysates from casein, which is a known source of antimicrobial peptides.[Citation10]
Conclusions
Fish skin from grass carp can serve as a direct substrate for release of bioactive peptides by enzymatic treatment. By use of Alcalase, proteinase K, and collagenase (alone or followed by trypsin), the skin was completely or partially hydrolyzed resulting in liberation of bioactive peptides and possibly free amino acids. Both Alcalase and collagenase resulted in extensive hydrolysis with a DH of 12%. Peptides that could inhibit ACE were released by all enzymes, depending on DH, and IC50 values around 120 μg mL−Citation1 was obtained for the Alcalase hydrolysate. The specificity of proteinase K seemed to be optimal for generation of ACE-inhibitory peptides. All enzymes also released peptides with antioxidant activity. Alcalase and the combination of collagenase and trypsin resulted in hydrolysates with the highest capacity to chelate iron and scavenge radicals in aqueous solutions, the former increasing with DH. Although the same hydrolysates also resulted in the strongest inhibition of lipid oxidation in liposomes, this was obtained at an intermediate DH, probably due to further degradation of the active hydrophobic peptides at higher DH. None of the enzymes, however, released hydrolysates exerting antimicrobial activity in the tests performed. The above results show that fish skin, an industrial by-product, can be exploited by enzymatic hydrolysis producing hydrolysates with strong ACE-inhibitory activity and also antioxidant activity. Such hydrolysates could potentially be applied in functional foods to alleviate moderate hypertension, and also to increase the shelf life of the food products.
Acknowledgments
The authors would like to thank the Huzhou Nanxun Xinya Produce Company, Zhejiang, China, for providing the fish samples. Yuhao Zhang, Anders H. Karlsson, Henriette Erichsen, Kirsten Sjøstrøm, and Finn K. Vogensen at the Department of Food Science, University of Copenhagen, are appreciated for their help with experimental work and data analysis, and Colin Ray from the same department is thanked for proofreading of the English language.
Funding
The financial support from the Danish Strategic Research Council through the NOVENIA project is gratefully acknowledged.
Additional information
Funding
References
- China Statistical Yearbook. http://www.stats.gov.cn/tjsj/ndsj/2014/zk/html/Z1215e.htm (2014). Accessed March 04, 2016.
- Wasswa, J.; Tang, J.; Gu, X.; Yuan, X.-Q. Influence of the Extent of Enzymatic Hydrolysis on the Functional Properties of Protein Hydrolysate from Grass Carp (Ctenopharyngodon Idella) Skin. Food Chemistry 2007, 104(4), 1698–1704.
- Gómez-Guillén, M.C.; Giménez, B.; López-Caballero, M.E.; Montero, M.P. Functional and Bioactive Properties of Collagen and Gelatin from Alternative Sources: A Review. Food Hydrocolloids 2011, 25, 1813–1827.
- Kasankala, L.M.; Xue, Y.; Weilong, Y.; Hong, S.D.; He, Q. Optimization of Gelatine Extraction from Grass Carp (Catenopharyngodon Idella) Fish Skin by Response Surface Methodology. Bioresource Technolology 2007, 98(17), 3338–3343.
- Zhang, Y.; Liu, W.; Li, G.; Shi, B.; Miao, Y.; Wu, X. Isolation and Partial Characterization of Pepsin-Soluble Collagen from the Skin of Grass Carp (Ctenopharyngodon Idella). Food Chemistry 2007, 103(3), 906–912.
- Wang, L.; Yang, B.; Wang, R.; Du, X. Extraction of Pepsin-Soluble Collagen from Grass Carp (Ctenopharyngodon Idella) Skin Using an Artificial Neural Network. Food Chemistry 2008, 111(3), 683–686.
- Alemán, A.; Giménez, B.P.; Montero, P.; Gómez-Guillén, M.C. Antioxidant Activity of Several Marine Skin Gelatins. LWT–Food Science and Technology 2011, 44, 407–413.
- Ngo, D.H.; Ryu, B.; Vo, T.S.; Himaya, S.W.; Wijesekara, I.; Kim, S.K. Free Radical Scavenging and Angiotensin-I Converting Enzyme Inhibitory Peptides from Pacific Cod (Gadus Macrocephalus) Skin Gelatin. International Journal of Biological Macromoles 2011, 49(5), 1110–1116.
- Wilson, J.; Hayes, M.; Carney, B. Angiotensin-I-Converting Enzyme and Prolyl Endopeptidease Inhibitory Peptides from Natural Sources with a Focus on Marine Processing by-Products. Food Chemistry 2011, 129, 235–244.
- Di Bernadini, R.; Harnedy, P.; Bolton, D.; Kerry, J.; O’Neill, E.; Mullen, A.M.; Hayes, M. Antioxidant and Antimicrobial Peptidic Hydrolysates from Muscle Protein Sources and by-Products. Food Chemistry 2011, 124, 1296–1307.
- Suarez-Jimenez, G.-M.; Burgos-Hernandez, A.; Ezquerra-Brauer, J.-M. Bioactive Peptides and Depsipeptides with Anticancer Potential, Sources from Marine Animals. Marine Drugs 2012, 10, 963–986.
- Mendis, E.; Rajapakse, N.; Kim, S.K. Antioxidant Properties of a Radical-Scavenging Peptide Purified from Enzymatically Prepared Fish Skin Gelatin Hydrolysate. Journal of Agricultural and Food Chemistry 2005, 53(3), 581–587.
- Kittiphattanabawon, P.; Benjakul, S.; Visessanguan, W.; Shahidi, F. Gelatin Hydrolysate from Blacktip Shark Skin Prepared Using Papaya Latex Enzyme, Antioxidant Activity and Its Potential in Model Systems. Food Chemistry 2012, 135(3), 1118–1126.
- Byun, H.G.; Kim, S.K. Purification and Characterization of Angiotensin I Converting Enzyme (ACE) Inhibitory Peptides from Alaska Pollack (Theragra Chalcogramma) Skin. Process Biochemistry 2001, 36, 1155–1162.
- Mahmoodani, F.; Ghassem, M.; Babji, A.S.; Yusop, S.M. Bioactive Peptides Isolated from Enzymatic Hydrolysate of Catfish (Pangasius Sutchi) Skin Gelatin, Proceedings from the 58th International Congress of Meat Science and Technology, Montreal, Canada; NUTRIENTP-3, Aug 12–17, 2012.
- Pampanin, D.M.; Larssen, E.; Provan, F.; Sivertsvik, M.; Ruoff, P.; Sydnes, M.O. Detection of Small Bioactive Peptides from Atlantic Herring (Clupeaharengus L.). Peptides 2012, 34(2), 423–426.
- Lee, J.K.; Jeon, J.-K.; Byun, H.-G. Antihypertensive Effect of Novel Angiotensin I Converting Enzyme Inhibitory Peptide from Chum Salmon (Oncorhyncus Keta) Skin in Spontaneously Hypertensive Rats. Journal of Functional Foods 2014, 7, 381–389.
- Ren, J.; Zhao, M.; Shi, J.; Wang, J.; Jiang, Y.; Cui, C.; Kakuda, Y.; Xue, S.J. Purification and Identification of Antioxidant Peptides from Grass Carp Muscle Hydrolysates by Consecutive Chromatography and Electrospray Ionization-Mass Spectrometry. Food Chemistry 2008, 108, 727–736.
- Cheung, I.W.Y.; Cheung, L.K.Y.; Tan, N.Y.; Li-Chan, C.Y. The Role of Molecular Size in Antioxidant Activity of Peptide Fractions from Pacific Hake (Merluccius Productus) Hydrolysates. Food Chemistry 2012, 134, 1297–1306.
- Ryan, J.T.; Ross, R.P.; Bolton, D.; Fitzgerald, G.F.; Stanton, C. Bioactive Peptides from Muscle Sources, Meat and Fish. Nutrients 2011, 3, 765–791.
- Samaranayaka, A.; Li-Chan, E. Food-Derived Peptidic Antioxidants: A Review of Their Production; Assessment; and Potential Applications. Journal of Functional Foods 2011, 3, 229–254.
- Zhang, Y.; Olsen, K.; Grossi, A.; Otte, J. Effect of Pretreatment on Enzymatic Hydrolysis of Bovine Collagen and Formation of ACE-Inhibitory Peptides. Food Chemistry 2013, 141, 2343–2354.
- Jridi, M.; Lassoued, I.; Nasri, R.; Ayadi, M.A.; Nasri, M.; Souissi, N. Characterisation and Potential Use of Cuttle Fish Skin Gelatin Hydrolysates Prepared by Different Microbial Proteases. BioMedical Research International Article ID 461728, 14 pages; doi:10.1155/2014/461728
- See, S.F.; Hoo, L.L.; Babji, A.S. Optimization of Enzymatic Hydrolysis of Salmon (Salmo Salar) Skin by Alcalase. International Food Research Journal 2011, 18(4), 1359–1365.
- De Gobba, C.; Tompa, G.; Otte, J. Bioactive Peptides from Caseins Released by Cold-Active Proteolytic Enzymes from Arsukibacterium Ikkense. Food Chemistry 2014, 165, 205–215.
- Gómez-Guillén, M.C.; Perez-Mateos, M.; Gomez-Estaca, J.; López-Caballero, E.; Giménez, B.; Montero, P. Fish Gelatin, A Renewable Material for Developing Active Biodegradable Films. Trends Food Science and Technology 2009, 20, 3–16.
- Kim, S.-K.; Byun, H.-G.; Park, P.-J.; Shahidi, F. (a). Angiotensin I Converting Enzyme Inhibitory Peptides Purified from Bovine Skin Gelatin Hydrolysate. Journal of Agricultural and Food Chemistry 2001, 49, 2992–2997.
- Espejo-Carpio, F.J.; De Gobba, C.; Guadix, A.; Guadix, E.M.; Otte, J. Angiotensin I-Converting Enzyme Inhibitory Activity of Enzymatic Hydrolysates of Goat Milk Protein Fractions. International Dairy Journal 2013, 32, 175–183.
- Zhang, F.; Wang, Z.; Xu, S. Macroporous Resin Purification of Grass Carp Fish (Ctenopharyngodon Idella) Scale Peptides with in Vitro Angiotensin-I Converting Enzyme (ACE) Inhibitory Ability. Food Chemistry 2009, 117(3), 387–392.
- Fahmi, A.; Morimura, S.; Guo, H.C.; Shigematsu, T.; Kida, K.; Uemura, Y. Production of Angiotensin I Converting Enzyme Inhibitory Peptides from Sea Bream Scales. Process Biochemistry 2004, 39, 1195–1200.
- Bougatef, A.; Nedjar-Arroume, N.; Ravallec-Plé, R.; Leroy, Y.; Guillochon, D.; Barkia, A.; Nasri, M. Angiotensin I-Converting Enzyme (ACE) Inhibitory Activities of Sardinelle (Sardinella Aurita) by-Products Protein Hydrolysates Obtained by Treatment with Microbial and Visceral Fish Serine Proteases. Food Chemistry 2008, 111, 350–356.
- Dragnes, B.T.; Stormo, S.K.; Larsen, R.; Ernstsen, H.H.; Elvevoll, E.O. Utilisation of Fish Industry Residuals, Screening the Taurine Concentration and Angiotensin Converting Enzyme Inhibition Potential in Cod and Salmon. Journal of Food Composition and Analysis 2009, 22(7–8), 714–717.
- Abubakar, A.; Saito, T.; Kitazawa, H.; Kawai, Y.; Itoh, T. Structural Analysis of New Antihypertensive Peptides Derived from Cheese Whey Protein by Proteinase K Digestion. Journal of Dairy Science 1998, 81(12), 3131–3138.
- Ghassem, M.; Arihara, K.; Babji, A.S.; Said, M.; Ibrahim, S. Purification and Identification of ACE Inhibitory Peptides from Haruan (Channa Striatus) Myofibrillar Protein Hydrolysate Using HPLC–ESI-TOF MS/MS. Food Chemistry 2011, 129(4), 1770–1777.
- Fernández-Musoles, R.; Salom, J.B.; Martínez-Maqueda, D.; López-Díez, J.J.; Recio, I.; Manzanares, P. Antihypertensive Effects of Lactoferrin Hydrolyzates, Inhibition of Angiotensin- and Endothelin-Converting Enzymes. Food Chemistry 2012, 139, 994–1000.
- Otte, J.; Shalaby, S.M.A.; Zakora, M.; Nielsen, M.S. Fractionation and Identification of ACE-Inhibitory Peptides from α-lactalbumin and β-Casein Produced by Thermolysin-Catalysed Hydrolysis. International Dairy Journal 2007, 17, 1460–1472.
- Norris, R.; FitzGerald, R.J. Antihypertensive Peptides from Food Proteins, Chapter 3. In Bioactive Food Peptides in Health and Disease; Hernández-Ledesma. B; Hsieh C.-C; Eds.; InTech: Rijeka; Croatia, 2013 ( available online).
- Vermeirssen, V.; Van Camp, J.; Verstraete, W. Bioavailability of Angiotensin-I-Converting Enzyme Inhibitory Peptides. British Journal of Nutrition 2004, 92, 357–366.
- Sai-Ut, S.; Benjakul, S.; Sumpavapol, P.; Kishimura, H. Antioxidant Activity of Gelatin Hydrolysate Produced from Fish Skin Gelatin Using Extracellular Protease from Bacillus Amyloliquefaciens H11. Journal Food Processing and Preservation 2014, doi: 10.1111/jfpp.12244
- Yarnpakdee, S.; Benjakul, S.; Kristinsson, H.G.; Kishimura, H. Antioxidant and Sensory Properties of Protein Hydrolysates Derived from Nile Tilapia (Oreochromis Niloticus) by One- and Two-Step Hydrolysis. Journal of Food Science and Technology 2015, 52(6), 3336–3349.
- Klompong, V.; Benjakul, S.; Kantachote, D.; Shahidi, F. Antioxidative Activity and Functional Properties of Protein Hydrolysate of Yellow Stripe Trevally (Selaroides Leptolepis) as Influenced by the Degree of Hydrolysis and Enzyme Type. Food Chemistry 2007, 102, 1317–1327.
- Wiriyaphan, C.; Xiao, H.; Decker, E.; Yongsawatdigul, J. Chemical and Cellular Antioxidative Properties of Threadfin Bream (Nemipterus sp.) surimi Byproduct Hydrolysates Fractionated by Ultrafiltration. Food Chemistry 2015, 167, 7–15.
- Khantaphant, S.; Benjakul, S. Comparative Study on the Proteases from Fish Pyloric Caeca and the Use for Production of Gelatin Hydrolysate with Antioxidative Activity. Comparative Biochemistry and Physiology Part B. Biochemistry and Molecular Biology 2008, 151(4), 410–419.
- Gómez-Guillén, M.C.; López-Caballero, M.E.; Alemán, A.; Lopez de Lacey, A.; Giménez, B.; Montero, P. Antioxidant and Antimicrobial Peptide Fractions from Squid and Tuna Skin Gelatin, Chapter 7. In Sea By-Products as Real Material, New Ways of Application; Le Bihan, E. Ed.; Transworld Research Network; Kerala, India, 2010.
- Skibsted, L.H. Antioxidant Evaluation Strategies, Chapter 10. In Lipid Oxidation Pathways; Vol 2; Kamal-Eldin, A.; Min, D.B.; Eds.; AOACS Press: Urbana, IL, 2008.
- Dong, S.; Zeng, M.; Wang, D.; Liu, Z.; Zhao, Y.; Yang, H. Antioxidant and Biochemical Properties of Protein Hydrolysates Prepared from Silver Carp (Hypophtalmichthys Molitrix). Food Chemistry 2008, 107, 1485–1493.
- Doucet, D.; Otter, D.E.; Gauthier, S.F.; Foegeding, E.A. Enzyme-Induced Gelation of Extensively Hydrolysed Whey Proteins by Alcalase, Peptide Identification and Determination of Enzyme Specificity. Journal of Agricultural and Food Chemistry 2003, 51(21), 6300–6308.
- Saiga, A.; Tanabe, S.; Nishimura, T. Antioxidant Activity of Peptides Obtained from Porcine Myofibrillar Proteins by Protease Treatment. Journal of Agricultural and Food Chemistry 2003, 51, 3661–3667.
- Kim, S.-K.; Kim, Y.-T.; Byun, H.-G.; Nam, K.-S.; Joo, D.-S.; Shahidi, F. (b) Isolation and Characterization of Antioxidative Peptides from Gelatin Hydrolysate of Alaska Pollack Skin. Journal of Agricultural and Food Chemistry 2001, 49, 1984–1989.
- Sathivel, S.; Bechtel, P.J.; Babbitt, J.; Smiley, S.; Crapo, C.; Reppond, K.D.; Prinyawiwatkul, W. Biochemical and Functional Properties of Herring (Clupea Harengus) Byproduct Hydrolysates. Journal of Food Science 2003, 68(7), 2196–2200.
- Farvin, K.H.S.; Andersen, L.L.; Nielsen, H.H.; Jacobsen, C.; Jakobsen, G.; Johansson, I.; Jensen, F. Antioxidant Activity of Cod (Gadus Morhua) Protein Hydrolysates, in Vitro Assays and Evaluation in 5% Oil-in-Water Emulsion. Food Chemistry 2014, 149, 326–334.
- Je, J.-Y.; Park, P.-J.; Kim, S.-K. Antioxidant Activity of a Peptide Isolated from Alaska Pollack (Theragra Chalcogramma) Frame Protein Hydrolysate. Food Research International 2005, 38, 45–50.
- Yang, J.L.; Ho, H.Y.; Chu, Y.J.; Chow, C.J. Characteristic and Antioxidant Activity of Retorted Gelatin Hydrolysates from Cobia (Rachycentron Canadum) Skin. Food Chemistry 2008, 110, 128–136.
- Wu, H.-C.; Chen, H.-M.; Shiau, C.-Y. Free Amino Acids and Peptides as Related to Antioxidant Properties in Protein Hydrolysates of Mackerel (Scomber Austriasicus). Food Research International 2003, 36, 949–957.
- Rydlo, T.; Miltz, J.; Mor, A. Eukaryotic Antimicrobial Peptides, Promises and Premises in Food Safety. Journal of Food Science 2006, 71(9), R125–R135.