ABSTRACT
This study describes the development of an electrochemical sensor system for the determination of bisphenol A using tannic acid functionalized CuO nanoglobules. The utilized tannic acid acted simultaneously as both bio-compatible template and functionalizing agent for the as-synthesised CuO nanostructures. The excellent synergy of high surface area and favorable interactions between the moieties of tannic acid and bisphenol A molecules enabled sensitive electrochemical oxidation of bisphenol A within wide detection window (0.1–5.5 µM) and limit of detection estimated to be 0.01 µM. Moreover, the sensor exhibited satisfactory stability and strong anti-interference capability when evaluated against common interferents molecules such as phenol, hydroquinone, and 4-nitrophenol.
Introduction
The growing demand of the plastic commodities in the daily life style has resulted in a perpetual increment in the production of polycarbonate plastics.[Citation1,Citation2] Such plastics are usually produced by the application of chemicals considered highly toxic and detrimental both to humans and aquatic environment. In particular, bisphenol A (BPA), an indirect food additive is the most common and widely used material for the production of such plastics.[Citation3] Although, the usage of this material provides strength, toughness, and transparency to the manufactured plastic, BPA is known to leach during the washing of plastic commodities which as a consequence leads to its accumulation in the environment.[Citation4] In this context, accurate determination of BPA is an important issue when it comes to monitoring and regulating such toxins within the environment. Although, traditional techniques such as fluorescence, high-performance liquid chromatography (HPLC), liquid chromatography-mass spectrometry (LC-MS), gas chromatography-mass spectrometry (GC-MS) and Enzyme-linked Immunosorbent Assay (ELISA) are highly sensitive and efficient toward determination of BPA with impressive detection limit extending to nanomolar concentration.[Citation5–Citation9] However, the associated lengthy protocols, higher analysis cost and complexity restricts the wide usage of such techniques. In contrast, the electrochemical approach provides much analytical privileges with simple, sensitive, and yet cheap route for the quantitative determination of such molecules. In addition to this, electrochemical approach unlike above mentioned conventional techniques also provides the advantage of miniaturization. Although BPA is an electro-active molecule, but the direct electrochemical detection is usually accompanied by the electrode inactivation (i.e., formation of electro-polymerised firm). In addition to this, the relatively large over potential provides wide window for the interference which compromises the selectivity of the sensor system.[Citation10,Citation11] Improvements in the electrochemical assay have been achieved through the usage of electrode modifying materials such as mesoporous silica, multi walled carbon nanotubes (MWCNTs)–gold nanoparticles (GNPs) hybrid and chitosan assisted nitrogen-doped graphene sheets. Recently, Jiao et al.[Citation12] demonstrated the application of tannic acid (TA) functionalized N-doped graphene modified electrode for the sensitive determination of BPA. The achieved high sensitive was suggested to be the consequence of excellent synergy between the high surface area graphene and favourable interactions between TA and BPA molecules. Similarly, Wu et al.[Citation13] have demonstrated the amplification of BPA oxidation signal using hybrid of graphene-Fe2O3 nanostructures. Although the previously mentioned approaches are efficient, much improvement can be achieved using novel nanostructures which can not only provide higher surface area but also reduces the necessary redox potential required for the electrochemical oxidation of BPA. In this regard, utilization of bio-compatible template provides a simple and attractive approach toward production of new and novel nanostructure.[Citation14] In continuation of our previous work,[Citation15,Citation16] this study provides a new approach toward electro-chemical determination of BPA by using TA functionalized copper oxide (CuO) nanostructures. The utilized TA simultaneously works both as bio-compatible template and functionalizing agent. The fabricated nanostructures not only possessed excellent structural and morphological features but were also known to exhibit excellent electro-catalytic characteristic enabling highly sensitive determination of BPA in aqueous solution.
Experiments
Chemicals
All utilized chemicals were analytical grade. Copper chloride (CuCl2·2H2O), TA (C76H52O46), ammonia solution (NH3; 35%), and sodium hydroxide (NaOH) were obtained from Sigma Aldrich. Nafion® (C7HF13O5S.C2F4; 5%) solution in isopropanol (Merck) was utilized as ionic membrane. The phosphate buffer solution (PBS) 0.1 M (pH 6.5; Sigma Aldrich) was used as active electrolyte in this study.
Characterization
Field emission gun-scanning electron microscopy (FEG-SEM; Zeiss SIGMA), x-ray diffraction (XRD), and Fourier transform infrared (FTIR) spectroscopy were utilized for the morphological, compositional, and functionalization characteristics of TA functionalized CuO nanostructures. The electrochemical measurements were performed using Bipotentiostate model 760E, CH Instruments, USA.
Growth of functionalized copper oxide (CuO)
The CuO nanostructures were synthesised using simple hydrothermal growth method. In a typical experiment, 1.68 g of CuCl2.5H2O was vortex with 0.5 g of TA in a container for few minutes to obtain a homogenous mixture. The prepared mixture was then added 5 mL of 33% ammonia (NH3) solution and container was sealed with aluminium foil to avoid any solvent spills. The container was then subjected to heat treatment in a pre-heated electric oven at 95°C for 8 h. Herein, the utilized TA works simultaneously as an efficient template and functionalizing agent. The choice of using TA, is based on its capability to generate favorable interactions between the associated carbonyl groups and phenolic moieties of the BPA molecules. At the completion of growth, the as-synthesised nanostructures were separated from the solution and washed thoroughly with de-ionized water to remove any surface bound impurities.
Modification of the electrode
The electrode modification was achieved using similar strategies employed in our previous studies.[Citation14,Citation17,Citation18] Briefly, clean and thoroughly polished glassy carbon electrodes (GCEs) were treated in an ultrasonic bath of de-ionized water and ethanol to obtain a proper surface prior to modification. Simple drop casting methodology was used to modify the GCEs surface with a specific volume (3 µL) from the suspension (0.2 g/mL ethanol) of TA functionalized CuO nanostructures. The modified layer was then dried under N2 stream followed by casting of Nafion® (Naf; 2 µL) membrane to ensure complete adherence of the modified layer. The devised electrode is denoted as TA-CuO/GCE/Naf throughout the manuscript.
Electrochemical detection of BPA
The electrochemical detection of BPA was achieved using differential pulse voltammetry (DPV) as a primary mode of quantification. The experimental system consisted of a three electrode system with Ag/AgCl as reference and Pt wire as counter electrode. The devised electrode (TA-CuO/GCE/Naf) was further used as working electrode. The measurements were performed at room temperature and pressure within the potential window of 0.15–0.55 V and sensitivity of 1×10−6 (A/V). The PBS was taken as the active electrolytic solution with concentration of 0.1 M and pH 6.5.
Results and discussion
Structural and compositional characteristics of synthesised CuO nanostructures
The as-synthesised CuO nanostructures were characterized for their morphological structures using FEG-SEM. The captured images are presented in . It can be seen that the fabricated nanostructures possesses structural morphology similar to globules. The formed globules can be seen to have adopted shape of large sphere within which high density of such nanoglobules (NGBs) can be viewed. The average size of these NGBs was estimated to be in range of 25–50 nm. To further confirm the functionality over the surface of CuO nanostructures, FTIR spectra were recorded for TA-functionalized CuO nanostructures and was evaluated against FTIR spectra of pure TA ().
Figure 2. FTIR spectrum of A: Pure tannic acid and B: tannic acid functionalized CuO nanostructures.
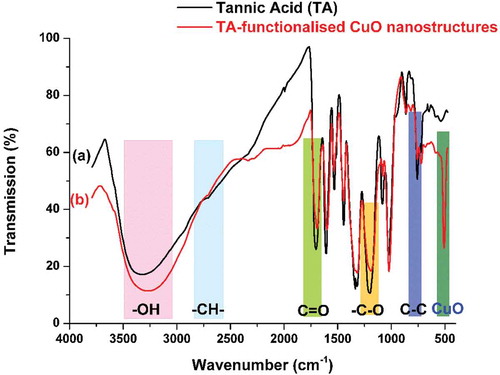
TA contains multiple hydroxyl groups and thus, the steric arrangement would allow some of these moieties to interact with the surface of CuO while others may be available at the nanomaterial/surface interface for interaction with other nuclei or act as a functional group. It is, therefore, expected to observe a shift in the frequencies of the corresponded functional groups relative to pure TA. The said scenario is noted in the recorded FTIR spectra’s shown in . The significant shift is noted for major groups like -OH and C=O along with minor variations in the associated groups like (-CH-), (-C-O) and (-C-C). In addition to this, a strong peak residing near 500 cm−1 attributed to Cu-O vibration frequency is noted for TA-functionalized CuO NGBs. The observed variations are in complete coherence with many TA related studies.[Citation19–Citation21] The experimental observation clearly indicates the successful functionalization of CuO with TA. The XRD analysis was carried to ensure the purity of as-synthesised CuO nanostructures. The recorded patterns () consisted of peaks indexed to (110), (111), (002), (212), (113), (220), (311), (004), and (222) planes of pure monoclinic CuO as referenced against the ICCD No: 45-0937.[Citation22] The absence of any peaks other than CuO signifies the purity of as-synthesised product.
Electrochemical behavior of modified GCE
The electrochemical response of the modified electrode was assessed against 0.1 µM of BPA using cyclic voltammetry (CV) as the basic mode of operation. The electrode behavior was evaluated in comparison to bare GCE both in the absence and presence of BPA with 0.1 M PBS (pH 6.5) taken as active electrolyte solution. displays the recorded CV curves where relatively significant response of TA-CuO/GCE/Naf can be seen for BPA in comparison to bare GCE. The observed high current intensity and negative shift in the measured oxidation potential indicates the intrinsic sensitivity of the modified electrode. The recorded sensitivity in this case may be attributed to the favourable interaction prevailing between the oppositely charged moieties of TA and BPA molecule. Such interactions, synergized with the excellent surface features of CuO NGBs can enable faster and greater adsorption of BPA which in turn allow sensitive signal generation and assist in achieving lower detection limit.
Figure 4. The electrochemical behavior of (a-b) bare (c-d) TA-CuO/GCE/Naf in the absence and presence of 0.1 µM BPA.
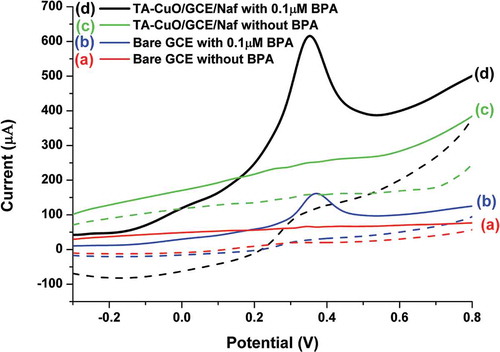
To tune the observed response of the modified electrode (TA-CuO/CGE/Naf) in order to achieve maximum current response, some crucial parameter like scan rate and pH of the buffer solution were optimized. shows the variation of current response for TA-CuO/CGE/Naf in 0.1 µM BPA with 0.1 M PBS buffer (pH 6.5). Increment in the observed response with noted linearity between the current and square root scan rate (inset figure) signifies the dominance of diffusion controlled process in the electro-oxidation reaction of interest. shows the variation of current response against the pH of the buffer solution in range of 3.5 to 8.5. As seen, an initial increment was achieved up to pH 6.5, where after decline was observed at higher pH values. Henceforth, pH 6.5 was considered as the optimum pH for determination of BPA.
Analytical assessment of developed sensor
The analytical assessment of the developed sensor was carried by measuring the current response of TA-CuO/CGE/Naf against BPA in concentration range of 0.1 to 5.5 µM. The DPV was used for the quantification due to its advantage of low-background current. The recorded DPV waves are shown in with inset graph reflecting the plotted calibration and working linearity (R2 of 0.998) estimated using linear fit analysis. The limit of detection (LOD) and quantification (LOQ) of the developed sensor was estimated to be 0.01 and 0.15 µM, respectively.
Selectivity and stability of the developed sensor
The sensor system was evaluated for its selectivity in the presence of common co-existing molecules, such as phenol, hydroquinone, and 4-nitrophenol, with concentration 10-fold higher than of BPA (0.1 µM). The observed variation in current against these interferents is shown as normalized bar-graph in . The negligible variation in the noted current indicates the selectivity of the sensor system. The higher selectivity in this case may be attributed to the measured low-over potential which restrains the utilised catalyst (TA-CuO NGBs) to oxidize other molecules. The developed sensor system also demonstrated excellent stability when stored in refrigerator within electrolyte solution at 4°C for 8 weeks. The observed small percentage (<1.0%) of relative standard deviation (RSD) in the measured current responses () suggests the inherent stability of the developed sensor system.
Conclusion
The present study describes the application of CuO nanostructures for the potential electrochemical sensing of BPA. The utilized CuO nanostructures were synthesised using TA which acted simultaneously both as an efficient bio-compatible template and functionalizing agent. The usage of TA enabled formation of unique globular shaped nanostructures, whereas the excellent synergy between the high surface area of as-synthesized CuO NBGs and favorable interactions between TA and BPA molecules enabled production of highly sensitive electrochemical sensor.
Acknowledgments
The authors would like to thank the National Centre of Excellence in Analytical Chemistry, University of Sindh, Jamshoro, Pakistan for providing the facilities during this research. The authors acknowledge the contribution of IAC, University of Bristol and Dr. Keith Richard Hallam for his assistance in FEG-SEM analysis.
Funding
The authors acknowledge the Higher Education Commission, Islamabad, Pakistan for funding this research under IRSIP program.
Additional information
Funding
References
- Martínez Urreaga, J.; González-Sánchez, C.; Martínez-Aguirre, A.; Fonseca-Valero, C.; Acosta, J.; de la Orden, M.U. Sustainable Eco-Composites Obtained from Agricultural and Urban Waste Plastic Blends and Residual Cellulose Fibers. Journal of Cleaner Production 2015, 108(Part A), 377–384.
- Hopewell, J.; Dvorak, R.; Kosior, E. Plastics recycling: Challenges and opportunities. Philosophical Transactions of the Royal Society B: Biological Sciences 2009; 364(1526), 2115–2126.
- Vandenberg, L.N.; Maffini, M.V.; Sonnenschein, C.; Rubin, B.S.; Soto, A.M. Bisphenol-A and the Great Divide: A Review of Controversies in the Field of Endocrine Disruption. Endocrine Reviews 2009, 30(1), 75–95.
- Hardy, M.L.; Banasik, M.; Stedeford, T. Toxicology and Human Health Assessment of Decabromodiphenyl Ether. Critical Reviews in Toxicology 2009, 39(Suppl3), 1–44.
- Portaccio, M.; Di Tuoro, D.; Arduini, F.; Lepore, M.; Mita, D.G.; Diano, N.; Mita, L.; Moscone, D. A Thionine-Modified Carbon Paste Amperometric Biosensor for Catechol and Bisphenol a Determination. Biosensors and Bioelectronics 2010, 25(9), 2003–2008.
- Khedr, A. Optimized Extraction Method for LC–MS Determination of Bisphenol A, Melamine and Di(2-Ethylhexyl) Phthalate in Selected Soft Drinks, Syringes, and Milk Powder. Journal of Chromatography B 2013, 930, 98–103.
- Kosarac, I.; Kubwabo, C.; Lalonde, K.; Foster, W. A Novel Method for the Quantitative Determination of Free and Conjugated Bisphenol A in Human Maternal and Umbilical Cord Blood Serum Using a Two-Step Solid Phase Extraction and Gas Chromatography/Tandem Mass Spectrometry. Journal of Chromatography B 2012, 898, 90–94.
- Yin, H.; Zhou, Y.; Ai, S.; Han, R.; Tang, T.; Zhu, L. Electrochemical Behavior of Bisphenol A at Glassy Carbon Electrode Modified with Gold Nanoparticles, Silk Fibroin, and PAMAM Dendrimers. Microchimica Acta 2010, 170(1), 99–105.
- Yu, C.; Gou, L.; Zhou, X.; Bao, N.; Gu, H. Chitosan–Fe3O4 Nanocomposite Based Electrochemical Sensors for the Determination of Bisphenol A. Electrochimica Acta 2011, 56(25), 9056–9063.
- Ngundi, M.M.; Sadik, O.A.; Yamaguchi, T.; Suye, S.-i. First Comparative Reaction Mechanisms of β-Estradiol and Selected Environmental Hormones in a Redox Environment. Electrochemistry Communications 2003, 5(1), 61–67.
- Pan, B.; Lin, D.; Mashayekhi, H.; Xing, B. Adsorption and Hysteresis of Bisphenol A and 17α-Ethinyl Estradiol on Carbon Nanomaterials. Environmental Science & Technology 2008, 42(15), 5480–5485.
- Jiao, S.; Jin, J.; Wang, L. Tannic Acid Functionalized N-Doped Graphene Modified Glassy Carbon Electrode for the Determination of Bisphenol A in Food Package. Talanta 2014, 122, 140–144.
- Wu, C.; Cheng, Q.; Li, L.; Chen, J.; Wu, K. Synergetic Signal Amplification of Graphene-Fe2O3 Hybrid and Hexadecyltrimethylammonium Bromide as an Ultrasensitive Detection Platform for Bisphenol A. Electrochimica Acta 2014, 115, 434–439.
- Razium Ali Soomro, Z.H.I.; Sirajuddin; Sherazi, S.T.H.; Abro, M.I.; Willander, M.; Mahesar, S.A.; Kalwar, N.H. Glycine-Assisted Preparation of Co3O4 Nanoflakes with Enhanced Performance for Non-Enzymatic Glucose Sensing. Material Express 2015, 5, 437–444.
- Soomro, R.A.; Hallam, K.R.; Ibupoto, Z.H.; Tahira, A.; Jawaid, S.; Hussain Sherazi, S.T.; Sirajjuddin; Willander, M. A Highly Selective and Sensitive Electrochemical Determination of Melamine Based on Succinic Acid Functionalized Copper Oxide Nanostructures. RSC Advances 2015, 5(127), 105090–105097.
- Soomro, R.A.; Hallam, K.R.; Ibupoto, Z.H.; Tahira, A.; Sherazi, S.T.H.; Sirajjuddin; Memon, S.S.; Willander, M. Amino Acid Assisted Growth of CuO Nanostructures and Their Potential Application in Electrochemical Sensing of Organophosphate Pesticide. Electrochimica Acta 2016, 190, 972–979.
- Ibupoto, Z.H.; Nafady, A.; Soomro, R.A.; Sirajuddin; Hussain Sherazi, S.T.; Abro, M.I.; Willander, M. Glycine-Assisted Synthesis of NiO Hollow Cage-Like Nanostructures for Sensitive Non-Enzymatic Glucose Sensing. RSC Advances 2015, 5(24), 18773–18781.
- Soomro, R.; Ibupoto, Z.; Sirajuddin; Abro, M.; Willander, M. Controlled Synthesis and Electrochemical Application of Skein-Shaped NiO Nanostructures. Journal of Solid State Electrochemistry 2015, 19(3), 913–922.
- Albu, M.G.; Ghica, M.V.; Giurginca, M.; Trandafir, V.; Popa, L.; Cotrut, C. Spectral Characteristics and Antioxidant Properties of Tannic Acid Immobilized on Collagen Drug-Delivery Systems. Revista de Chimie–Bucharest 2009, 60(7), 666–672.
- Pantoja-Castro, M.A.; González-Rodríguez, H. Study by Infrared Spectroscopy and Thermogravimetric Analysis of Tannins and Tannic Acid. Revista latinoamericana de Química 2011, 39(3), 107–112.
- Nedi, Z.P. A Study of the IR Spectra of the Copigments of Malvin Chloride with Organic Acids. Journal of the Serbian Chemical Society 2001, 66(7), 451–462.
- Duan, Y.; Liu, X.; Han, L.; Asahina, S.; Xu, D.; Cao, Y.; Yao, Y.; Che, S. Optically Active Chiral CuO “Nanoflowers.” Journal of the American Chemical Society 2014, 136(20), 7193–7196.