ABSTRACT
The effects of number of homogenization passes, pH, and NaCl concentration on the formation and stability of oil-in-water emulsions comprising a mixture of a biosurfactant (Quillaja bark saponin) and a globular protein (β-lactoglobulin) were investigated. The emulsions were characterized as to visual appearance, droplet size, droplet surface charge, and rheology. The emulsions obtained by different conditions (4, 6, or 8 passes; pH 7, 8, or 9; and 0, 100, or 200 mmol L−1 of NaCl) were polydisperse, presented relatively small average droplet sizes (z-average < 323 nm) as well as negative droplet charge (between –20 and –79.6 mV) in all evaluated conditions. Regardless of the number of homogenization passes, the emulsions exhibited low apparent viscosity and pseudoplastic behavior with small yield stress. Viscoelastic behavior was also observed, thus the emulsions were characterized as weak gels. Four homogenization passes were enough to obtain small droplets in the evaluated conditions. Droplet size was not significantly affected by NaCl concentration and pH (p > 0.05). On the other hand, the absolute ζ-potential values significantly decreased and increased upon increased NaCl content and pH, respectively. Regardless of the tested conditions, all emulsions had good stability against phase separation and droplet aggregation, since no significant changes in average droplet size were observed throughout storage (p > 0.05). In the presence of NaCl, in which droplet charge significantly decreased, emulsion was also stable. Thus, we can conclude that electrostatic repulsion as well as steric repulsion was responsible for stabilization.
Introduction
Emulsions are thermodynamically unstable systems consisting of two immiscible liquid phases, wherein one of these is dispersed within the other as small, spherical droplets of diameters typically ranging from 0.1 to 100.0 μm). Despite of the intrinsic thermodynamic instability of emulsions, the presence of emulsifying and/or stabilizing agents can provide these systems with kinetic stability to different extents, at least for a certain period of time.[Citation1] The emulsifying agent promotes emulsion formation and short-term stabilization by interfacial action. Two classes of emulsifying agents are commonly used in food formulations: low molecular weight surfactants and macromolecular emulsifiers (usually proteins),[Citation2] which can be used separately or in synergistic combinations. In the last years, most of the studies on emulsion formation and stabilization by mixtures of proteins and low molecular weight surfactants have dealt with synthetic surfactants, particularly those derived from petroleum.[Citation3] However, because of the growing demand from consumers for healthier, environmentally friendly products, the replacement of these surfactants by those of biological origin (e.g., biosurfactants) has been arousing increasing interest.[Citation4–Citation6]
Saponins are biosurfactants consisting of molecules that are formed by a triterpene or steroid aglycone moiety attached by glycoside bonds into a linear or branched sugar moiety, thus possessing an amphiphilic character.[Citation5,Citation7–Citation9] Among the various industrially available saponin sources, the Quillaja saponaria Molina tree (native to Chile and Peru) has been extensively exploited and pointed out as a promising source of saponins.[Citation10] Thus, Quillaja bark saponin (QBS) has attracted great attention from both academia and industry. Indeed, one may find recent studies addressing the interfacial, foaming, and emulsifying properties of QBS mixtures with different proteins.[Citation5,Citation10,Citation11]
In particular, Piotrowski et al.[Citation11] studied the interfacial and emulsifying properties of QBS and β-lactoglobulin (β-lg) mixtures at different molar ratios. At small biosurfactant/protein ratios, these authors observed a synergistic effect in the biosurfactant adsorption kinetics at the water–oil interface due to a protein-biosurfactant complexation, which facilitates the dissociation of the protein dimer and loosens its previously rigid structure, facilitating further unfolding upon reaching the interface and increasing the adsorption rate. This effect was, though, much less noticeable in foamability and emulsion formation/stability.[Citation11] This study was carried out with minimum β-lg concentration (10−7 mmol L−1) and dispersed phase volume fraction (0.2 wt% of oil), which do not correspond to real situations found in foods. Furthermore, not much is known about the effects of homogenization and aqueous phase composition (pH and ionic strength) on the formation and stability of oil-in-water emulsions (O/W) containing QBS and β-lg.
Given this context, the present study intended to assess the effects of number of homogenization passes, pH, and salt concentration on the formation and kinetic stability of model O/W emulsions formulated with QBS and β-lg mixtures. The characterization of these emulsions was performed through visual analysis and the determinations of droplet size, droplet surface charge, and rheology in order to explore the role that these variables play in emulsion formation as well as possible stabilization mechanisms.
Materials and methods
Materials
β-lactoglobulin was kindly provided by Davisco Foods International Inc. (La Sueur, MN, USA). The protein content was reported to be 90 wt%. QBS, a mixture of triterpene-glycosides extracted from the bark of the Quillaja saponaria Molina tree, was purchased from Sigma-Aldrich Co. (S4521 Lot # SLBD7908V; St. Louis, MO, USA). The surfactant (active ingredient) content of QBS was reported to be 32 wt%. The average molecular weight of Quillaja saponin has been reported to be 1650 g mol−Citation1. [Citation10,Citation12–Citation14] The concentrations reported in this study were all based upon the effective amount of the compound of interest, as disclosed by the suppliers. Commercial sunflower oil (Liza, Cargill Foods, SP, Brazil) was purchased from a local market. Analytical grade sodium chloride, tris(hydroxymethyl)aminomethane (tris), sodium azide, hydrochloric acid, and Sudan III were purchased from Sigma-Aldrich Co. (St. Louis, MO, USA). These materials were used as received, without further purification. Double distilled and deionized water (electrical resistivity equal to 18.2 MΩ·cm; Millipore Inc, Milli-Q, USA) was used in all experiments.
Emulsion preparation
O/W emulsions were prepared by homogenizing 10 wt% oil phase (sunflower oil colored with a pinch of Sudan III dye) with 90 wt% aqueous phase. The latter consisted of a tris buffer solution (5 mmol L−1) and also containing QBS (0.6 mmol L−1), β-lg (0.6 mmol L−1), and sodium azide (NaN3; 0.02 wt%). NaN3 was added to inhibit microbial growth throughout storage. In addition, the pH (7, 8, or 9) and NaCl concentration (0, 100, or 200 mmol L−1) of the aqueous phase were varied by adjusting the tris:HCl ratio.[Citation15] Pre-emulsions were prepared at room temperature by mixing the oil and aqueous phases for 3 min at 7500 rpm using a high-speed blender (Omni Macro ES Digital Programmable Homogenizer, Kennesaw, USA) equipped with a 20-mm working head. Subsequently, the coarse emulsions (25 mL) were submitted to 4, 6, or 8 passes within a high-pressure homogenizer (Emulsiflex-C5, Avestin, Ottawa, Canada) operating at approximately 10,000 psi at room temperature.
Emulsion characterization
Droplet characterization
The ζ-potential of the oil droplets was determined using an automated particle electrophoresis instrument (Zetasizer Nano ZS, Malvern Instrument Ltd., Worcestershire, UK), which measures the direction and velocity of droplet movement submitted to a controlled electric field (electrophoretic mobility). Droplet size was determined using the same instrument, but by dynamic light scattering technique. Z-average diameter (assigned to droplet size) and polydispersity index (PDI) were obtained by Cumulants analysis of correlation function using the initial portion of the data (up to 2000 μs).
Prior to each measurement, samples were diluted (at least 1:300) in a 5 mmol L−1 tris buffer with the same pH and NaCl concentration of the emulsion to avoid multiple scattering effects.[Citation16] The buffers were previously filtered using a 0.45 µm cellulose acetate membrane (Millipore). All measurements were accomplished right after homogenization process as well as after 7 days of storage at 25°C. For measurements after the storage period, samples were carefully withdrawn from the top, middle, and bottom parts of the flasks (which had been kept vertically), in an effort to avoid turbulence. All measurements were carried out at 25°C. All experiments were performed using at least two independent samples of each emulsion and at least three readings per sample. The results were reported as averages and standard deviations that were calculated from these replicate measurements.
Rheological properties
Rheological analyses of emulsions were performed using a modular advanced rheometer system (Haake Mars II, Thermo Electron Corp., Karlsruhe, Germany) equipped with a thermostatic bath (Phoenix 2C30P, Thermo Electron Corp., Germany) and a stainless steel cone-plate measuring unit (1°; diameter of 60 mm; gap of 52 µm). The measurements were performed at constant temperature (25.0 ± 0.1°C). Samples were carefully poured onto the surface of the plate and then the cone was lowered until it reached a gap of 52 μm. After loading, the sample was upheld for 5 min to allow sample relaxation and temperature equilibration prior to each measurement. Fresh samples were used in each assay to avoid droplet rupture and coalescence upon shearing. All rheological tests were done in duplicate.
Steady-shear flow measurements were carried out to assess the flow behavior of the emulsions. Flow curves were acquired by varying the shear rate from 10 to 300 s−1 during 2 min (up, down, and up flow curves). Shear stress and apparent viscosity were recorded as a function of shear rate. Flow behavior was described by fitting the Herschel-Bulkley model (Eq. 1) to the experimental data (second up flow curve):
where is the shear stress (Pa),
is the yield stress (Pa),
is the consistency coefficient (Pa.sn),
is the shear rate (s−1), and
is the flow behavior index (dimensionless). For dynamic viscoelastic measurements, the linear viscoelastic range of the emulsions was determined with a strain sweep (0.1 to 100 %) at a constant frequency (1 Hz). Then, frequency was swept from 0.1 to 3.16 Hz. Frequencies greater than 3.16 Hz undesirably led to sample wall slip (probably due to phase separation at higher frequencies). The constant strain amplitude was controlled in 0.3%, value that was within the linear viscoelastic range. The storage modulus (G’), loss modulus (G”), and loss tangent (tan δ = G”/G’) values were continuously determined as functions of frequency (ω).
Emulsion kinetic stability
The temporal stability of the emulsions against phase separation was visually assessed. Following the homogenization step, 5 mL of fresh emulsions were poured in 12-mm-inner diameter, 125-mm-high graduated glass test tubes that were tightly sealed with a plastic film and then stored at 25°C. Phase separation was visually determined by monitoring the thickness of the cream and serum phases (a turbid or transparent bottom layer) after storage. The extent of phase separation was quantitatively characterized by a phase separation index (PSI), which was calculated according to Eq. (2):
where and
represent the volume of clear serum formed at the bottom of the tubes at the end of the storage period and the initial emulsion volume, respectively.
Sunflower oil was dyed with a small amount of oil-soluble Sudan III dye to facilitate monitoring of creaming. Samples (3 mL) were also stored in capped glass flasks for further droplet size measurements after 7 days. Pictures of these emulsion samples were taken after 7 days using a digital camera (Cyber-shot DSC-W610 14.1 MP, Sony Corp, Tokyo, Japan) in order to record their aspects.
Statistical analyses
All of the measurements were carried out at least with two repetitions and were reported as average values and standard deviations. Data were evaluated by analysis of variance (ANOVA) using the SAS® software—version 9.0 (SAS Institute Inc., NC, USA), licensed for use by the Federal University of Viçosa. Significant differences among average values were determined by Tukey’s test and paired t-test when these were evaluated throughout time. The quality of adjustment of the Herschel-Bulkley model was given in terms of the coefficient of determination (RCitation2) and absolute mean percentage error (AMPE), as described by Eq. (3):
where is the ith experimental score,
is the ith predicted score, and
is the number of score pairs. The higher the RCitation2 values and the lower the AMPE value, the better the fitting. The level of significance (p) adopted was 0.05.
Results and discussion
Influence of the number of homogenization passes
The influence of the number of homogenization passes (4, 6, or 8 passes at 10,000 psi) on the formation and stability of O/W emulsion composed of 10 wt% sunflower oil, at pH 7 and without salt addition was evaluated. During high-pressure homogenization, a high energy input to the system is necessary to promote the successive droplet rupture and size reduction as well as to more evenly distribute emulsifier molecules on the surface of the newly formed drops, thereby reducing the Gibbs free energy of surface and, thus, enhancing the kinetic stability of the system.[Citation1] In general, it is expected that the average droplet size decreases and the uniformity of droplet size distribution increases (i.e., polidispersity decreases) with increasing number of homogenization passes. This is because each pass brings an additional amount of mechanical energy to the liquid mixture. However, as observed in , increasing the number of homogenization passes did not significantly reduce (p > 0.05) the average droplet size and the polydispersity of the studied emulsions. The production of small droplets with uniform size distribution depends on the completion of the size reduction, which requires more energy and higher emulsifier concentrations. Therefore, it is likely that the emulsifier concentration has not been enough to cover the interfacial area created in every single homogenization pass. Consequently, further droplet size reduction has not been possible from the fourth pass on. Besides, the relatively high PDI values indicate a polydisperse emulsions with a wide range of droplet sizes.
Table 1. Average droplet size (z-average), polydispersity index (PDI), and droplet surface charge (ζ-potential) for emulsions homogenized at different numbers of passes and stabilized by Quillaja bark saponin and β-lactoglobulin.
The effect of the number of homogenization passes on droplet ζ-potential is also presented in . Highly negative electrical droplet charges were observed for all emulsions, irrespective of the number of homogenization passes. This could be explained by the dissociation of –COOH groups of β-lg and QBS, provided that pH 7 is higher than the isoelectric point (pI) of the protein (pI = 5.2)[Citation17] and than the pKa of the glucuronic acid present in the glycone part of the saponin molecule (pKa = 3.18).[Citation12] As the number of homogenization passes was increased, a significant variation (p < 0.05) on droplet ζ-potential was observed. These changes may be attributed to the shear effects on the protein molecules, with concomitant increase of the system temperature during homogenization. The fluid mechanical shear might induce changes in the tertiary and/or secondary protein structures, which further unfold the protein and expose its hydrophobic groups, thus triggering changes in the net electrical charge balance around the droplets as well as favoring the formation of aggregates. For instance, Kuhn and Cunha[Citation18] studied the effect of high-pressure homogenization (20–100 MPa) and of the number homogenization passes (1–7) on O/W emulsions containing 30 vol% of oil and 3 wt% of whey protein isolate at pH 7. These authors observed the formation of aggregates after one homogenization pass at 80 MPa and that this was intensified by the increased temperatures after 4 and 5 passes, despite the fact that the denaturation temperature of whey protein fractions has not been reached. Therefore, it is believed that a similar phenomenon occurred in the present study, since the β-lg corresponds to the major protein fraction of whey protein and the homogenization pressure (≈70 MPa) used was close to that used by Kuhn and Cunha.[Citation18]
The flow curves of all emulsions indicated a shear-thinning behavior. This behavior is typical of associative weak interactions[Citation19] and has been reported for different protein-based emulsions.[Citation20–Citation25] This physical behavior can be attributed to different microscopic factors, such as changes in spatial distribution of disperse particles due to shear forces, alignment of non-spherical droplets, removal of solvent molecules bonded to droplets, and deformation and rupture of flakes.[Citation26] The Herschel-Bulkley model was well fitted to the data (), leading to RCitation2 > 97% and AMPE < 11% for all studied conditions. The shear rate equal to 100 s−1 was chosen for calculation and analysis of apparent viscosity ( because this value is typical of shear situations involving food, such as flow through pipes, stirring, and chewing.[Citation1,Citation27]
Table 2. Rheological parameters obtained from the Herschel-Bulkley model and apparent viscosity at 100 s−1 () for emulsions homogenized at different numbers of passes and stabilized by Quillaja bark saponin and β-lactoglobulin.
The pseudoplasticity of the emulsions significantly decreased with increased number of homogenization passes, as indicated by the increased (p < 0.05) flow behavior index (n). The consistency index (K) also decreased by increasing the number of passes through the homogenizer (p < 0.05), suggesting an overall increase of emulsion fluidity. These results are analogue to those found by Kuhn and Cunha[Citation18] for emulsions containing whey protein isolate homogenized at high pressure (80 and 100 MPa) as well as by Kuhn et al.[Citation24] for the top-phase of emulsions containing fixed of flaxseed oil (30 vol%) and flaxseed protein isolate alone (0.14, 0.35, or 0.7% w/v) at pH 7. However, no significant changes were observed on upon increased homogenization passes (p > 0.05). In addition, small yield stress values (τ0 > 0) were observed, which denote an important parameter in situations involving low deformations, including droplet creaming during storage, since the presence of yield stress implies a certain resistance to flow at low deformations. Yield stress values decreased with an increase in the number of passes through the homogenizer (p < 0.05), indicating that more fragile and less structured emulsions were formed with increased homogenization times. This may be harmful to the kinetic stability because droplets ought to exceed a lower yield stress to initiate flow. Moreover, by comparing the up and down flow curves (data not shown), little hysteresis was observed, suggesting that the emulsion structure was broken during the homogenization process.
The dynamic rheological behavior of the emulsions was also evaluated. The mechanical spectra () were obtained from frequency sweep to evaluate the emulsion viscoelastic behavior, as this physical property is sensible not only to the chemical composition and physical microstructure of the material, but also to the mechanical conditions to which it is submitted.[Citation27] Overall, the storage modulus (G’) ranged in magnitude from 1.5 to 9.5 times greater than the loss modulus (G”) values, indicating a predominantly elastic behavior within the entire analyzed frequency range. The relatively small values of these viscoelastic moduli (<4 Pa), along with the previously mentioned shear-thinning behavior of the samples, suggested a weak three-dimensional structure. An increase in the number of passes decreased the viscoelastic moduli but increased the dependence of G’ and G” on the frequency. Therefore, emulsions submitted to 6 and 8 homogenization passes showed lower viscoelastic moduli than that obtained with 4 passes. This is in agreement with the lower consistency index and yield stress exhibited by emulsions submitted to 6 and 8 homogenization passes (), supporting the previous inference concerning its weaker three-dimensional structure. Therefore, less structured emulsions were formed by greater number of passes and, consequently, longer homogenization times. The low frequency dependence of both moduli, the absence of crossing over (a frequency value for which G’ = G”), and the G’/G” ratio < 10 throughout the tested frequency range allow characterizing the emulsions as weak gels (also known as physical gel),[Citation27] which can be seen as a “structured liquid.”[Citation28] Santos et al.,[Citation29] who studied potato protein-based emulsions stabilized by guar gum, observed that emulsions containing 40 wt% of sunflower oil, 2 wt% of potato protein, and 0.1, 0.2, or 0.3 wt% of guar gum at pH 8 also showed a weak gel behavior. According to Steffe[Citation27] and Brummer,[Citation30] kinetically stable emulsions are characterized by mechanical spectra with G’ > G” and moduli curves almost parallel all along a broad frequency range.
Figure 1. Influence of the number of homogenization passes—4 (●), 6 (▼), or 8 (■) passes—on the elastic modulus (G’, closed symbol) and the viscous modulus (G”, open symbol) of the emulsions stabilized by QBS and β-lg at pH 7.
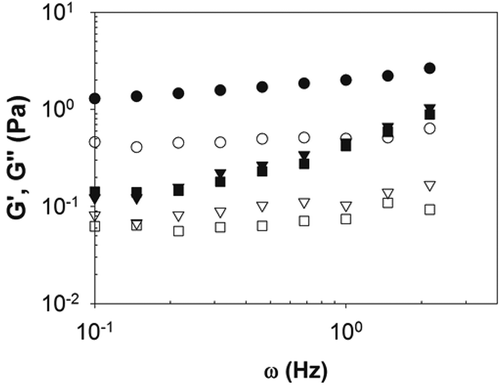
Regardless of the number of homogenization passes, all emulsions showed no clue of phase separation (PSI = 0 after 7 days storage at 25°C; –F1). The presence of a foam layer has been observed throughout the homogenization process. Indeed, it is unavoidable because both QBS and β-lg are also foaming agents and the system is kept in contact with air.[Citation11,Citation31,Citation32] Emulsion foaming during homogenization has also been observed in other studies, including that of Piotrowski et al.,[Citation11] who studied the effect of β-lg (10−7 mol L−1) on the kinetics of QBS adsorption (5×10−7 – 10−3 mol L−1) on the air–water and oil–water interfaces and compared the results with the foaming and emulsifying properties of the β-lg and QBS mixtures (phosphate buffer pH 7, ionic strength 0.1 mol L−1). A similar trend was reported by Yang and McClements[Citation32] when studying the influences of phase composition (vitamin E/medium chain triglyceride ratio, ranging from 0 to 100 wt% of vitamin E), aqueous phase composition (glycerol/water ratio, ranging from 0 to 50 wt% glycerol), and surfactant (either 1 wt% of QBS or Tween 80 at 10 mM sodium phosphate buffer, pH 7) on the size of droplets produced by high-pressure homogenization, aiming to identify the optimum condition for the development of all-natural emulsion-based delivery systems for encapsulating vitamin E.
Figure 2. Visual appearance of 10 wt% sunflower oil-in-water emulsions stabilized by Quillaja bark saponin (QBS) and β-lactoglobulin (β-lg) after 7 days of storage at 25°C. F1: pH 7 and A: 4; B: 6; and C: 8 homogenization passes; F2: 4 homogenization passes at 10,000 psi and pH A: 7; B: 8; and C: 9; F3: pH 7 and NaCl concentrations of A: 0, B: 100, and C: 200 mmol L-1. Black arrow denotes foam formation, observed during emulsion preparation.
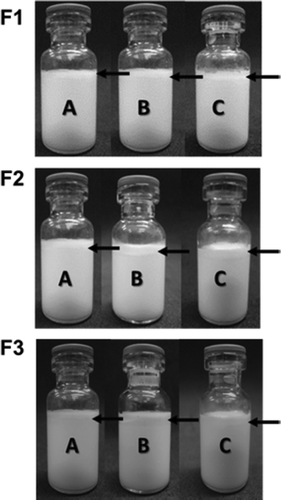
Here, regardless of the number of cycles, a vertical color gradient has also been observed, being the color slightly more intense on the top. This gradient has resulted from the different creaming speeds of emulsion droplets (larger droplets have moved more rapidly to the top) since the emulsions have not shown droplet size uniformity, as corroborated by the higher PDI values (). Thus, there has been a concentration of large red droplets at the top, which resulted in a more intense staining.
The physical stability of the emulsions was also evaluated in terms of droplet size and charge changes after storage. No significant differences were found (p > 0.05) for the average droplet diameter, PDI, and ζ-potential after 7 days, suggesting that the emulsions remained stable against droplet aggregation (). Electrostatic repulsion between emulsion droplets is a possible mechanism involved in the stabilization of these systems. It was remarkably intense in the emulsions studied here due to the high ζ-potential values, explaining our findings.
Influence of pH
Since the number of homogenization passes had no significant effect on droplet size (p > 0.05) and all emulsions were stable against droplet aggregation and phase separation (–F1 and ), 4 homogenization passes were used in the subsequent emulsion production procedures in order to lessen heating and energy costs. Hence, the influence of pH (7, 8, and 9) on emulsion average droplet size, ζ-potential, and physical stability in the absence of salt was examined.
Table 3. Average droplet size (z-average), polydispersity index (PDI), and ζ-potential for emulsions stabilized by Quillaja bark saponin and β-lactoglobulin at different pH values.
Table 4. Average droplet size (z-average), polydispersity index (PDI), and ζ-potential for emulsions stabilized by Quillaja bark saponin and β-lactoglobulin at different NaCl concentrations.
The droplet electrical charge was negative for all of the studied pH values (). This is because both QBS and β-lg are in the anionic form, i.e., the pH values are higher than the protein pI of the protein[Citation17] and the pKa of the glucuronic acid group[Citation12] present in the surfactant molecule. The ζ-potential was significantly influenced by the pH of the continuous phase (p < 0.05; ), being its magnitude slightly larger at pH 8 and 9 due to the higher degree of deprotonation of the carboxylic groups in the protein and the surfactant in such medium condition, making droplet surfaces more negatively charged. However, throughout the entire pH range, the ζ-potential values were higher (in magnitude) than 60 mV, indicating strong electrostatic repulsion between droplets and ensuring great electrostatic stability.[Citation33] The dynamic light scattering measurements indicated that the emulsions were polydisperse and had relatively small droplets (z-average < 323 nm; ). Differently from droplet charge, droplet size, and PDI were not influenced by the pH of the continuous phase (p > 0.05). Different result was observed by Romero, Cordobés and Guerrero,[Citation34] who assessed the effect of pH (2–11.5) on droplet size distribution of 75 wt% sunflower O/W emulsions containing 5 wt% of crayfish flour. These authors found larger droplets and wider droplet size distribution as pH approached 3.5 (average pI of these flour proteins). This outcome was attributed to the reduced electrostatic repulsion.
Emulsions formulated at different pH values were also stable against phase separation (PSI = 0) and showed a vertical color gradient due to the different droplet creaming rates (–). Droplet charge significantly changed (p < 0.05) to reduced absolute values during time at pH 7 and 8 (), which may be associated with possible rearrangements of emulsifiers’ layer adsorbed to droplet surface. However, despite the ζ-potential changes, the emulsions exhibited high surface charge, which provided them with high electrostatic stability because of the strong electrostatic repulsion between droplets. This is consistent with the dynamic light scattering results after storage, given that no significant changes were observed in the average droplet diameter and PDI of the emulsions (p > 0.05; ), suggesting the absence of net droplet aggregation. The stability of the emulsions prepared at different the pH values may be attributed to the high surface charge of the droplets, besides the fact that these were small and led to improved emulsion stability over time.
Influence of ionic strength
The effects of NaCl concentration (0, 100, or 200 mmol L−1) on the emulsion average droplet size, ζ-potential, and physical stability were also investigated. The ζ-potential of a particle depends on the chemical species on its surface and around it, as well as on pH and ionic strength of the surrounding medium.[Citation1] As expected, all emulsions showed negative net surface charge because the pH of the continuous phase was higher than the pI of β-lg and the pKa of the acidic group of QBS (). Increased NaCl concentrations resulted in significantly decreased ζ-potential magnitudes (p < 0.05). Such decrease in droplet surface charge may be explained by the binding of Na+ cations to anionic groups present in the molecules adsorbed on the droplet surface as well as by the electrostatic screening effect played by the added counter ions.[Citation1,Citation35] Thus, lower stability might be expected for NaCl-added emulsions due to their reduced ζ-potential values, which in turn lead to weaker electrostatic repulsion forces. The emulsions formulated with different NaCl concentrations were more polydisperse and showed relatively small droplets (z-average < 323 nm; ). The size properties were independent of the ionic strength, so the average droplet size and PDI of the emulsions were not significantly affected by NaCl concentration (p > 0.05).
The emulsions were stable against phase separation (PSI = 0), but unstable as to creaming since all emulsions showed a vertical color gradient (–F3). Significant changes over time were observed only for the salt-free emulsion droplets (p < 0.05; ). As previously mentioned, the change in the surface charge after storage may be associated with possible rearrangements of emulsifiers’ layer adsorbed on the droplet surface, but the surface charge remained high and contributed to emulsion stability. Dynamic light scattering revealed that droplet size remained virtually unchanged and that the PDI did not significantly change (p > 0.05) throughout storage for 7 days at 25°C (). Maier, Zeeb, and Weiss[Citation36] investigated the formation of aggregates with oppositely charged emulsions containing different emulsifiers (including Quillaja saponin) at different pH values (3–7). They stated that substantial steric effect for droplet stabilization by Quillaja saponin can be generated by its large sugar side groups, which extend into the aqueous phase.[Citation37] Additionally, Yang et al.[Citation16] studied the formation and stability of emulsions using a natural food-grade surfactant containing QBS and compared its effectiveness with that of Tween 80, a synthetic surfactant. The authors evaluated emulsions at different pH (2–8), NaCl concentrations (0–500 mmol L−1) and temperatures (20–90°C) and suggested that steric repulsion contribute to stabilize saponin coated droplets against aggregation. Therefore, it is likely that the steric effect along with the electrostatic repulsion played a stabilizing role on the oil droplets against aggregation. At low NaCl concentration, the electrostatic repulsion between droplets was strong enough to prevent their aggregation. At high NaCl concentrations, on the other hand, the ζ-potential magnitude was relatively low (|ζ|< 30 mV) and, consequently, the force of repulsion between adjacent droplet decreased, but the steric repulsion appeared to be strong enough to prevent coalescence.
Conclusion
A mixture of QBS and β-lg was capable of forming emulsions with relatively small droplets at different conditions of pH and NaCl concentration as well as using 4 homogenization passes at 10,000 psi. All emulsions exhibited considerable polydispersity. The emulsions presented a negative surface charge that was influenced by both pH and NaCl concentration. The ζ-potential magnitude significantly decreased with increased salt concentrations and decreased pH values. On the other hand, no significant differences were observed in the average diameter of droplets formed under different pH values and NaCl concentrations. The emulsions showed a pseudoplastic behavior with the presence of small yield stress and low apparent viscosity. The viscoelastic behavior of the emulsions is typical of a weak-gel system, which indicated the presence of a weakly elastic three-dimensional structure. The emulsion formulated at neutral pH either in the absence of NaCl or in the presence of low salt concentrations was found to be stable against phase separation, but unstable against creaming. No significant changes in the average droplet size were observed after 7 days of storage at 25°C at any of the evaluated conditions, indicating that the emulsions were stable enough to prevent droplet coalescence. From the results of the physical properties, it is possible to infer that the electrostatic repulsion is not the only mechanism involved in emulsion stabilization, since emulsions with lower surface electrical charge remained stable after 7 days of storage at 25°C. Most likely, the main stabilization mechanism of QBS and β-lg emulsions is the steric hindrance conferred by the adsorbed emulsifiers on the droplet surface. Therefore, electrostatic repulsion among emulsifier segments surrounding oil droplets favored the emulsion stability under the conditions evaluated in this study.
Funding
The authors are thankful to the financial support provided by the Brazilian agencies CNPq and FAPEMIG.
Additional information
Funding
References
- Mcclements, D.J. Food Emulsions: Principles, Practices, and Techniques. Taylor & Francis: Boca Raton, Florida, 2004; 632.
- Dickinson, E. Hydrocolloids at Interfaces and the Influence on the Properties of Dispersed Systems. Food Hydrocolloids 2003, 17, 25–39.
- Wan, Z.-L.; Wang, L.-Y.; Wang, J.-M.; Zhou, Q.; Yuan, Y.; Yang, X.-Q. Synergistic Interfacial Properties of Soy Protein–Stevioside Mixtures: Relationship to Emulsion Stability. Food Hydrocolloids 2014, 39, 127–135.
- Kitamoto, D.; Isoda, H.; Nakahara, T. Functions and Potential Applications of Glycolipid Biosurfactants—From Energy-Saving Materials to Gene Delivery Carriers. Journal of Bioscience and Bioengineering 2002, 94, 187–201.
- Wojciechowski, K.; Piotrowski, M.; Popielarz, W.; Sosnowski, T.R. Short- and Mid-Term Adsorption Behaviour of Quillaja Bark Saponin and Its Mixtures with Lysozyme. Food Hydrocolloids 2011, 25, 687–693.
- Perrechil, F.A.; Ramos, V.A.; Cunha, R.L. Synergistic Functionality of Soybean 7S and 11S Fractions in Oil-in-Water Emulsions: Effect of Protein Heat Treatment. International Journal of Food Properties 2015, 18, 2593–2602.
- Wojciechowski, K.; Kezwon, A.; Lewandowska, J.; Marcinkowski, K. Effect of β-Casein on Surface Activity of Quillaja Bark Saponin at Fluid/Fluid Interfaces. Food Hydrocolloids 2013, 34, 208–216.
- Stanimirova, R.; Marinova, K.; Tcholakova, S.; Denkov, N.D.; Stoyanov, S.; Pelan, E. Surface Rheology of Saponin Adsorption Layers. Langmuir 2011, 27, 12486–12498.
- Golemanov, K.; Tcholakova, S.; Denkov, N.; Pelan, E.; Stoyanov, S.D. Surface Shear Rheology of Saponin Adsorption Layers. Langmuir 2012, 28, 12071–12084.
- Wojciechowski, K.; Kezwon, A.; Lewandowska, J.; Marcinkowski, K. Effect of β-Casein on Surface Activity of Quillaja Bark Saponin at Fluid/Fluid Interfaces. Food Hydrocolloids 2014, 34, 208–216.
- Piotrowski, M.; Lewandowska, J.; Wojciechowski, K. Biosurfactant–Protein Mixtures: Quillaja Bark Saponin at Water/Air and Water/Oil Interfaces in Presence of β-Lactoglobulin. The Journal of Physical Chemistry B 2012, 116, 4843–4850.
- Mitra, S.; Dungan, S.R. Micellar Properties of Quillaja Saponin. 1. Effects of Temperature, Salt, and pH on Solution Properties. Journal of Agricultural and Food Chemistry 1997, 45, 1587–1595.
- Kezwon, A.; Wojciechowski, K. Interaction of Quillaja Bark Saponins with Food-Relevant Proteins. Advances in Colloid and Interface Science 2014, 209, 185–195.
- Wojciechowski, K. Surface Activity of Saponin from Quillaja Bark at the Air/Water and Oil/Water Interfaces. Colloids and Surfaces B: Biointerfaces 2013, 108, 95–102.
- Mohan, C. Buffers: A Guide to the Preparation and Use of Buffers in Bological Systems. Calbiochem-Novabiochem International: La Jolla, California, 1995; 24.
- Yang, Y.; Leser, M.E.; Sher, A.A.; Mcclements, D.J. Formation and Stability of Emulsions Using a Natural Small Molecule Surfactant: Quillaja Saponin (Q-Naturale®). Food Hydrocolloids 2013, 30, 589–596.
- Cheftel, J.-C.; Cuq, J.-L.; Lorient, D. Protéines Alimentaires Biochimie, Propriétés Fonctionnelles, Valeur Nutritionnelle, Modifications Chimiques [Food Protein Biochemistry, Functional properties, Nutritional Value, Chemical Modifications]. Technique et documentation-Lavoisier: Paris, 1992; 310.
- Kuhn, K.R.; Cunha, R.L. Flaxseed Oil—Whey Protein Isolate Emulsions: Effect of High Pressure Homogenization. Journal of Food Engineering 2012, 111, 449–457.
- Maskan, M.; Gogus, F. Effect of Sugar on the Rheological Properties of Sunflower Oil-Water Emulsions. Journal of Food Engineering 2000, 43, 173–177.
- Khan, N.M.; Mu, T.-H.; Zhang, M.; Arogundade, L.A. The Effects of pH and High Hydrostatic Pressure on the Physicochemical Properties of a Sweet Potato Protein Emulsion. Food Hydrocolloids 2014, 35, 209–216.
- Fernández-Ávila, C.; Escriu, R.; Trujillo, A.J. Ultra-High Pressure Homogenization Enhances Physicochemical Properties of Soy Protein Isolate-Stabilized Emulsions. Food Research International 2015, 75, 357–366.
- Qiao, X.; Wang, L.; Shao, Z.; Sun, K.; Miller, R. Stability and Rheological Behaviors of Different Oil/Water Emulsions Stabilized by Natural Silk Fibroin. Colloids and Surfaces A: Physicochemical and Engineering Aspects 2015, 475, 84–93.
- Çakır-Fuller, E. Enhanced Heat Stability of High Protein Emulsion Systems Provided by Microparticulated Whey Proteins. Food Hydrocolloids 2015, 47, 41–50.
- Kuhn, K.R.; Silva, F.G.D.; Netto, F.M.; Cunha, R.L. Assessing the Potential of Flaxseed Protein as an Emulsifier Combined with Whey Protein Isolate. Food Research International 2014, 58, 89–97.
- Khan, N.M.; Mu, T.-H.; Sun, H.-N.; Zhang, M.; Chen, J.-W. Effects of High Hydrostatic Pressure on Secondary Structure and Emulsifying Behavior of Sweet Potato Protein. High Pressure Research 2015, 35, 189–202.
- Hunter, R.J. Introduction to Modern Colloid Science. Oxford University Press: Oxford, UK, 1993; 352.
- Steffe, J.F. Rheological Methods in Food Process Engineering. Freeman Press: East Lansing, Michigan, 1996; 418.
- Tokita, M.; Nishinari, K. Gels: Structures, Properties, and Functions: Fundamentals and Applications. Springer Berlin Heidelberg: Japan, 2009; 213.
- Santos, J.; Calero, N.; Guerrero, A.; Muñoz, J. Relationship of Rheological and Microstructural Properties with Physical Stability of Potato Protein-Based Emulsions Stabilized by Guar Gum. Food Hydrocolloids 2015, 44, 109–114.
- Brummer, R. Rheology Essentials of Cosmetic and Food Emulsions. Springer Berlin Heidelberg: Germany, 2006; 180.
- Güçlü-Üstündağ, Ö.; Mazza, G. Saponins: Properties, Applications and Processing. Critical Reviews in Food Science and Nutrition 2007, 47, 231–258.
- Yang, Y.; Mcclements, D.J. Encapsulation of Vitamin E in Edible Emulsions Fabricated Using a Natural Surfactant. Food Hydrocolloids 2013, 30, 712–720.
- Müller, R.H.; Nitzsche, R.; Paulke, B.R. Zetapotential und Partikelladung in der Laborpraxis: Einführung in die Theorie, praktische Messdurchführung, Dateninterpretation [Zeta potential and particle charge in the laboratory:Introduction to the theory and practical test execution, data interpretation]. Wiss. Verlag-Ges.: Germany, 1996; 2254.
- Romero, A.; Cordobés, F.; Guerrero, A. Influence of pH on Linear Viscoelasticity and Droplet Size Distribution of Highly Concentrated O/W Crayfish Flour-Based Emulsions. Food Hydrocolloids 2009, 23, 244–252.
- Hunter, R.J.; White, L.R. Foundations of Colloid Science. Clarendon Press: Oxford, New York, 1987; 816.
- Maier, C.; Zeeb, B.; Weiss, J. Investigations into Aggregate Formation with Oppositely Charged Oil-in-Water Emulsions at Different pH Values. Colloids and Surfaces B: Biointerfaces 2014, 117, 368–375.
- Ikedo, S.; Shimoyamada, M.; Watanabe, K. Interaction Between Bovine Serum Albumin and Saponin as Studied by Heat Stability and Protease Digestion. Journal of Agricultural and Food Chemistry 1996, 44, 792–795.