ABSTRACT
In this study principal component analysis and artificial neural networks were used to evaluate the potential of using binary mixtures of sodium alginate and other polysaccharide biopolymers as the carriers for microencapsulation of green tea bioactive compounds. Using binary mixtures of alginate and adjunct biopolymers increased the particle size (from 722 to 1344 µm) and textural parameters of the microbeads. Chemometric techniques revealed the combination of biopolymers and their ratio as the main factors influencing the encapsulation performance. The combination of alginate with hydroxypropyl methylcellulose and locust bean gum enabled to retain the highest (-)-epigallocatechin gallate and caffeine contents, the highest total phenols encapsulation efficiency, and their most retarded release in water, confirming these as the best delivery systems of polyphenol-type active compounds and signifying their potent food applications.
Introduction
Improvement of the physicochemical, functional, and release properties of encapsulated biopolymeric matrices is currently one of the most extensively studied ongoing research subjects, followed by achieving cost-effective delivery systems of various active ingredients.[Citation1] Alginate, an anionic polymer with carboxyl end groups, is characterized with biocompatibility and simple gelation with divalent cations, thus producing rigid hydrogels;[Citation2] however, alginate hydrogel beads exhibit very porous structure and poor physico-mechanical properties, crucial for the potential food applications of formulated delivery systems. Previous research indicated that incorporation of some substances can modify the physical and even chemical properties of calcium-alginate beads. Some authors have reported that alginates can form strong complexes with other natural polyelectrolytes, which improves the mechanical and chemical stability of beads, thus improving the encapsulation effectiveness.[Citation3,Citation4] Binary mixtures of alginate and other polysaccharide bipolymers such as cellulose derivatives, starch or xanthan gum were also evaluated as the delivery systems of different active compounds.[Citation1,Citation5–Citation13] However, a systematic study of macromolecular interactions and variables that modulate those interactions and affect the encapsulation performance is required. Moreover, there is a lack of studies evaluating the interactions and potential of biopolymer mixtures of alginate and other hydrocolloids for the entrappment of naturally derived bioactive compounds, since most of the studies are still conducted with the purpose of encapsulating pharmaceutically active compounds. Green tea is currently one of the most popular research substrates and acknowledged nutraceutical as a consequence of high concentrations of polyphenolic compounds and caffeine as the main representative bioactive compounds.[Citation14] However, due to the low molecular weight, their entrapment is very difficult to be achieved in the porous alginate-based particles, so specific interactions occuring between alginate and other polysaccharides constituting the delivery matrix may be of crucial importance to increase the encapsulation efficiency of microencapsulates.
In order to achieve the optimal process performance, especially in the field of food technology and biotechnology, it is beneficial to use methods and software tools to understand the obtained process results. Chemometric techniques, especially principal component analysis (PCA) and artificial neural network (ANN) modeling gained increased importance in recent years due to higher accuracy in modeling and better ability in generalization, as well as the ability to simulate the bio-process and predict the results.[Citation15,Citation16] Those multivariate statistical techniques still do not have a wider application in the encapsulation field, so their use in computing, interpreting, and predicting the optimal encapsulation parameters would be a quite novel approach in this field. Therefore, this study was focused on evaluating the potential of binary mixtures of sodium alginate and other polysaccharide biopolymers; pectin, carageenan, modified starch, hydroxypropyl methylcellulose (HPMC), xanthan gum, locust bean gum (LBG), and agar as the carriers for encapsulation of green tea bioactive compounds as the model substrate. Potential interactions between the delivery systems constituents (carriers and bioactives) were characterized by Fourier transform infrared (FTIR) spectroscopy, while the evaluated physico-chemical and bioactive characteristics such as the particle size, textural properties of produced hydrogels, and entrapped bioactives compounds content of formulated microbeads were computed using multivariate statistical techniques such as PCA and ANN.
Materials and methods
Chemicals
All chemicals used for the experimental procedures were of analytical grade. Sodium alginate (molecular weight: 80–120 kDa) was purchased from Sigma-Aldrich (St. Louis, USA). Pectin (low methoxyl pectin, molecular weight: 70–140 kDa), κ-carageenan, xanthan gum, LBG, modified corn starch and agar from Cargill (Zagreb, Croatia). HPMC was supplied by Alfa Aesar GmbH and Co. (Karlsruhe, Germany). Green tea Darjeeling (Müller, Abtswind, Germany) was purchased at a local organic market.
Preparation of green tea extract
Green tea leaves were ground using a domestic grinder Braun KSM2 (Braun, Kronberg, Germany) and sieved through 0.45 mm pore-size sieve to obtain a homogenized fraction. The extraction was carried out repeatedly (2-fold), by pouring 100 mL of distilled water heated to 80°C over 8 g of ground tea, and mixing on a magnetic stirrer at 80°C for 15 min, whereafter the obtained extract was filtered through a tea strainer and the crude residue again extracted with another 100 mL of distilled water in the same way as during the first extraction. After the extraction, the supernatants were collected, filtered through a 4-layer cotton gauze and filled to 200 mL.
Preparation of binary alginate-biopolymer blended mixtures
The preparation of microbeads was carried out by ionic gelation technique following the method adopted by Belščak-Cvitanović et al.[Citation17] Plain sodium alginate (1.6% A and 1.4% A) and seven binary mixtures of sodium alginate with different biopolymers were produced. Binary mixtures were formulated from alginate and agar (AAg), κ-carrageenan (ACar), hydroxypropyl methylcellulose (AHPMC), locust bean gum (ALbg), pectin (APec), starch (AStarch), and xanthan (AXan) by dissolving sodium alginate or the respective biopolymer in the prepared green tea extract and stirring until complete dissolution. Each prepared solution of different biopolymers was blended with sodium alginate solution in mass proportions of 80:20 (w/w) and 70:30 (w/w), in favor of alginate. All blended formulations were well homogenized and sonicated for 15 s in an ultrasound bath.
The prepared delivery solutions were microencapsulated using the electrostatic droplet extrusion by a syringe pump (Razel Scientific Instruments, Stamford, CT, USA), through a blunt stainless steel needle (18–22 gauge) at a constant flow rate of 25.2 mL/h. The electric field potential difference was controlled by a high-voltage unit (Model 30R; Bertan Associates, Inc., New York, USA) with the voltage varying between 4.0 and 6.0 kV, depending on the formulation (). The crosslinking solution consisted of 2% (w/v) calcium chloride prepared in the green tea extract. After the ions exchange, the microparticles were allowed to stir gently in the collection solution and then stored in that way until the analysis. All obtained microparticles were stored in the original extract containing 2% (w/v) calcium chloride in the dark at 4°C, while a proportion of the hydrogel beads was subjected to freeze drying. Prior to analyses, the beads were separated from the storage medium, washed with distilled water, and blotted gently by a filter paper to remove excess liquid.
Table 1. Particle size distribution and textural parameters of formulated hydrogel microbeads encapsulating green tea bioactive compounds.
Particle size determination
Particle size of the obtained microparticles was determined using Mastersizer 2000 (Malvern Instruments, Worcestershire, UK) equipped with the Hydro 2000S dispersion unit. About 0.5 g of hydrogel microbeads was dispersed in 5 mL of water at ambient temperature (20 ± 2°C) and then added to the Hydro S dispersion unit. Water was used as the dispersant, until appropriate obscuration was achieved. Stirring and ultrasonic dispersion for 2 min was conducted to ensure particles were independently dispersed. All measurements were performed in triplicate. PSD parameters obtained included the largest particle size d(0.9), mean particle size d(0.5), smallest particle size d(0.1) and surface weighted mean. The PSD width (span) of the size distribution was calculated as follows:
Instrumental textural analysis
Instrumental analysis of hydrogel beads was carried out using a TA.HDPlus texture analyzer (Stable Micro Systems, Godalming, Great Britain), which measures the applied force during time and produces force-time and force-deformation graphs. A compression test was performed using a 4 mm metal (stainless steel) cylindrical probe with flat bottom and a 5 kg load cell. Each (single) bead was positioned on the center of the holder plate. Beads were compressed to >50% of their diameter or to the breaking point (whichever comes first) at the speed of 0.5 mm/s. Changes in force during decompressing phases were measured in intervals of 0.1 ms, and results were later obtained from the force-time and force-deformation graphs. Each analysis was executed 5-fold (on five different large beads of approximately equal dimensions [>2 mm], obtained by dripping method instead of electrostatic extrusion). All bead dimensions were taken into account by their initial input in the software, allowing the recalculation of results based on the bead diameter, which, in turn, ensures consistent results independently of the bead size and diameter. The analyses were carried out at room temperature. The maximum force (N) needed for compression, which represents maximal resistance of the surface to compression of probe, gives an indication of the hardness of the samples. Elasticity of samples was measured as a ratio of probe travel distance and the diameter of beads, and was expressed in %. All calculations were performed using Texture Exponent 6.0 software (Stable Micro Systems, Great Britain).
Encapsulation efficiency and bioactive compounds
The content of total and specific polyphenols entrapped in the obtained beads was estimated by dissolving a known amount of capsules in 2% sodium citrate (w/v), under vigorous shaking on a Vortex mixer (Tehnica, Železniki, Slovenia) at an ambient temperature. The content of total polyphenols was determined using the Folin-Ciocalteu assay,[Citation18] and the contents of (-)-epigallocatechin gallate (EGCG) and caffeine loaded in the microbeads by high-performance liquid chromatography (HPLC) analysis. The percentage of loading efficiency was calculated as the ratio between the total polyphenols content in the citrate solution of dissolved beads and their respective content in the initial solution. For the HPLC analysis, all samples were filtered through a 0.45 µm filter (Nylon Membranes, Supelco, Bellefonte, USA) before HPLC analysis. The sample (20 µL) was injected for HPLC analysis using a Agilent 1100/1200 Series HPLC device (Agilent, Santa Clara, USA) and a photodiode array detector (Agilent, Santa Clara, USA) by using a reversed-phase column Zorbax extended C-18 column (Agilent, Santa Clara, USA; 250 × 4.6 mm, 5 µm i.d.). The solvents consisted of 2% formic acid in water (solvent A) and methanol (solvent B) at a flow rate of 1 mLmin−1. The elution was performed with a gradient starting at 10% A, rising to 40% A after 25 min, and then to 70% A after 15 min. Chromatograms were recorded at 278 nm. Detection was performed with a photodiode array detector by scanning between 200–400 nm, with a resolution of 1.2 nm. Caffeine was identified by comparing the retention times and spectral data with those of standards. The data acquisition and treatment were conducted using Star Chromatography Workstation Version 5 software. All analyses were repeated three times.
Release profile of total polyphenols from different carrier systems
The release of total polyphenols was estimated from the obtained hydrogel microbeads in distilled water by evaluating the total polyphenols content using the Folin-Ciocalteu assay (as described in the Encapsulation efficiency section). For the analysis, a known amount of microparticles (of about 3 g) was suspended in 50 mL of the media (distilled water). The samples were submitted to continuous agitation at room temperature on an orbital shaker (New Brunswick Scientific Co., Inc., Edison, NJ), operating at 100 rpm. At defined time intervals, an aliquot of the supernatant was taken for analysis and replaced by the same amount of fresh medium.
FTIR spectroscopy analysis
For the FTIR spectroscopic analysis of plain green tea extract, biopolymer materials used as the carriers, plain binary mixtures, and obtained microbeads encapsulating the green tea extract, the stated samples were crushed using a mortar and pestle, mixed with potassium bromide, dried, and compressed to pastilles. Infrared spectra over the wavelength range 4000 to 600 cm−1 were recorded as KBr pastilles with a Bomem MB 100 mid FTIR spectrometer. The resolution was 4 cm−1. The effect of binary mixtures ratio (80:20 and 70:30) was not presented in the current article since it was revealed that the difference in the binary mixtures ratio had no effect on FTIR spectra of the samples.
Chemometric techniques and statistical analyses
The results were analyzed statistically using the commercial statistical software STATISTICA v. 10 (StatSoft Inc., USA) to determine the average value and standard error. Variance analysis, with a significance level of α = 0.05%, was performed to determine the influence of carrier system formulations on the encapsulation attributes of obtained microbeads. The PCA, calculated using the STATISTICA v. 10 software, was based on the correlation matrix between the values of the characteristics, which means that the contribution of each variable was independent of the range of its values. In order to verify the sampling adequacy of the data set, the Bartlett’s Test of Sphericity and the Kaiser-Meyer-Olkin (KMO) value were determined prior to performing the PCA analysis by using the SPSS v. 17.0 software (SPSS Inc., Chicago, USA).
An ANN was designed for the correlation analysis of main output parameters (encapsulation efficiency of total polyphenols and release percentage of polyphenols) to input variables. For the production of ANN five input and 11 output parameters were used. Continuous input parameters were the concentration of used carrier material, needle size, and voltage for the production of hydrogel particles, while categorical input parameters was the type of carrier combination (biopolymer). The selected feed-forward, back propagation network type before training was multilayer perceptron (MLP) with up to 10 hidden units. Network training was performed with separation of experimental data into training and validation sets as 70:30 ratios. Selection of the optimal neural network architecture was performed comparing the values of the root mean square error (RMSE) and the linear correlation coefficient (R2). The final selected network was MLP 11-9-14 using BFGS 3718 algorithm.
Results and discussion
Particle size distribution of microencapsulates
The particle size analysis () of obtained microbeads revealed a high variability of all PSD parameters in dependance on the binary mixture, i.e., biopolymer composition. According to the mass median diameter d0.5, the size of formulated microparticles varied between 722.90 µm (A1.6%, control) and 926.10 µm (AAg 80:20), with the exception of alginate and carageenan mixture in case of 70:30 ratio, where markedly larger particles were formed ranging up to 1344.56 μm (ACar 70:30). If comparing the d0.1 and d0.5 size parameters for each formulated delivery system, no marked differences in the established values can be observed, indicating the uniformity and size homogenicity of electrostatic-extrusion produced microbeads. By using binary mixtures of alginate and other polysaccharides as the carriers, larger particles than in case of plain control alginate were obtained and augmented PSD width of produced microparticles was determined, revealing poorer size homogenicity obtained by using those combinations as the delivery matrices. Apart from the binary mixture composition, the ratio of alginate and reinforcing material also exhibited a significant effect on the particle size of obtained microbeads. The microencapsulates formulated from the mixtures composed of 80% of alginate and 20% of adjunct biopolymers were all characterized with higher d0.5 in comparison to plain, control alginate particles (A1.6%); however, in the case of the 70:30 binary mixtures ratio (mixtures composed of 70% of alginate and 30% of other biopolymers), no significant differences between control alginate (A1.4%) and alginate-agar, alginate-starch, and alginate-pectin particles were observed. Such observations could be a consequence of the changes induced in the polymeric structure by ionic interactions between Ca2+ and the polysaccharide chains. For example, in case of large particles, like the previously mentioned AAg 80:20 and ACar 70:30, it may be possible that the incorporation of agar and carageenan made the calcium bonding sites of alginate chains less accessible and subsequently less crosslinked, which results with more intensively swelled microbeads and thus, their larger size.
Textural properties of microencapsulates
The textural parameters () of formulated microbeads were affected by both carrier system composition and ratio. Tukey’s test revealed a significant difference (p < 0.05), predominantly in terms of hardness and springiness of binary microbead mixtures. The hardness of all tested beads was in the range between 0.30–0.62 N, with the highest hardness of ALBG 80:20 microparticles. This could be explained by the low chelation level of LBG,[Citation19] leaving more free Ca2+ ions to react with alginate, thus creating a more rigid and hard microbead structure. Additionally, the possibility of creating separate aggregates of LBG within the hydrogel matrix may exist, because of its hydrocolloid, self-gelling abilities, influencing the final hardness of beads. No significant differences in the hardness of plain alginate microparticles, regardless of the alginate concentration (1.4 or 1.6%) were observed, while in case of binary mixtures the lack of a unique trend with respect to the binary mixture ratio (80:20 or 70:30) was obtained; instead, the hardness of the beads was in dependance on the binary mixture composition. By increasing the content of reinforcing biopolymer from 20 to 30%, a decrease in hardness of AAg, ALBG, Astarch, and AXan was observed; however, a marked increase was exerted in case of AHPMC and APec microparticles. The pronounced hardness of alginate-pectin beads (APec 70:30) may be explained by the calcium-alginate-pectinate crosslinking reactions, which resulted in the creation of a rigid polymeric network.[Citation3] The lowest hardness was determined in case of both binary mixture ratios of alginate-carageenan beads, which may be the consequence of the carageenan flexible structure and the formation of weak, less rigid physical hydrogels resulting with low hardness.[Citation20] This is in accordance with the largest particle size of ACar beads, since the weak gelling ability of this binary mixture and potentially weaker intermolecular associations may impart low hardness and largest particle size of the ACar beads. According to the obtained results, using gums and starch as the carrier materials for ionic gelation-based encapsulation in alginate hydrogel results with lower hardness, which corroborates to some reports stating that in the presence of gums the hardness of beads decreases.[Citation21]
Springiness (%) or the elasticity of formulated microbeads varied between 65.85 and 91.21%. Alginate-pectin mixture (70:30) was characterized with the highest elasticity in comparison to all other investigated delivery combinations. According to the results of Draget et al.,[Citation22] the incorporation of more elastic, flexible segments (e.g., pectin) can permit a more rapid relaxation as a mean of mechanical response to deformation, but enables a high syneresis intensity. In contrast, employing hydrocolloid gums such as xanthan, LBG, or HPMC provides more elastic gels, with low syneresis levels.[Citation23] According to a study by Pongjanyakul and Puttipipatkhachorn,[Citation24] higher xanthan gum contents decreased the syneresis intensity of the beads, which may be attributed to the formation of intermolecular hydrogen bonding between xanthan gum and Ca2+-alginate. Results of the present study confirm the higher elasticity of beads composed of binary mixtures of alginate and xanthan, LBG, or HPMC.
It has generally been established that the extent of entanglement and retractive forces within the polymeric networks can limit the expansion/contraction of microbeads and thus, influence the mechanical and textural properties of formulated beads.[Citation25] This is also reflected with respect to cohesivity of the beads, since as the particle size decreases, the intermolecular forces between the microbeads become stronger and the cohesivity of the particles increases. The same was observed in case of plain alginate beads, which were characterized with low particle size and high cohesivity. Correspondingly, ACar 70:30 beads exhibiting the largest particle size (d[0.5]: 1344.56 mm) were characterized with the lowest cohesivity of 1.11. Work (mJ) required for the compression of formulated microbeads was in an inversely proportional relation to the cohesivity, since it can be observed that AHPMC 80:20 beads with a high cohesivity were characterized with the lowest work, and vice versa. ALBG 80:20 beads with a low cohesivity required the highest work for the maximum compression of the beads.
Encapsulation efficiency and loading of specific polyphenols in formulated microbeads
Encapsulation efficiency of bioactive compounds from the green tea extract () was quantified as the loading capacity of total polyphenols in relation to the initial polyphenolic content of binary mixture previous to ionotropic gelation and microbead formation, and was expressed as a relative value (%). Generally, by increasing the content of adjunct biopolymer of the binary carrier mixture (and consequently decreasing the alginate content) from 20 to 30% a decrease in total phenols encapsulation efficiency was observed for all delivery systems apart from the alginate-pectin (APec) combination. This can be attributed to the pectin gelling mechanism, which is also based on ionotropic gelation by divalent calcium ions, due to the formation of intermolecular junction zones between homogalacturonic regions of different chains. Therefore, it may be possible that association of two polymers enabled to retain more polyphenolic compounds as the consequence of increasing the pectin content in APec particles. With regard to the carrier (binary mixture) composition, the use of pectin, agar, and carageenan did not enable to entrap a higher polyphenolic content in comparison to plain alginate particles, regardless of the mixture ratio. In contrast, by employing gums (xanthan and LBG), starch, and HPMC, significantly higher encapsulation efficiencies were achieved. The highest encapsulation efficiencies were observed in case of using alginate-HPMC and alginate-LBG as the binary carrier delivery matrix. The highest encapsulation efficiency of AHPMC microbeads is attributed to specific interactions of green tea catechins with HPMC. It has been previously revealed that hydrophobic interactions and hydrogen bonding are responsible for creating sufficiently strong molecular associations between catechins and HPMC, so that the catechins may act as crosslinkers to produce polyphenol-HPMC beads.[Citation26]
Figure 1. Encapsulation efficiency (%) of A: total polyphenols; B: epigallocatechin gallate (EGCG); and C: caffeine contents (mg/g beads) entrapped in the formulated microparticles.
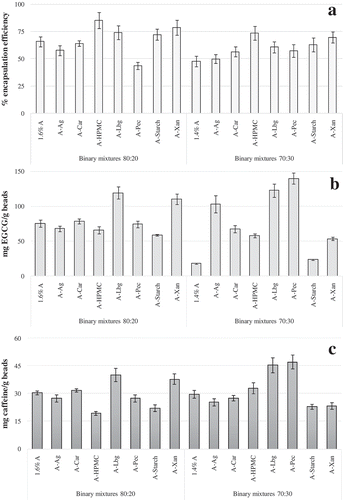
The contents of main representative, specific polyphenolic compounds of green tea, EGCG, and caffeine retained within the formulated microbeads were also determined. A decrease of EGCG content with the increase of adjunct biopolymer from 20 to 30% in the carrier mixture was observed (), apart from the combinations of AAg, pectin, or LBG. In case of caffeine (), increasing the adjunct biopolymer content (70:30 ratio microbeads) resulted with insignificant differences in comparison to 80:20 ratio microbeads, while in case of using HPMC, LBG, and pectin even an increase in the retained caffeine content was observed. The results imply on a variable effect of adjunct biopolymer added to the binary carrier mixtures that results with diverse entrapment efficiency of specific polyphenols. Namely, the ranking of encapsulation or retained bioactive compounds differs between total polyphenols, EGCG, and caffeine. The commune biopolymer that enables to retain more of all three compound groups while augmenting its content from 20 to 30% is LBG. Apart from this biopolymer, using HPMC enables to retain a high total polyphenols and caffeine content in the microbeads formulated from alginate and these carrier types.
Release profiles of total polyphenols from green tea extract encapsulating microbeads
The release profiles of polyphenolic compounds in water were quantified as the TPC in mg gallic acid equivalent (GAE)/g beads, as well as the percentage of cumulative total phenol release (). According to the results, the major content of microbeads was released within the first 10 min, whereafter a steady state and/or slight increase was reached. The fast release of polyphenolic compounds from the alginate microbeads in water is attributed to the initial burst release of polyphenolic compounds located on the surface of the beads. Upon the initial release, the release of total polyphenols from the alginate microbeads is known to be diffusion controlled, while the pronounced alginate gel porosity enables the diffusion mediated release of polyphenols during prolonged residence in a liquid medium. The constant release of polyphenolic compounds can be observed for some delivery systems (plain alginate), while even a decreasing tendency of the total polyphenols release curve can be observed for blended systems indicating the potential reverse difussion of polyphenols into the particles. Only in the case of AHPMC and ALBG microbeads, a sharper gradual increase of polyphenols occured for up to 240 min. These results are in agreement with the ones of Giunchedi et al.[Citation27] who determined that HPMC enhanced the sustained-release properties of alginate beads by providing a dense inner matrix. This is also in correlation with the results of AHPMC beads high cohesivity, denoting a more consistent and unified structure of the beads, which was due to more pronounced intermolecular associations that may have retarded the difussion mediated release of polyphenolics from the beads in water. In case of the ALBG 80:20 binary mixture, prolonged release profile was also apparent with its peak and eqilibrium state reached at 240 min. A slower, prolonged release profile can be explained by the increase in the extent of swelling and the gel layer thickness that acted as a barrier for the penetration medium, thereby retarding the diffusion of the extract from the swollen ALBG microbeads.[Citation5]
FTIR spectroscopy analysis
The IR spectrum of alginate contains the absorption bands of free (3564 cm−1) and associated (3318 cm−1) OH groups, carboxylate salt ion (1600 and 1412 cm−1), skeletal vibration band (1296 cm−1), and C–O–C stretching vibrations of pyranosyl ring (1095–1026 cm−1). Shifting in the peak position of the main functions enables the monitoring of chemical interactions between two polysaccharides.[Citation28] The comparison of IR spectrum of alginate-LBG matrix to those of pure alginate and LBG revealed the strengthening of the hydrogen-bonded OH bands followed with shouldering of the band at 1600 cm−1, confirming the potential interactions between these biopolymers (). Incorporation of xanthan into alginate matrix induced not only the strengthening of bonded OH peak but also the shifting of COOˉ stretching peak from 1600 to 1613 cm−1, strongly indicating the formation of intermolecular hydrogen bonds between both components (). Pronounced intensity of the band of OH groups was observed upon addition of pectin to alginate, owing to their engagement in hydrogen bonds (). Furthermore, changes were observed in the carbohydrate finger print region (950–1250 cm−1) of alginate-pectin IR spectrum, suggesting the presence of pectin galactouronic acid moieties. The different chemical environment around carbonyl groups upon addition of carrageenan to alginate matrix is suggested, due to the shifting of their absorption bands. The IR spectrum of alginate-carrageenan mixture is altered in the carbohydrate fingerprint region by appearance of new bands (1240 and 924 cm−1) characteristic for carrageenan (). Spectroscopic characterization of starch-alginate binary mixture reveals the strengthening and shifting of the band of associated OH groups from 3318 to 3293 cm−1, suggesting the improvement of hydrogen bonding. Thus, the peak of hydrogen-bonding-accepting C=O group is modified (from 1600 to 1618 cm−1) due to the exchanged hydrogen-bonding pattern (). The presence of starch component in its mixture with alginate is additionally supported by the presence of its specific peaks in carbohydrate finger print region (1163, 1077, and 1022 cm−1). The incorporation of HPMC into alginate matrix influenced the region of OH groups and carbohydrate finger print region. Namely, the absorption band of bonded OH group was shifted from 3318 to 3329 cm−1, suggesting that the hydrogen-bonding motif was changed in the presence of HPMC. In addition, the intensity increment of the peaks presented in the carbohydrate finger print region of the binary mixture HPMC-alginate was observed ().
Figure 3. FT-IR spectra of plain biopolymers (alginate, polysaccharides A: locust bean gum; B: xanthan; C: pectin; D: carageenan; F: starch; and G: HPMC) and their binary mixtures.
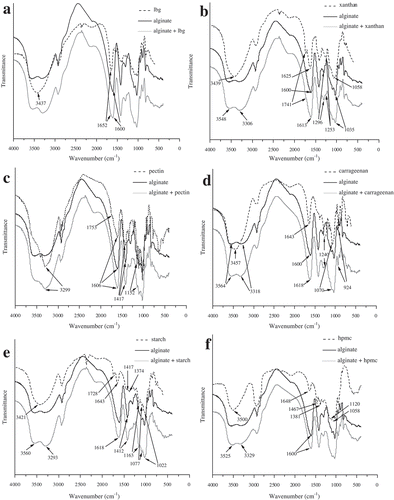
The encapsulation and entrappment of green tea bioactives identified by FTIR analysis will be discussed on the example of AAg binary mixture as a delivery system. Alteration of hydrogen-bonding pattern, i.e., strengthening of the bands of bonded OH groups followed with shifting of the C=O stretch peak from 1638 to 1600 cm−1, was observed upon addition of agar to alginate matrix (). Unlike the peaks at 1250 cm−1 (S=O of sulphate esters) and 864 cm−1 (L-galactopyranose-6-sulfate), the bands related to glycosidic bonding (1072 cm−1) and C-O-C bending of 3,6-anhydro-L-galactopyranose moiety in agar (932 cm−1) were not shifted in alginate-agar IR spectrum.[Citation29] The IR data of green tea extract are in good agreement with the literature data. A strong band observed at 3306 cm−1 corresponds to OH stretching, aromatic C=C bending is represented by bands at 1698, 1638, and 1606 cm−1 and C-O-C of aromatic alcohol is evident from the bands between 1143 and 1028 cm−1. The encapsulation of green tea extract with the predominant catechin fraction containing five hydrogen-bonding donating OH groups into alginate-agar binary matrix is expected to influence the hydrogen-bonding pattern established through intermolecular interaction. Accordingly, the IR curve in the region of free and bonded OH groups is somewhat altered and the COOˉ peak from binary matrix (1600 cm−1) is split, suggesting the new chemical environment upon binding of green tea extract constituents (). Moreover, the IR spectrum of formulated microbeads contains the peaks attributed to the C-O-C stretching of aromatic alcohol. A closer inspection of the IR spectrum obtained upon encapsulation of green tea extract within the binary mixtures of alginate with LBG, pectin, carrageenan, xanthan, starch, and HPMC, respectively, revealed a significant resemblance with the IR behavior of the previously described encapsulate Aag (). These findings strongly support the entrapment of green tea bioactives within the examined binary mixtures.
PCA and ANN modeling
In order to get a better insight in the relationship between the particle size, textural and bioactive properties of formulated microparticles, PCA was used as a statistical tool that would indicate multivariate dependence among variables. Principal component weighting for the formulated delivery systems was done for the entire data set, along with conducting the sampling adequacy tests, more specifically the Bartlett’s Test of Sphericity and calculating the KMO value. The Bartlett’s Test of Sphericity revealed the p-value to be 0.000 (<0.001), indicating the suitability of results for conducting a valid factor analysis. The KMO value amounted to 0.673 which positioned the data set to a mediocre (moderate) relevance according to some interpretations of the KMO index. However, by taking into account both sampling adequacy measures the obtained calculations confimed the relevance of the results dataset for further factor analysis, which was supported by the fact that no reported scientific data could have been found on the application of PCA in the encapsulation efficiency determination, so this proposed approach could be well informative and beneficial to scientists dealing with similar research subjects.
Higher weighting scores indicate a tighter association with that principal component (). The levels of variation described by each of the calculated principal components computed based on the data obtained in this study revealed that PC-1 describes 28.60% of the variation in the sample set, PC-2 describes 17.78%, whereas PC-3 describes 16.01% and PC-4 an additional 14.36%. and graphically depict the distribution of data for PC-1 and PC-2, and PC-2 and PC-3. As can be seen in , the textural parameters of hardness and work, with the highest weighting on PC-1 (), exhibit the highest values for the samples ALBG 80:20, and AXan 80:20, which are located on the most right part of the graphical display. Similarly, caffeine content also with the second highest weighting on PC-1 is the highest for the sample APec 70:30 located mostly right, while the grouping of samples consisting of samples APec 70:30, ALBG 70:30, ALBG 80:20, and AXan 80:20 indicates the samples with the highest caffeine and EGCG contents (weighted high on PC-1). Correspondingly, the samples located mostly on the left side of the graphical display (AHPMC 80:20 and ACar 70:30) were characterized with the lowest hardness and work, as well as the lowest caffeine content. In case of plotting the factor 1 and 3 coordinates (PC-1 versus PC-3), it can be seen that sample ACar 70:30 located mostly up (the highest) on the graphical display () with the highest weighting score on PC-3 for d0.5 has the highest particle size (median). Similarly, the samples A1.4 and A1.6% located mostly on the bottom along the y-axis (PC-3) exhibit the lowest d0.5 particle size. The distribution and significant dispersion of the samples and the lack of an obvious grouping indicate that the compositional diversity and ratio of used binary mixtures, as well as the extrusion parameters (voltage and needle size) for the formulation of microbeads are the result for the observed differences and dispersion of the results. Therefore, an additional statistical technique was applied to reveal which of the factors and in what measure contribute and mostly affect the evaluated characteristics of the microbeads.
Table 2. Principal component weighting of the data set.
Figure 5. Plot of A: PC-1 versus PC-2; and B: PC-2 versus PC-3 for the formulated microbeads composed of 80:20 and 70:30 binary mixtures.
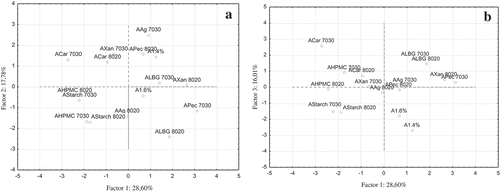
An ANN is a non-linear computing system consisting of a large number of interconnected processing units (neurons), which simulates the human brain learning.[Citation30] Based on the ANN computed from the data obtained in the present study, the most suitable network was selected based on training and validation preferences and errors, as previously stated by Di Scala et al.[Citation31] and Carvalho et al.[Citation32] The selected network had a Tanh hidden activation function and identity output function, with the lowest validation error of 0.072 achieved in the training period, and was selected for analysis of the formulated binary mixtures.
The global sensitivity analysis revealed the ranking of input parameters as the major factors that influence mostly the output parameters of microbeads produced from different binary mixtures: composition (type) of the carrier material > concentration (ratio) of carrier solutions > extrusion voltage > needle size. In this way the composition (type) of the binary mixtures and their concentration (ratio) were revealed as the prevalent factors influcencing the output parameters of microbeads encapsulating green tea extract. The highest correlation between the experimentally obtained and ANN predicted results were obtained for the percentage of encapsulation efficiency of total polyphenols, followed by the EGCG and caffeine contents (all exceeding R2 > 0.99), while the lowest correlation was observed for textural parameters of formulated hydrogel particles (R2 > 0.80).
For achieving the maximum encapsulation efficiency of total polyphenols (TP%), the optimal analyzed delivery formulation was the binary mixture of alginate and HPMC, in the ratio of 80:20%, respectively, and formulating microparticles at the electrostatic extrusion parameters of 5.5 kV of voltage and 22 Gauge needle size. The same extrusion parameters, i.e., the needle size and extrusion voltage equal to or above 5.0 kV were predicted by the selected ANN as the optimal ones for providing the smallest microparticles (d0.5 in the range between 700 and 800 µm). In the case of predicting the optimal microencapsulation conditions while taking into account EGCG and caffeine as the output parameter, the best case scenario for maximizing both output parameters was achieved by using pectin and LBG as the carrier combination in the content of 20% added to alginate. The experimentally obtained results also revealed pectin, LBG, and HPMC as the best carrier adjuncts for achieving the optimal bioactive (encapsulation) parameters, indicating that the combination obtained by the ANN modeling approach was in agreement with the experimentally obtained results.
Conclusion
The results obtained in the present study provide a comprehensive overview of the main characteristics of microparticles encapsulating green tea bioactive compounds in the binary mixtures of alginate and different biopolymers as the delivery systems. Each formulated delivery system revealed to be specific in terms of different physico-chemical or bioactive content characteristics, while FTIR measurements conducted before and upon encapsulation of green tea polyphenols evidenced specific molecular interactions for each binary mixture. Modifications of the IR curve of microbeads encapsulating green tea bioactives occured in the region of free and bonded OH groups, as well as COOˉ peak and C-O-C stretching of aromatic alcohol, confirming the entrapment of green tea constituents within the formulated delivery systems. With regard to the particle size, the combination of alginate and carageenan provided the largest particles, while plain alginate acquired the smallest ones. Increasing the content of adjunct, reinforcing biopolymer from 20 to 30% in the binary mixture with alginate resulted with increased hardness of formulated beads, but a decrease in the encapsulation efficiency of total polyphenols. The highest encapsulation efficiencies were observed in the case of alginate-HPMC and alginate-LBG employed as the binary carrier delivery matrix, which also provided the most retarded release of these compounds in water. The increment in EGCG and caffeine contents upon the scaling up of the content of LBG and pectin from 20 to 30%, accompanied by the evaluated and described features, implies that alginate in combination with LBG, HPMC, and pectin provides the the most efficient delivery system for polyphenol-type active compounds.
Funding
This work was supported by the COST action FA1001 “The application of innovative fundamental food-structure-property relationships to the design of foods for health, wellness, and pleasure,” as well as the Ministry of Education, Science, and Technological Development, Republic of Serbia (Project No. III46010).
Additional information
Funding
References
- Lozano-Vazquez, G.; Lobato-Calleros, C.; Escalona-Buendia, H.; Chavez, G.; Alvarez-Ramirez, J.; Vernon-Carter, E.J. Effect of the Weight Ratio of Alginate-Modified Tapioca Starch on the Physicochemical Properties and Release Kinetics of Chlorogenic Acid Containing Beads. Food Hydrocolloids 2015, 48, 301–311.
- Eiselt, P.; Yeh, J.; Latvala, R.K.; Shea, L.D.; Mooney, D.J. Porous Carriers for Biomedical Applications Based on Alginate Hydrogels. Biomaterials 2000, 21, 1921–1927.
- Pillay, V.; Fassihi, R.J. In Vitro Release Modulation from Crosslinked Pellets for Site-Specific Drug Delivery to the Gastrointestinal Tract: II. Physicochemical Characterization of Calcium-Alginate, Calcium-Pectinate and Calcium-Alginate-Pectinate Pellets. Journal of Controlled Release 1999, 59, 243–256.
- Belščak-Cvitanović, A.; Komes, D.; Karlović, S.; Djaković, S.; Špoljarić, I.; Mršić, G.; Ježek, D. Improving the Controlled Delivery Formulations of Caffeine in Alginate Hydrogel Beads Combined with Pectin, Carrageenan, Chitosan and Psyllium. Food Chemistry 2015, 167, 378–386.
- Jana, S.; Gandhi, A.; Sheet, S.; Sen, K.K. Metal Ion-Induced Alginate–Locust Bean Gum IPN Microspheres Forsustained Oral Delivery of Aceclofenac. International Journal of Biological Macromolecules 2015, 72, 47–53.
- Balanč, B.; Kalušević, A.; Drvenica, I.; Coelho, M.T.; Djordjević, V.; Alves, V.D.; Sousa, I.; Moldão-Martins, M.; Rakić, V.; Nedović, V.; Bugarski, B. Calcium–Alginate–Inulin Microbeads As Carriers for Aqueous Carqueja Extract. Journal of Food Science 2015, 81, 65–75.
- Cheow, W.S.; Kiew, T.Y.; Hadinoto, K. Controlled Release of Lactobacillus Rhamnosus Biofilm Probiotics from Alginate-Locust Bean Gum Microcapsules. Carbohydrate Polymers 2014, 103, 587–595.
- Manjanna, K.M.; Rajesh, K.S.; Shivakumar, B. Formulation and Optimization of Natural Polysaccharide Hydrogel Microbeads of Aceclofenac Sodium for Oral Controlled Drug Delivery. American Journal of the Medical Sciences 2013, 1, 18–20.
- Ong, W.-D.; Tey, B.-T.; Quek, S.Y.; Tang, S.-Y.; Chan, E.-S. Alginate-Based Emulsion Template Containing High Oil Loading Stabilized by Nonionic Surfactants. Journal of Food Science 2014, 80, 93–100.
- Wichchukit, S.; Oztop, M.H.; McCarthy, M.J.; McCarthy, K.L. Whey Protein/Alginate Beads As Carriers of a Bioactive Component. Food Hydrocolloids 2013, 33, 66–73.
- Totosaus, A.; Ariza-Ortega, T.D.J.; Perez-Chabela, M.D.L. Lactic Acid Bacteria Microencapsulation in Sodium Alginate and Other Gelling Hydrocolloids Mixtures. Journal of Food and Nutrition Research 2013, 52, 107–120.
- Schoebitz, M.; Simonin, H.; Poncelet, D. Starch Filler and Osmoprotectants Improve the Survival of Rhizobacteria in Dried Alginate Beads. Journal of Microencapsulation 2012, 29, 532–538.
- Fioramonti, S.A.; Perez, A.A.; Aríngoli, E.E.; Rubiolo, A.C.; Santiago, L.G. Design and Characterization of Soluble Biopolymer Complexes Produced by Electrostatic Self-Assembly of a Whey Protein Isolate and Sodium Alginate. Food Hydrocolloids 2013, 1, 1–8.
- Dufrene, B. Global Tea Consumption Remains Robust. Tea & Coffee Trade Journal 2012, 184, 24–30.
- Simutis, R.; Lübbert, A. Exploratory Analysis of Bioprocesses Using Artificial Neural Network-Based Methods. Biotechnology Progress 1997, 13, 479–487.
- Vlassides, S.; Ferrier, J.G.; Block, D.E. Using Historical Data for Bioprocess Optimization: Modeling Wine Characteristics Using Artificial Neural Networks and Archived Process Information. Biotechnology and Bioengineering 2001, 73, 55–68.
- Belščak-Cvitanović, A.; Stojanović, R.; Manojlović, V.; Komes, D.; Juranović Cindrić, I.; Nedović, V.; Bugarski, B. Encapsulation of Polyphenolic Antioxidants from Medicinal Plant Extracts in Alginate–Chitosan System Enhanced with Ascorbic Acid by Electrostatic Extrusion. Food Research International 2011, 44, 1094–1101.
- Lachman, J.; Hosnedl, V.; Pivec, V.; Orsák, M. Polyphenols in Cereals and Their Positive and Negative Role in Human and Animal Nutrition, Proceedings of Conference Cereals for Human Health and Preventive Nutrition, Brno, Czech Republic, July 7-11, 1998; Vaculová, K.; Ehrenbergerová, J.; Eds.; Agricultural Research Institute Kromeriz, Ltd., 1998; 118–125 pp.
- Maiti, S.; Dey, P.; Banik, A.; Sa, B.; Ray, S.; Kaity, S. Tailoring of Locust Bean Gum and Development of Hydrogel Beads for Controlled Oral Delivery of Glipizide. Drug Delivery 2010, 17, 288–300.
- Hermansson, A.-M.; Eriksson, E.; Jordansson, E. Effects of Potassium, Sodium and Calcium on the Microstructure and Rheological Behaviour of Kappa-Carrageenan Gels. Carbohydrate Polymers 1991, 16, 297–320.
- Zhu, J.-H.; Yang, X.-Q.; Ahmad, I.; Jiang, Y.; Wang, X.-Y.; Wu, L.-Y. Effect of Guar Gum on the Rheological, Thermal and Textural Properties of Soybean B-Conglycinin Gel. International Journal of Food Science & Technology 2009, 44, 1314–1322.
- Draget, K.I.; Gaserod, O.; Aune, I.; Andersen, P.O.; Storbakken, B.; Stokke, B.T.; Smidsrod, O. Effects of Molecular Weight and Elastic Segment Flexibility on Syneresis in Ca-Alginate Gel. Food Hydrocolloids 2001, 15, 485–490.
- Schrieber, R.; Gareis, H. Chapter 2. From Collagen to Gelatine. In Gelatine Handbook: Theory and Industrial Practice; Schrieber, R.; Gareis, H.; Eds.; Wiley-VCH GmbH & Co.: Weinheim, Germany, 2007.
- Pongjanyakul, T.; Puttipipatkhachorn, S. Xanthan-Alginate Composite Gel Beads: Molecular Interaction and in Vitro Characterization. International Journal of Pharmaceutics 2007, 331, 61–71.
- Sriamornsak, P.; Kennedy, P.A. Swelling and Diffusion Studies of Calcium Polysaccharide Gels Intended for Film Coating. International Journal of Pharmaceutics 2008, 358, 205–213.
- Patel, A.R.; Nijsse, J.; Velikov, K.P. Novel Polymer–Polyphenol Beads for Encapsulation And Microreactor Applications. Soft Matter 2011, 7, 4294–4301.
- Giunchedi, P.; Gavini, E.; Moretti, M.D.L.; Pirisino, G. Evaluation of Alginate Compressed Matrices As Prolonged Drug Delivery Systems. AAPS PharmSciTech 2000, 1, 19.
- Wanchoo, R.K.; Sharma, P.K. Viscometric Study on the Compatibility of Some Water-Soluble Polymer–Polymer Mixtures. European Polymer Journal 2003, 39, 1481–1490.
- Samiey, B.; Ashoori, F. Adsorptive Removal of Methylene Blue by Agar: Effects of NaCl and Ethanol. Chemistry Central Journal 2012, 6, 1–14.
- Basherr, I.A.; Hajmeer, M. Artificial Neural Networks: Fundamentals, Computing, Design, and Application. Journal of Microbiological Methods 2000, 43, 3–31.
- Di Scala, K.; Meschino, G.; Vega-Gálvez, A.; Lemus-Mondaca, R.; Roura, S.; Mascheroni, R. An Artificial Neural Network Model for Prediction of Quality Characteristics of Apples During Convective Dehydration. LWT–Food Science and Technology 2013, 33, 411–416.
- Carvalho, N.A.T.; Soares, J.G.; Porto Filho, R.M.; Gimenes, L.U.; Souza, D.C.; Nichi, M.; Sales, J.S.; Baruselli, P.S. Equine Chorionic Gonadotropin Improves the Efficacy of a Timed Artificial Insemination Protocol in Buffalo During the Nonbreeding Season. Theriogenology 2013, 79, 423–428.