ABSTRACT
In this study, the effect of gamma irradiation treatment on the secondary structure of the whole egg-white proteins was investigated. Liquid, frozen, and dried egg-white samples were subjected to gamma irradiation doses of 0, 1, 2, and 3 kGy. Protein secondary structures were determined by Attenuated Total Reflectance Fourier-Transform Infrared (ATR-FTIR) spectroscopy. In liquid egg-white samples, irradiation caused a conformational change from α-helix to disordered structure. Besides, a decrease in intermolecular β-sheet content was detected. On the other hand, no significant changes were observed in the secondary structure of frozen and dried egg-white samples. The significance of the results and their possible relationship to the viscosity decrease was also discussed. It was suggested that radiation-induced structural rearrangements may play a key role in the viscosity decrease.
Introduction
Egg, as a stand-alone product or as a food ingredient, is an important source of nutrition. Egg-white consists of approximately 40 different kinds of proteins such as ovalbumin, ovotransferrin, ovomucoid, ovomucin, avidin, and lysozyme.[Citation1] The effect of some treatment processes on physical, chemical, and functional properties of egg-white proteins has become an attractive and essential area of research.[Citation2–Citation11] Eggs contaminated with microorganisms have been a major problem for public health. Gamma radiation treatment is an alternative technology that can destroy harmful pathogens and extent shelf life of eggs and egg products.[Citation12] Food and Drug Administration (FDA) has approved irradiation of eggs at doses up to 3 kGy.
The irradiation treatment may affect the secondary structure of the egg-white proteins. Previously, the effect of gamma irradiation on the secondary structure of ovomucoid and ovalbumin solutions has been identified by circular dichroism (CD) spectroscopy.[Citation13] In that study, however, analyses have been performed on the dilute (0.02%) individual protein samples. So, the reported secondary structure profiles for each sample is probably not representative of the original egg-white sample, since the intermolecular interactions between the proteins may change the secondary structure profile of albumen. Moreover, since egg-white is a very concentrated solution of proteins in water, the effect of gamma radiation can cause different degrees of changes in the secondary structure profile.
In addition, it has been reported that irradiation can affect the functional properties of egg-white in a distinct way.[Citation14] For instance, irradiation induces a dramatic decrease in viscosity of the egg-white samples.[Citation15–Citation18] It has been suggested that the decrease in viscosity may be due to the breakdown of proteins. However, Ma et al.[Citation15] obtained only minor bands with polyacrylamide gel electrophoresis of irradiated egg-white proteins at doses up to 2.98 kGy. Besides, Hajós et al.[Citation19] studied the effect of irradiation on the polypeptide pattern of egg-whites and observed only slight changes regarding the molecular masses of protein zones and reported that cleavage of protein chains is not significant. Pinto et al.[Citation17] also reported only slight degradation of the higher molecular weight proteins with an irradiation dose of 5 kGy, however, no degradation of the major egg white protein. Although Song et al.[Citation18] explained the viscosity decrease of liquid egg-white samples by chain scission, in the previous study,[Citation13] the authors showed that the radiation effect was more significant at low protein concentration, with an increase of protein concentration the molecular weight profile of irradiated ovalbumin became close to non-irradiated control samples. In summary, these studies suggested that the degree of molecular weight profile change of the irradiated proteins was affected by protein concentration and absorbed dose. Low-dose radiation did not cause a significant change in the molecular weight of concentrated samples. However, a marked decrease in the viscosity of egg-white samples was observed, even after low-dose irradiation. As a result, another important factor, change in the secondary structure, can contribute to the decrease in viscosity of irradiated egg-white proteins.
Fourier-Transform Infrared (FTIR) spectroscopy is recognized as a valuable tool for the examination of protein conformation in H2O-based solution, as well as in deuterated forms and dried states, resulting in a greatly expanded use in studies of the protein secondary structure and protein dynamics.[Citation20–Citation25] The most sensitive spectral region to the protein secondary structural components is the amide I band (1700−1600 cm−1), which is due almost entirely to the C=O stretch vibrations of the peptide linkages. The frequencies of the amide I band components are found to be correlated closely with the each secondary structural element of the proteins.[Citation26] In our study, therefore, the effect of gamma irradiation on the secondary structure of real liquid, dried, and frozen egg-white samples was examined using Attenuated Total Reflectance Fourier-Transform Infrared (ATR-FTIR) spectroscopy. ATR accessory is simpler to use than the conventional transmission mode. All types of samples (e.g. solids, liquids, powders, pastes, pellets, slurries, fibers etc.) can be analyzed within a few seconds. In addition, the effect of secondary structural change on the viscosity of egg-white samples was discussed.
Materials and methods
Samples and gamma irradiation
Liquid and dried egg white samples were supplied from AB Foods Inc. (Balıkesir, Turkey). A certain amount of liquid egg samples were frozen immediately at –18±1 °C in a freezer (Beko 3400 CF, Turkey). All egg samples (liquid, frozen, and dried) were exposed to ionizing radiation using tote-box type irradiator equipped with 60Co gamma source (SVST-1 category IV) at Turkish Atomic Energy Authority, Sarayköy Nuclear Research and Training Center (Ankara, Turkey) at a dose rate of 1.5 kGy/h. Irradiation was performed at doses of 0, 1, 2, and 3 kGy at ambient temperature and atmosphere. The dose distribution was measured using a Harwell Amber 3042 dosimeter. Irradiated and non-irradiated liquid, frozen, and dried egg white samples were stored until analysis at +4 ± 1°C, −18 ± 1°C, and at room temperature, respectively.
FTIR measurements
Irradiated and non-irradiated liquid, frozen, and dried egg white samples were analyzed in a TENSOR 27 FTIR spectrometer (Bruker Optik GmbH, Ettlingen, Germany). Samples were placed on the PIKEMIRacle attenuated total reflectance (ATR) device (ZnSe crystal) and carefully pressed down to ensure a good contact with the ATR crystal. Spectra were recorded in the 4000-600 cm−1 infrared spectral range with 4.0 cm−1 resolution and for each measurement 128 scans were averaged. A minimum of three spectra was used for spectral analysis. Spectra of the samples were processed using the Bruker OPUS 6.5 software.
Spectral analysis and secondary structure estimation
The quantitative estimation of secondary structures of the proteins present in the egg-white samples was determined according to the frequency-based curve-fitting procedures described elsewhere.[Citation26–Citation29] According to these procedures, mathematical methods are necessary to resolve the individual band component corresponding to specific secondary structure.[Citation26] In this paper, in order to determine α-helix, β-sheet, β-turn, and disordered structures individually and carry out quantitative analysis of these secondary structures, the amide I region of the spectra (1600–1700 cm−1) were subjected to Fourier self-deconvolution (FSD) and curve fitting methods. FSD was performed with Lorentzian band shape, an enhancement factor of 1.3 and bandwidth of 15 cm−1. Deconvolution spectra were used to determine the number and the positions of the absorbance peaks located in the amide I region. These bands were used as starting parameters for the curve-fitting procedure. The relative proportions of secondary structures quantitatively estimated from the areas under the peaks centered at this absorption bands. The entire procedure was replicated three times and differences among means were tested using Duncan’s multiple range test, at a significant level of p < 0.05.
Viscosity measurements
A digital rotary viscometer (Sine-wave Vibro Viscometer SV-10, JAPAN) was used to determine the viscosity of irradiated and non-irradiated liquid egg-white samples. Samples were cooled to 4°C and all the viscosity measurements were performed at this temperature. Viscometer was calibrated before each measurement with purified water. The entire procedure was replicated three times and differences among means were tested using Duncan’s multiple range test, at a significant level of p < 0.05.
Results and discussion
FTIR spectroscopy is a valuable tool for the investigation of protein secondary structure. The amide I vibration depends on the secondary structure of the backbone and is therefore used for secondary structure analysis. shows the FSD spectra of non-irradiated liquid, dried and frozen egg-white samples. As can be seen, the amide I band was resolved into five component bands. Bands in the region of 1610−1700 cm−1 have been assigned to different type of secondary structures (α-helix, β-sheet, β-turn, and disordered conformations) by many authors.[Citation26,Citation30–Citation32] Other bands can arise from the side chain absorptions. Thus, the weak bands around 1607 cm−1 in all samples were assigned to side-chain absorption and the other four bands were used to evaluate the secondary structures. The assignments of the secondary structures reported in the literature[Citation32] and obtained in our study are presented in .
Table 1. Assignment of amide I band positions to the secondary structure for non-irradiated liquid, frozen, and dried egg-white samples.
Figure 1. Fourier self-deconvoluted amide I band of non-irradiated liquid, frozen and dried egg-white sample.
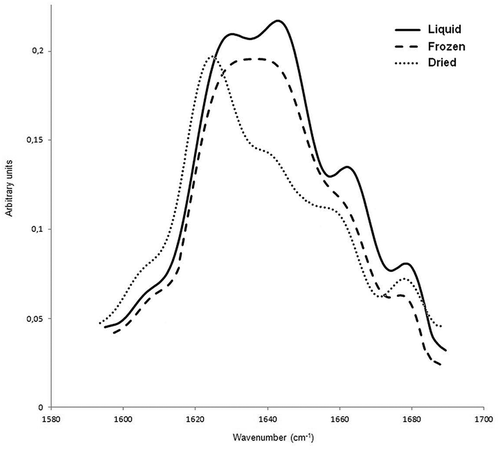
In the present study, curve fitting technique was used to determine the amounts of secondary structure content. However, only one peak was obtained in the region between 1642−1657 cm−1 (), which is associated with α-helical and disordered structures. The presence of more than one protein species in the samples may probably cause the overlap between two adjacent peaks. Moreover, the attempts to resolve the bands were unsuccessful, probably due to extensive overlapping. It has also been reported that the difficulty in resolving α-helices from random coils is one of the shortcomings of amide I IR absorption spectroscopy.[Citation25,Citation33] So, the results obtained from the α-helix bands were reported as the sum of the absolute amounts of α-helix + disordered structures. Beside the obtained percentages of secondary structures, band frequency shifts were also used to discuss the effect of freezing, drying and irradiation on secondary structures of egg-white samples.
As seen in , the difference between relative peak areas of α-helix + disordered structure in non-irradiated liquid and frozen samples was not significant, however, the band centered at 1645 cm−1 for liquid egg-white sample (0 kGy) was appeared at 1643 cm−1 for non-irradiated frozen sample ( and ). Although the two samples seem to have almost the same amount of α-helix + disordered structures, the shift in peak positions indicates a structural transition. A peak shift to lower wavenumber of a composite absorption can be produced by an increase in the intensity of a low-frequency component.[Citation34] Since the disordered conformation is the low-frequency component of this band, it has been suggested that freezing promote a structural transition from α-helical conformation to disordered structure due to the cold denaturation. In addition, the decrease in the α-helical structure with the concomitant increase in the disordered structure did not cause any change in the relative area of this band. On the other hand, same band was more shifted towards lower wavenumber (1642 cm−1) for the non-irradiated dried sample, indicating helix-coil transition was more pronounced in this sample. As can be seen in , percentage contribution of α-helix + disordered peak was decreased while the relative area of the low-frequency β-sheet was increased in dried sample (0 kGy) compared to the same peaks obtained from the non-irradiated liquid and frozen samples. Low-frequency β-sheet band is composed of two components; the intermolecular (1620–1630 cm−1) and intramolecular (1630–1640 cm−1) hydrogen-bonded β-sheet structures,[Citation32,Citation35] however, the high-frequency β-sheet is more useful in quantitative band analysis because it is separated from the other bands and integrated area of this peak is proportional only to the population of intramolecular β-sheet structures.[Citation36] As shown in , the high-frequency β-sheet content down to ~ 4% in dried sample (~6% in liquid sample), indicating a decrease in intramolecular β-sheet population. In addition, the peak shift observed for low-frequency β-sheet band in dried sample also give valuable information about the structural transition. This band was appeared at 1631 and 1627 cm−1 for non-irradiated liquid and dried egg-white samples, respectively. The shift to lower wavenumber indicates an increase in the intermolecular hydrogen-bonded β-sheet component and/or a decrease in the intramolecular β-sheet structures. The reduction in the high-frequency β-sheet band, as mentioned above, is indicative of the decrease in the intramolecular component. On the other hand, Ngarize et al.,[Citation37] reported that heat denaturation caused an increase in the intermolecular hydrogen-bonded β-sheet structures of heated ovalbumin due to the aggregation. So, the observed low-frequency band shift of the non-irradiated dried sample can be explained by the sum of both effects. In terms of the amount of the secondary structure, the obtained result is a substantial increase in the low-frequency β structure compared to the native conformation (liquid sample) due to aggregation. Another point that needs to be emphasized is that the total β-sheet content present in liquid egg-white sample was high (49%) compared to that in protein samples consist of only one species, for instance, Ngarize et al.,[Citation37] reported that native ovalbumin had 34% total β-sheet structure. It is an important result because it shows the behavior of the real sample. It can be suggested that protein-protein interactions cause this significant increase since the protein mixture in high concentration (real liquid egg-white) was used as sample in this study.
Table 2. Secondary structure percentages of non-irradiated and irradiated liquid, frozen, dried egg white samples.
In non-irradiated frozen samples, helix-coil transition was also observed, however, the low-frequency β-sheet band did not shift towards lower wavenumber, indicating cold denaturation did not give rise to the intermolecular β-sheet formation (, ). In addition, the percentages of the high-frequency β-sheet component in non-irradiated liquid and frozen samples are not significantly (p < 0.05) different. When compared to the liquid sample, there was an increase in β-turn content of the dried sample, whereas the frozen sample showed a decrease in this structure. These results suggest that the pathway of the cold denaturation is substantially different from that of the heat denaturation.
Band positions () and percentages of secondary structures () of the dried and frozen samples were not significantly (p < 0.05) change after irradiation up to 3 kGy. In liquid samples, however, the band assigned to α-helix + disordered structure (1645 cm−1, non-irradiated) was shifted toward lower wavenumber (1643 cm−1) after irradiation with a dose of 3 kGy, indicating a decrease in the α-helical structure with a concomitant increase in the disordered structure (). The radiation energy absorbed by the dried sample can break the hydrogen bonding network of the ordered structures, but free radicals are not present in high quantity due to low-dose irradiation. Dry samples hold practically no water, so polar radicals can not interact with water and/or radiolysis products of water, instead of this they undergo recombination to form regular structures again. In addition, it can be suggested that the mobility of radicals in dried samples is not fast enough to cause secondary structural transition. As a result, irradiation at low-dose did not cause significant changes in the secondary structure of the dried samples. A similar result was obtained for irradiated frozen samples. Radicals diffuse much more slowly in frozen samples than in liquid samples due to the lower temperature. They are trapped in the frozen material; tend to recombine to form the original substances.[38a] Therefore, the effect of low-dose radiation on the secondary structure of frozen sample was not significant.
Figure 2. Fourier self-deconvoluted amide I band of non-irradiated and irradiated (3 kGy) liquid egg-white sample.
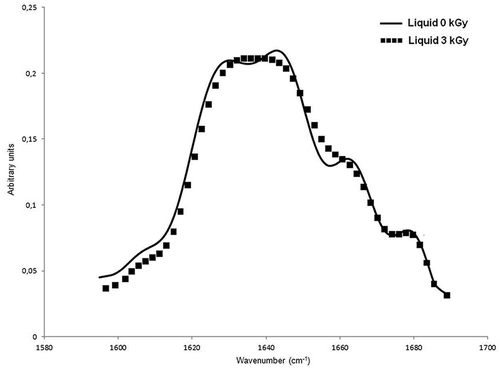
On the other hand, during irradiation of liquid samples, water absorbs large fraction of the radiation energy and peroxy and hydroxyl radicals are formed.[38b]. In particular, hydroxyl radicals can generate radical sites on the molecules and free radical mediated chain reactions can cause polypeptide chain scissions. These modifications in the primary structure of the proteins can give rise to distortions in the secondary structure. However, as mentioned in the introduction section, the effect of low-dose irradiation on the molecular weight profile of concentrated protein samples is less drastic. This result can be explained by the ratio of the amount of the proteins to the amount of radiolysis products of water. At low concentrations, the total number of target protein molecules is not large, therefore, the necessary amount of reactive radiolytic products of water for the degradation of the molecules will appear even after irradiation with low doses. In concentrated solutions, with the dose being same, same amount of radiolytic products of water is formed, but the protein content is much higher than the diluted solutions. Therefore, the number of reactive species will not sufficient to cause significant damage. In this study, liquid egg-white samples were directly irradiated without diluting them, so the protein concentration in liquid samples was extremely high (~10% or ~100 mg/ml). Thus, no significant change is expected in the primary structure of the liquid egg-white proteins. So, the reason of the observed helix-coil transition for liquid samples might be the radiation-induced cleavage of the hydrogen bonds that form the helix structure and/or destabilization of α-helical structure due to the reactive oxygen species, giving rise to an enhancement in disordered conformation. However, it should be noted that low-dose radiation caused partial unfolding; applied doses in this study probably could not supply the energy required to destabilize all existing hydrogen bonds, hence limited helix-coil transition was observed up to 3 kGy. This transition was proposed based on the shift of the helix-coil band in non-irradiated sample (1645 cm−1) to lower wavenumber (1643 cm−1) in irradiated sample at 3 kGy (, ). On the other hand, the band position of a low-frequency β-sheet in non-irradiated samples (1631 cm−1) shifted to a higher wavenumber after irradiation up to 3 kGy (1633 cm−1), suggesting a decrease in the intermolecular β-sheet and/or an increase in intramolecular β-sheet content. The decrease in the intermolecular β-sheet could be due to the hydration of the coils on the protein surface that cause reduction of protein-protein interaction. The formation of intramolecular β-sheet structures was ruled out since the percentage of high frequency β-sheet content did not change (). As can be seen in , the turn content of irradiated liquid sample remained unchanged after irradiation and this may be an explanation for maintaining the folded state of the egg-white proteins.
The results of viscosity measurements are given in . As can be seen, irradiated liquid egg-white samples show a decrease in viscosity with increasing doses of irradiation. Several authors have explained the decrease in viscosity of irradiated dilute egg-white proteins by peptide chain scission.[Citation15,Citation18,Citation39] As mentioned before, at low irradiation doses (below 2–3 kGy) the primary structure of proteins in a high concentration solution is expected to be stable or chain scissions would be very small in the dose range up to 3 kGy. However, we observed a significant decrease in the viscosity of whole liquid egg-white sample after irradiation even at a dose of 1 kGy. Two explanations can be postulated with respect to this observation: decrease in intermolecular interactions and dehydration of the protein core during irradiation. Galush et al.,[Citation40] showed that the high viscosity characteristic of high protein concentration solutions is caused by molecular crowding and direct interactions among proteins. Such interactions lead to the formation of long-range protein networks and result in high solution viscosity. For instance, it has been reported that viscosity decreases with aging as the ovomucin-lysozyme complex breaks down.[Citation41] Similarly, Panozzo et al.[Citation7] also described the viscosity decrease after a homogenization process at 0 MPa as the disruption of the weak and unstable network characterizing the large protein aggregates naturally occurring in the untreated egg-white. So, the observed decrease in intermolecular interaction of the irradiated liquid samples in this study may be the one of the reasons for the viscosity decrease in irradiated liquid egg-white samples. However, this decrease was obtained after irradiation at dose of 2 kGy. So, another reason, the radiolysis of buried water, may contribute to the viscosity decrease.
Table 3. Viscosity of non-irradiated and irradiated liquid egg-white samples.
The studies of buried water have revealed that cavities of protein interiors contain solvent molecules and these solvent molecules tend to form hydrogen bonds with main-chain polar atoms. It has been observed that buried water molecules appear preferentially near regions without regular secondary structures.[Citation42] Ionizing radiation can cause radiolytic decomposition of buried water and the core of the protein become more hydrophobic. It has been reported that buried water molecules in globular proteins exchange with bulk solvent on a nanosecond to microsecond timescale.[Citation43] According to this, after radiolysis of deeply buried water, transition of a new water molecule from the bulk is expected to occur in microseconds or less. However, formation a new intramolecular hydrogen bond between the polar R-groups of the polypeptide chain in the core will be much faster. However, these interactions could not be observed in the spectrum since they did not form a regular secondary structure. Besides, hydrophobic interactions between nonpolar R-groups in the protein interior can also be increased. Consequently, it could be suggested that radiolysis of the buried water molecules caused more hydrophobic environment in the interior of proteins, thereby packing interactions in the core collapsed the structure and lead to reduce the of the volume of the proteins. Proteins probably occupied a smaller spherical size after irradiation, and this may be other the reason that cause a considerable decrease in the viscosity of liquid egg-white samples. However, more studies are needed to confirm this suggestion.
Conclusion
For the first time (to our knowledge), the secondary structure distribution and freezing-, drying- and gamma radiation-induced changes in the secondary structure profile of whole egg-white proteins was determined by FTIR in this study. According to the results, the composite nature of egg-white showed different secondary structure profile compared to that of individual proteins in egg-white. Since the egg-white sample is a mixture of numerous proteins, contribution of intermolecular β-sheet component to the secondary structure profile was found to be high due to the protein-protein interactions. Both freezing- and drying-induced denaturation caused a helix-coil transition in non-irradiated samples. However, low-frequency β-sheet maxima was shifted to lower wavenumber only in dried sample, indicating heat denaturation gave rise to the intermolecular β-sheet formation due to aggregation and the pathway of the cold denaturation is substantially different from that of the heat denaturation. It was observed that gamma irradiation did not lead to complete unfolding and left a significant part of the proteins in a globular form. Moreover, irradiation gave rise to a decrease in intermolecular interactions and it was suggested that this might be a reason for the reduction of viscosity. Another reason to be considered might be the radiolysis of buried water, which caused more hydrophobic environment in the interior of proteins. In this environment probably the hydrogen bond donors and the acceptors of side chains were close enough together and the interaction became strong enough for them to form hydrogen bonds. Thus, this effect improved internal packing and reduced the volume of the proteins, resulting in a decrease in viscosity in the liquid egg-white samples.
Funding
The authors wish to express their gratitude to Turkish Atomic Energy Authority (TAEK-A3.H1.P1.01), which financially supported this work.
Additional information
Funding
References
- Yamamoto, T.; Juneja, L.R.; Hatta, H.; Kim, M. Hen Eggs: Basic and Applied Science; CRC Press: New York, 1997; 19–22 pp.
- Chang, C.; Niu, F.; Su, Y.; Qiu, Y.; Gu, L.; Yang, Y. Characteristics and Emulsifying Properties of Acid and Acid-Heat Induced Egg White Protein. Food Hydrocolloids 2016, 54, 342–350.
- Qian, J.Y.; Ma, L.J.; Wang, L.J.; Jiang, W. Effect of Pulsed Electric Field on Structural Properties of Protein in Solid State. LWT - Food Science and Technology 2016, 74, 331–337.
- Singh, A.; Sharma, M.; Ramaswamy, H.S. Effect of High Pressure Treatment on Rheological Characteristics of Egg Components. International Journal of Food Properties 2015, 18, 558–571.
- Lv, L.; Chi, Y.; Chen, C.; Xu, W. Structural and Functional Properties of Ovalbumin Glycated by Dry-Heating in the Presence of Maltodextrin. International Journal of Food Properties 2015, 18, 1326–1333.
- Zhu, S.; Zhou, M.; Yu, Y.; Li, J.; He, J. Coagulation of Egg White of Soft-Shell Turtle (pelodiscus sinensis) at High Pressure or High Temperature. International Journal of Food Properties 2014, 17, 2332–2343.
- Panozzo, A. Manzocco, L.; Calligaris, S.; Bartolomeoli, I.; Maifreni, M.; Lippe, G.; Nicoli, M.C. Effect of High Pressure Homogenisation on Microbial Inactivation, Protein Structure and Functionality of Egg White. Food Research International 2014, 62, 718–725.
- Onwulata, C.I.; Thomas-Gahring, A.E.; Phillips, J.G. Physical Properties of Mixed Dairy Food Proteins. International Journal of Food Properties 2014, 17, 2241–2262.
- Uzun, H.; Ibanoglu, E.; Catal, H.; Ibanoglu, S. Effects of Ozone on Functional Properties of Proteins. Food Chemistry 2012, 134, 647–654.
- Acero-Lopez, A.; Ullah, A.; Offengenden, M.; Jung, S.; Wu, J. Effect of High Pressure Treatment on Ovotransferrin. Food Chemistry 2012, 135, 2245–2252.
- Ahmed, J.; Ramaswamy, H.S.; Alli, I.; Raghavan, V.G.S. Protein Denaturation, Rheology, and Gelation Characteristics of Radio-Frequency Heated Egg White Dispersions. International Journal of Food Properties 2007, 10, 145–161.
- Crawford, L.M.; Ruff, E.H. A Review of the Safety of Cold Pasteurization Through Irradiation. Food Control 1996, 7, 87–97.
- Moon, S.; Song, K.B. Effect of Gamma-Irradiation on the Molecular Properties of Ovalbumin and Ovomucoid and Protection by Ascorbic Acid. Food Chemistry 2001, 74, 479–483.
- Campbell, L.; Raikos, V.; Euston, S.R. Modification of Functional Properties of Egg-White Proteins. Nahrung/Food 2003, 47, 369–376.
- Ma, C.Y.; Sahasrabudhe, M.R.; Poste, L.M.; Harwalkar, V.R.; Chambers, J.R. Gamma Irradiation of Shell Eggs. Internal and Sensory Quality, Physicochemical Characteristics, and Functional Properties. Canadian Institute of Food Science and Technology Journal 1990, 23, 226–232.
- Min, B.R.; Nam, K.C.; Lee, E.J.; Ko, G.Y.; Trampel, D.W.; Ahn, D.U. Effect of Irradiating Shell Eggs on Quality Attributes and Functional Properties of Yolk and White. Poultry Science 2005 84, 1791–1796.
- Pinto, P.; Ribeiro, R.; Sousa, L.; Cabo Verde, S.; Lima, M.G.; Dinis, M.; Santana, A.; Botelho, M.L. Sanitation of Chicken Eggs by Ionizing Radiation: Functional and Nutritional Assessment. Radiation Physics and Chemistry 2004, 77, 33–36.
- Song, H.P.; Kim, B.; Choe, J.H.; Jung, S.; Kim, K.S.; Kim, D.H.; Jo, C. Improvement of Foaming Ability of Egg White Product by Irradiation and Its Application. Radiation Physics and Chemistry 2009, 78, 217–221.
- Hajós, G.; Kiss, I.; Halasz, A. Chemical Changes of Proteins of Irradiated Egg White. Radiation Physics and Chemistry 1990, 36, 639–643.
- Singh, A.; Lahlali, R.; Vanga, S.K.; Karunakaran, C.; Orsat, V.; Raghavan, V. Effect of High Electric Field on Secondary Structure of Wheat Gluten. International Journal of Food Properties 2016, 19, 1217–1226.
- Vanga, S.K.; Singh, A.; Kalkan, F.; Gariepy, Y.; Orsat, V.; Raghavan, V. Effect of Thermal and High Electric Fields on Secondary Structure of Peanut Protein. International Journal of Food Properties 2016, 19, 1259–1271.
- Rafe, A.; Razavi, S.M.A. Effect of Thermal Treatment on Chemical Structure of β-Lactoglobulin and Basil Seed Gum Mixture at Different States by ATR-FTIR Spectroscopy. International Journal of Food Properties 2015, 18, 2652–2664.
- Long, G.; Ji, Y.; Pan, H.; Sun, Z.; Li, Y.; Qin, G. Characterization of Thermal Denaturation Structure and Morphology of Soy Glycinin by FTIR and SEM. International Journal of Food Properties 2015, 18, 763–774.
- Blume, K.; Dietrich, K.; Lilienthal, S.; Ternes, W.; Drotleff, A.M. Exploring the Relationship between Protein Secondary Structures, Temperature-Dependent Viscosities, and Technological Treatments in Egg Yolk and LDL by FTIR and Rheology. Food Chemistry 2015, 173, 584–593.
- Ulrichs, T.; Drotleff, A.M.; Ternes, W. Determination of Heat-Induced Changes in the Protein Secondary Structure of Reconstituted Livetins (Water-Soluble Proteins from Hen’s Egg Yolk) by FTIR. Food Chemistry 2015, 172, 909–920.
- Kong, J.; Yu, S. Fourier-Transform Infrared Spectroscopic Analysis of Protein Secondary Structures. Acta Biochimica et Biophysica Sinica 2007, 39, 549–559.
- Dong, A.; Huang, P.; Caughey, W.S. Protein Secondary Structures in Water from Second-Derivative Amide I Infrared Spectra. Biochemistry 1990, 29, 3303–3308.
- Georget, D.M.R.; Belton, P.S. Effects of Temperature and Water Content on the Secondary Structure of Wheat Gluten Studied by FTIR Spectroscopy. Biomacromolecules 2006, 7, 469–475.
- Litvinov, R.I.; Faizullin, D.A.; Zuev, Y.F.; Weisel, J.W. The α-Helix to β-Sheet Transition in Stretched and Compressed Hydrated Fibrin Clots. Biophysical Journal 2012, 103, 1020–1027.
- Jones, C. In Microscopy, Optical Spectroscopy, and Macroscopic Techniques; Jones, C.; Mulley, B.; Thomas, A.H. Eds.; Humana Press Inc.: New Jersey, 1994; 190 pp.
- Pelton, J.T.; McLean, L.R. Spectroscopic Methods for Analysis of Protein Secondary Structure. Analytical Biochemistry 2000, 277, 167–176.
- Barth, A. Infrared Spectroscopy of Proteins. Biochimica et Biophysica Acta 2007, 1767, 1073–1101.
- Fayer, M.D. Ultrafast Infrared Vibrational Spectroscopy; CRC Press: New York, 2013; 367 pp.
- Jackson, M.; Mantsch, H.H. The Use and Misuse of FTIR Spectroscopy in Determination of Protein Structure. Critical Reviews in Biochemistry and Molecular Biology 1995, 30, 95–120.
- Avis, K.E.; Wu, V.L. Biotechnology and Biopharmaceutical Manufacturing, Processing, and Preservation; CRC Press: New York, 1996; 233 pp.
- Wang, T.; Xu, Y.; Du, D.; Gai, F. Determining Beta-Sheet Stability by Fourier Transform Infrared Difference Spectra. Biopolymers 2004, 75,163–172.
- Ngarize, S.; Herman, H.; Adams, A.; Howell, N. Comparison of Changes in the Secondary Structure of Unheated, Heated, and High-Pressure-Treated Beta-Lactoglobulin and Ovalbumin Proteins Using Fourier Transform Raman Spectroscopy and Self-Deconvolution. Journal of Agricultural and Food Chemistry 2004, 52, 6470–6477.
- Woods, R.J.; Pikaev, A.K. Applied Radiation Chemistry Radiation Processing; Wiley Press: New York, 1994; 446–447 pp.
- Woods, R.J.; Pikaev, A.K. Applied Radiation Chemistry Radiation Processing; Wiley Press: New York, 1994; 165 pp.
- Liu, X.D.; Han, R.X.; Yun, H.; Jung, K.C.; Jin, D.I.; Lee, B.D.; Min, T.S.; Jo, C. Effect of irradiation on foaming properties of egg white proteins. Poultry Science 2009, 88, 2435–2441.
- Galush, W.J.; Le, L.N.; Moore, J.M.R. Viscosity behavior of high-concentration protein mixtures. Journal of Pharmaceutical Sciences 2012, 101, 1012–1020.
- Regenstein, J.M.; Regenstein, C.E. Food Protein Chemistry: An Introduction for Food Scientists; Academic Press Inc: Orlando, 1984; 46 pp.
- Park, S.; Saven, J.G. Statistical and Molecular Dynamics Studies of Buried Waters in Globular Proteins. Proteins 2005, 60, 450–463.
- Mattos, C. Protein-Water Interactions in a Dynamic World. Trends in Biochemical Sciences 2002, 27, 203–208.