ABSTRACT
A set of protein-stabilised emulsions at pH 7.0, pH 6.0, and pH 5.0, and their counterpart surfactant emulsions, was designed with near-identical droplet size distribution and phase volume to study the specific contribution of hydrodynamic and pair potential interactions to the interfacial mechanisms of these emulsions systems. In this way, further the interfacial layer of these creaming emulsions to enhance perceived fat content could be manipulated. Creaming behaviour, surface shear, and bulk rheological measurements were performed. This work reflects the great importance of local pair potential in the formation of a highly viscoelastic interfacial film, which could be manipulated changing the surface charge of the protein to develop a well-packed cream layer in the protein-stabilised emulsions.
Introduction
Obesity and diet-related disease is an increasing problem in the developed world. A significant proportion of dietary fat intake is in the form of food emulsions (sauces, dressing, creams, dairy and baked products). The sensory perception of fat in food is a complex function of physical, chemical, and even psychological parameters.[Citation1] By gaining a more complete understanding of how the physical properties of interfaces can control the structure and sensory properties of emulsions, we can develop strategies for reducing fat content with minimal impact on sensory acceptability.
Proteins and surfactants stabilise interfaces in very different ways. Proteins are complex macromolecules with different levels of structure that form an immobile viscoelastic adsorbed layer on the surfaces of oil droplets, which mechanically prevent coalescence. In contrast, surfactants tend to form more fluid adsorbed layers, which stabilise the interface at short range through a hydrodynamic mechanism known as the Gibbs–Marangoni mechanism, which relies on the mobility of the adsorbed molecules to maintain interfacial equilibrium.[Citation2] Moore et al.[Citation3] observed that changes in interfacial composition influenced the sensory perception of creaminess and also the emulsion rheological behaviour elaborated with two proteins: sodium caseinate and whey protein. However, the mechanism(s) underlying this phenomenon were not very clear but could be related to the different mechanisms that stabilise interfaces between proteins and surfactants.[Citation4] Therefore, a fundamental understanding of them would allow strategies to be developed to improve the texture and behaviour of food colloids maintaining their sensory properties whilst reducing the fat content.
Using this strategy, Mackie et al.[Citation4] created two emulsion systems as identical as possible in size and long-range interactions but with very different interfacial compositions. One emulsion was stabilised by a protein (whey protein isolate (WPI)), possessing a rigid, viscoelastic interface, and the other one was stabilised by a surfactant possessing a fluid interface. Significant differences in the bulk rheology and creaming behaviour were observed, mainly due to two contributing factors: the hydrodynamic interactions, which might explain the differences observed in the interfacial rheology, and the close-range interactions between the layers of proteins, which might explain the degree of adhesion observed in this system. The protein-stabilised emulsions appeared to slow or arrest the packing within the cream, leading to the formation of a lower density network because of their immobile, viscoelastic interfaces. However, the surfactant system showed a quicker rearrangement and a well-packed cream layer because of its mobile, fluid interfaces, which have little effect on the velocity of the solvent close to the interfaces.
However, it was not possible to quantify the specific contribution of these short-range interactions (hydrodynamic and pair potential interactions) to the emulsion rheology and structure. In order to determine the contribution of these parameters (the nature of the interface, the long- and close-range interactions) to the rheological behaviour of creaming emulsions, a set of emulsion systems based on the original work of Mackie et al. was designed.[Citation4]
For this purpose, first a set of protein-stabilised emulsions at different pH values (identical interfacial composition and near-similar droplet size distribution but with different long-range interactions) was designed to study how the local distribution charge on droplets is influenced by changing the net charge. After that, these protein interfaces were disrupted and displaced by various types of low molecular weight (LMW) surfactants (sodium dodecyl sulphate (SDS), Brij 35, and a mixture of Brij 35/SDS) to modify the composition of the interfaces. The creaming behaviour and bulk and interfacial rheology of these model systems were examined in order to understand the generic interfacial mechanisms controlling the physical structure and breakdown of these emulsion systems.
Materials and methods
Materials
n-tetradecane, SDS, and Brij 35 were purchased from the Sigma Chemical
Company (St Louis, MO, USA). WPI (86% β lactoglobulin) was purchased from Bipro (Davisco Foods International Inc., MN).
Emulsion systems’ preparation
Five protein-stabilised emulsions were made up from a stock oil-in-water emulsion with a dispersed phase content of 30% (w/w) of n-tetradecane as the dispersed phase and 0.455% (w/v) of WPI (86% β-lactoglobulin) (Bipro, Davisco Foods International Inc., MN) in a 10 mM citrate buffer at pH 6.0 as the continuous phase. This single parent emulsion was homogenised with a homogeniser (Vortmix, Hampton, UK), using three shearing cycles of 30 s with periods of rest of 30 s in between. The particle size distribution of this protein stabilised emulsion was measured (). Afterwards, this stock emulsion was diluted with concentrated buffers at different pH values, above and below the isoelectric point (IEP) (4.5) of WPI (0.1 M phosphate buffer for pH 7.0 and 0.1 M citrate buffer for pH 5.0, pH 4.0, and pH 3.0) to give the final emulsions at 20% (w/w) n-tetradecane and 0.3% WPI (w/v).
Table 1. Droplet size distribution and zeta potential measurements for the emulsions systems (protein-and surfactant-stabilised emulsions) studied.
Subsequently, three surfactant-stabilised emulsions were also made up from the stock oil-in-water emulsion defined above as shown in Fig. S1. For each surfactant emulsion, 66 g of this stock emulsion was diluted with 24 g 0.1 M sodium citrate buffer (pH 6.0) and then 9 g of a “surfactant solution” was added, stirring the resulting solution for 20 min. From previous measurements, we know that this surfactant concentration was sufficient to displace the protein from the oil droplet surface. The data in show that the reduction in interfacial tension induced by the surfactant was 10 mN/m. This difference has been shown in the past to be sufficient to induce displacement of protein from an interface by surfactant.[Citation5] The final continuous phase buffer concentration was 10 mM. The surfactant solution was specific for each final emulsion in order to imitate the net charge of the protein-stabilised emulsions (). The LMW surfactants used were SDS (Sigma-Aldrich, Poole, UK), which is an ionic surfactant, polyoxyethylene 23 lauryl ether (Brij 35), which is a non-ionic surfactant, as well as a mixture of both (Brij 35/SDS) at a 2:3 ratio. Therefore, the concentrations of the final surfactant-stabilised emulsions were: 20% n-tetradecane and 0.3% WPI, as well as 0.32% surfactant (SDS), 0.81% surfactant (Brij 35) or 0.25% surfactant (Brij 35/SDS). Droplet size measurements at the end of the experiments showed no change from the initial values.
Emulsion systems’ characterisation
The droplet size distribution of the emulsions was measured using a Coulter LS 230 laser diffraction particle size analyser (Beckman Coulter Inc., CA) to ensure that all the emulsion systems prepared had a uniform average droplet diameter (D3,2) (), and the data were analysed using an optical model for a fluid with real parts of the complex refractive index set to 1.332 and 1.391 for the continuous and dispersed phases, respectively. The ξ potential of the emulsions was measured using a Zetasizer Nano model ZEN3600 (Malvern Instruments, UK) and calibrated using the –50 mV standards supplied by Malvern. All samples were measured having been diluted using the relevant continuous phase (separated by centrifugation). The interfacial tension between the aqueous phase of the emulsion and n-tetradecane was measured using the pendant drop technique.[Citation6]
Creaming
The creaming behaviour was examined by visually measuring the height of a layer formed in a 75-mm-high measuring cylinder filled with emulsion, and also by the technique of ultrasound velocity scanning. These measurements were made using an Acoustican system (Leeds University, UK).[Citation7] Readings were taken every 2 mm over the entire height of the emulsion to give a profile of the dispersed phase volume throughout the emulsion. Measurements continued until the majority of the oil was in the cream layer and the distribution was approaching equilibrium. All measurements were made at 20°C.
Rheological measurements
Surface shear and bulk rheological measurements utilised a TA Instruments AR2000 controlled stress rheometer (TA Instruments Ltd., Crawley, UK) in controlled strain mode. In situ rheological measurements during creaming used a modified cup and bob arrangement (depth of cup 150 mm, height of inner cylinder 40 mm). With this arrangement, we are able to monitor the bulk rheology of a sample at a range of heights within a creaming emulsion. The cylinder was placed near the top of the cup to monitor the development of the creamed phase or towards the bottom of the cup to monitor the serum phase. A measuring frequency of 1 Hz and a strain of 1% were chosen as the measuring conditions, and the samples were monitored over the same time scale as the ultrasonic creaming measurements. Rheological measurements of the separated cream were carried out using a parallel plate configuration in either steady or dynamic mode as required. Surface rheological measurements of the continuous phase at the oil-in-water interface were carried out with a polished aluminium bicone (6°, 60 mm diameter) placed at the interface, over a 1 h time period, again using a strain of 1% and at a frequency of 0.5 Hz. Dilatational rheological measurements were conducted using the oscillating pendant drop method[Citation8] at room temperature and 0.1 Hz with a deformation of less than 4%.
Results and discussion
Emulsion systems’ characterisation
As described above, a set of emulsions was designed from a single parent protein emulsion being near identical in terms of droplet size distribution, phase volume, and surface charge (), but differing in their surface composition, such that some were stabilised by a viscoelastic protein film and others by a fluid surfactant interface to study the specific contribution of the short-range interactions (hydrodynamic and pair potential interactions) to the rheological behaviour of these emulsions systems.
Initially, the WPI emulsion systems at pH 7.0, pH 6.0, and pH 5.0 showed a monomodal distribution with a large and narrow peak (data not shown). As can be seen in , these protein-stabilised emulsions at pH 7.0, pH 6.0, and pH 5.0 showed a near-identical D3,2 parameter (around 4.2 μm) but with different net charges (ξ potential values). The interface of the parent protein emulsion at pH 6.0 was disrupted and replaced by surfactants (SDS, Brij 35, and a mixture of both SDS/Brij 35) to match the “ζ” potential of the corresponding protein-stabilised emulsions (at pH 7.0, pH 6.0, and pH 5.0, respectively). These surfactant systems showed a near-identical droplet size distribution (around 4.5 μm) and a net charge (ξ potential) very similar to their corresponding protein systems (). However, protein emulsions at pH 4.0 and pH 3.0 showed a broad droplet size distribution (data not shown) with a larger D3,2 parameter (7–8 μm), which could be indicating the presence of large flocs because of aggregation of droplets.
Creaming
shows the creaming profiles of the emulsion systems stabilised by WPI at pH 7.0 (), pH 6.0 (), and pH 5.0 (), together with their respective emulsion counterparts stabilised by surfactants: SDS (), Brij 35/SDS (), and Brij 35 (). The emulsions shown in and , and , and and have the same net charge. The results are shown in terms of the dispersed phase volume as a function of height for a series of time intervals over a period of 48 h.
Figure 1. Dispersed phase volume as a function of height within protein-stabilised emulsions at pH 7.0 (a), pH 6.0 (c), and pH 5.0 (e) and within surfactant-stabilised emulsions with SDS (b), Brij 35/SDS (40:60) (d), and Brij 35 (f). Each line represents a set of measurements taken 60 min apart over 40 h. The arrows indicate the progression with time.
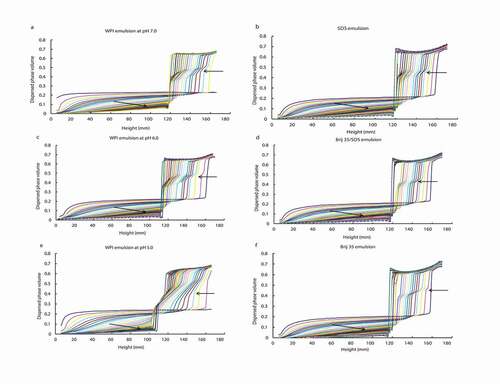
In all these systems, the development of a cream layer (high dispersed phase volume) could be observed at the top of the tube, which gradually increased in depth with time as the droplets migrated upwards, leaving a clear serum layer at the base of the tube. The lack of a sharp boundary at the base of the creaming emulsion indicates a broad size distribution and a lack of flocculation. Because the size distributions of both emulsions were identical, the oil droplets floated upwards at identical rates in both emulsions. These observations were in agreement with those reported by Mackie et al.[Citation4] The differences among the diverse emulsion systems were found in a pseudoplateau region in the cream layer itself. Thus, the pseudoplateau was more evident in WPI systems (, , and ) than in surfactant ones (, , and ), and was even more marked as the pH decreased. This pseudoplateau might be due to droplet interactions, delaying, or possibly arresting, the rearrangement into a packed cream layer, as suggested previously.[Citation4]
Unlike these WPI systems, in the surfactant-stabilised emulsions the creaming profiles were all very similar, reflecting that these systems were denser, with a similar well-packed cream layer, than their respective protein-stabilised emulsion counterparts at the same local charge. Moreover, in these latter systems the pseudoplateau disappeared much earlier than in the corresponding WPI emulsions, in which it disappeared almost at the end of creaming in the case of WPI at pH 7.0 and pH 6.0 ( and , respectively), and it even continued to develop at the end of creaming in the case of pH 5.0 (). In the protein-stabilised systems, the maximum phase volume at the top of the cream was reached very early, in around 5 h. Therefore, the different local charge distribution does not seem to influence the hydrodynamic interactions present at the interfaces. Thus, the surfactant-stabilised systems showed a fraction with faster and better packing than the protein-stabilised systems, as has also been reported in emulsions stabilised by protein and LMW surfactants.[Citation4] One reason to explain the presence of the pseudoplateau in the surfactant-stabilised systems could be that there might still be small amounts of protein at the interface. Another reason could be that other types of interactions at local level could be formed besides the ones between the surfactant headgroup and water molecules in the aqueous phase.[Citation2]
A second difference to note is that there were no significant differences among the creaming profiles of the surfactant-stabilised systems, all of which showed a similar velocity of the solvent close to the interface. This is because a fluid interface has little effect on the solvent velocity close to the interface, unlike a highly viscoelastic interfacial layer.[Citation4] Therefore, the hydrodynamic interactions between the molecules of the surfactant at the interface and the solvent do not seem to be influenced by the net local surface charge.
shows the dispersed phase volume contour plots of height against time for all the emulsions. The contours represent lines of equal oil phase volume starting at 2% (lowest y values) and increasing in 2% intervals up to around 60% depending on the final phase volume at the top of the cream layer. As can be observed, there was no aggregation among the droplets in the subcream region either in the WPI systems at pH 7.0, pH 6.0, and pH 5.0 (, , and , respectively) or in the surfactant-stabilised systems (, , and ), which is reflected by the linearity of the contour lines below the cream layer. Therefore, the droplets rise to the cream layer with fixed velocities. In contrast, the contours within the protein emulsions at low pH (3.0 and 4.0) again showed coalescence as could be expected (data not shown). As can be seen in , the pseudoplateau at the base of the cream layer in the WPI system at pH 5.0 () was more noticeable (it has the maximum depth) than in the emulsions at pH 7.0 and pH 6.0 ( and , respectively). However, in the three surfactant-stabilised systems this pseudoplateau at the base of the cream layer was almost negligible (, , and ), indicating a rearrangement into a well-packed, concentrated cream layer. Therefore, in the development of the cream layer in the protein-stabilised systems the adhesive (sticky) interactions at close range are more important than the hydrodynamic interactions, and they are dependent on the conformational state of the protein, which at the same time depends on its net charge.
Figure 2. Dispersed phase volume contour plots of height as a function of time for protein-stabilised emulsions at pH 7.0 (a), pH 6.0 (c), and pH 5.0 (e) and surfactant-stabilised emulsions with SDS (b), Brij 35/SDS (40:60) (d), and Brij 35 (f).
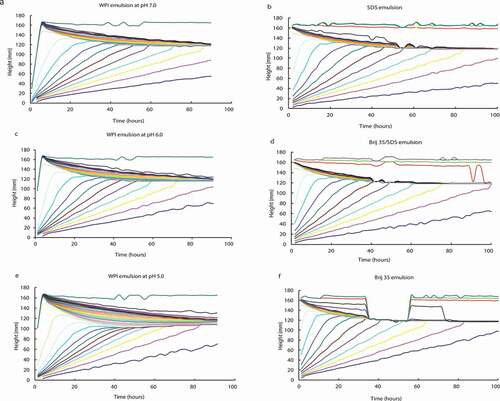
These close-range “adhesive” interactions were responsible of the delaying, or possibly arresting, the rearrangement into a packed cream layer being more remarkable at pH values near IEP (4.5) because the electrostatic interactions among the protein-covered droplets are negligible.[Citation9,Citation10] Thus, the sample at pH 5 showed a less dense cream layer than the samples at pH 7 and pH 6, probably because the formation of a higher number of “sticky” interactions () prevented rearrangement into a well-packed, concentrated layer. In the case of pH values that are higher from the IEP, the formation of these intermolecular bonds is not possible, because the electrostatic repulsion between the droplets prevents the close approach of the adsorbed layers.[Citation10]
Rheological measurements
The interactions between emulsion droplets in the dense creamed layer were studied by using rheological measurements of these layers. shows the bulk storage (G′) and loss (G″) moduli of the cream layers as they developed for the emulsion systems at different surface charge, that is, for the protein-stabilised emulsions at pH 7.0 (), pH 6.0 (), and pH 5.0 () and their counterpart surfactant-stabilised emulsions (SDS, SDS/Brij35, and Brij35, respectively). These measurements were carried out on duplicates of the samples monitored in and and were made for 48 h at a frequency of 1 Hz and 1% strain. The six emulsion systems showed a cream layer that was almost completely developed at 30 h (–3c) and in all them G′ was greater than G″ during the structure development in the creamed region, and it increased with time, which means that the number and/or strength of interactions increased with time too. This rheological response is the characteristic of solid-like materials. Nevertheless, the protein-stabilised emulsion showed much higher values of the elastic and viscous moduli than their respective surfactant-stabilised emulsion in all cases (–3c), in which G′ was more than one order of magnitude greater than G″ which means these protein emulsions were higher elastic systems than surfactants. It should be noted that in samples at pH 5.0 both moduli, G′ and G″ (), were considerably greater than in the sample at pH 7.0 and pH 6.0. This means that the emulsions at pH 5.0 were more rigid systems than the emulsion at pH 7.0. The values of the G′ and G″ moduli after the creaming layer had developed for WPI at pH 7.0 and pH 6.0 were similar to those found by Mackie et al.[Citation4] for WPI-stabilised system (around 1000 Pa). In these cases, the protein has the ability to form a viscoelastic adsorbed layer around the droplets,[Citation11] resulting in more elastic systems. In contrast, surfactants form a highly fluid or mobile interface stabilising emulsion through the Gibbs–Marangoni mechanism.[Citation2] On the other hand, in the surfactant systems the difference between the two moduli (G′ and G″) was smaller,[Citation4] the G′ values being almost identical in all three systems.
Figure 3. Bulk storage (large square symbols) and loss (small square symbols) moduli for creaming emulsions stabilised with WPI at pH 7.0 (a), pH 6.0 (b), and pH 5.0 (c) and with their counterpart surfactant emulsions: SDS (a), Brij 35/SDS (b), and Brij 35 (c).
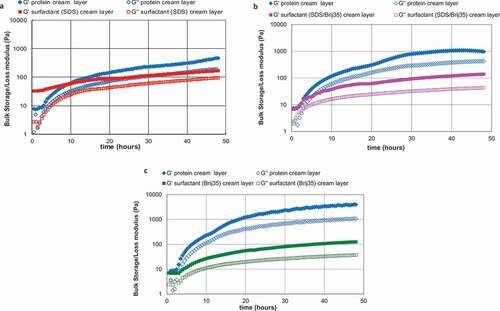
The G′ values for the different emulsions in are very similar to those presented in , which shows the storage modulus (G′) and loss modulus (G″) values as a function of applied strain of the final creamed phase of protein-stabilised emulsions at pH 7.0, pH 6.0, and pH 5.0 (–) and of their counterpart surfactant-stabilised emulsions (–). In the case of the WPI systems, the emulsion at pH 5.0 showed the highest G′ value (> 1000 Pa) () followed by the WPI emulsions at pH 6.0 (around 1000 Pa) () and at pH 7.0 (< 1000 Pa) (), in that order. The WPI emulsion at pH 5.0 showed a crossover of G′ and G″ at a lower strain value (around 0.03%) than its correspondent surfactant emulsion, Brij35 (around 0.05%) (). However, in the samples at pH 7.0 and pH 6.0 the opposite was true showing the surfactant-stabilised emulsions a crossover of G′ and G″ at a lower strain value (around 0.03%) than the protein systems (around 0.05%). The crossover point defines whether the nature of the sample is more fluid-like or gel-like.[Citation12] This could mean that the cream layer at pH 5.0 was stiffer and, at the same time, more brittle than those at pH 7.0 and pH 6.0, and it could easily be broken, allowing drop-drop coalescence as a result of small mechanical disturbances of the emulsion. This result is in accordance with the previous observations of and , and it is a consequence of slowing or arresting the packing within the cream leading not well-packed cream layer as discussed above. Therefore, the emulsion at pH 5 (near the IEP of WPI) seems to show a rigid, brittle cream layer.
Figure 4. Storage modulus (filled symbols) and loss modulus (empty symbols) for protein-stabilised emulsions at pH 7.0, pH 6.0, and pH 5.0 and their counterpart surfactant-stabilised emulsion cream layers plotted as a function of strain (%) at a frequency of 1 Hz.
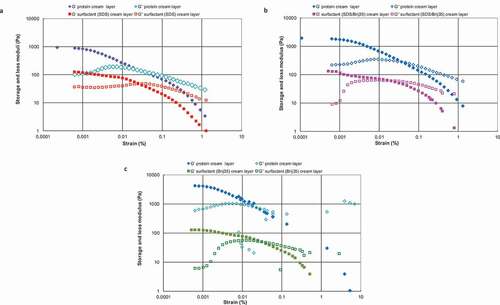
These observations are also consistent with the findings obtained by Tcholakova et al.[Citation10] These authors studied how β-lactoglobulin (BLG) adsorption on the drop surface changed by varying the protein and electrolyte concentrations and the pH of the aqueous phase, finding that the absorbed protein layer formed at pH ~ 5 showed a different structure from those formed from charged protein molecules (further away from the IEP). The layer formed at pH 5 was a fragile and relatively rigid layer which ruptured easily, resulting in minimal emulsion stability. Dimitrova et al.[Citation13] also suggested that in protein-stabilised emulsions at a pH close to the IEP the interfacial adsorption layers are stiffer, so the conformation of the protein is strongly pH-dependent. Because the WPI studied here contains 86% of BLG, it would be logical for it to show a similar behaviour. We could check this by measuring the rheological properties of the emulsion systems.
It is clear that the protein adsorption process involves a partial unfolding and subsequently a conformational rearrangement of the protein,[Citation14,Citation15] allowing the formation of close-range interactions among neighbouring molecules.[Citation16] The pH seems to influence the conformational structure of the adsorbed protein at the interface. In our experiment (), the protein-stabilised emulsions at a pH close to the IEP (~ 5) also show more rigid and brittle behaviour in which the adsorbed molecules are in a different conformational state that facilitates the formation of sticky interactions at close range or other types of interactions, such as hydrogen bonds between the same or different layers. In contrast, at pH values further away from the IEP the conformational state of WPI allows the formation of repulsive electrostatic interactions which stabilise the cream network. Thus, the interfaces of the protein-stabilised systems at pH 6 and pH 7 seem to be more resistant and stable than those at pH 5.
The next step was to look for the mechanism underlying these differences in the interfaces. In turn, and show the interfacial shear and dilatation storage moduli, respectively, for the protein and surfactant serum phases at the n-tetradecane/solution interface. The higher values of both interfacial shear and dilatation storage moduli in protein systems with respect to surfactants indicate their different interfaces mechanisms corroborating the observations found from the creaming and bulk rheology. Likewise, the lower value of interfacial shear storage modulus for serum phase at pH 5.0 than at pH 7.0 and pH 6.0 also reflects the differences found in their interfaces. The difference in the interfacial tension between the protein and surfactant systems will also have had an influence on the droplet deformability.[Citation4] On the other hand, the interfacial shear storage modulus values for the surfactant-stabilised emulsions were too small (negative values), and it was not even possible to obtain a value for the Brij 35 system.
Conclusion
The differences found in the creaming and rheological behaviour of protein- and surfactant-stabilised emulsions are mainly due to the different close-range interactions between the droplets at the interface. These close-range interactions were influenced by the local distribution charge in the case of protein-stabilised emulsions. The pH affects the conformational structure of the WPI at the interface, and consequently the number of local “sticky” intermolecular interactions, resulting in more open or closed cream structures. As a result, it seems that at a pH near the IEP of the protein (pH 5.0) a higher number of “sticky” intermolecular interactions can be formed, resulting in a stiffer, more brittle interface than at pH values further away from the IEP (pH 7.0 and pH 6.0), which produce less elastic and less stable interfaces and, at the same time, more open structures. Therefore, it seems that these intermolecular interactions have a direct effect on the viscoelastic nature of the interfacial film. In contrast, surfactant-stabilised emulsions showed a well-packed, concentrated cream layer and did not seem to be affective by the local surface charge distribution. Therefore, it would be possible to “manipulate” the development of the cream layer formed in protein-stabilised emulsions changing the surface charge of the protein. More studies are necessary, such as confocal microscopy, for further study of the structure of these emulsions.
Additional information
Funding
References
- Akhtar, M.; Stenzel, J.; Murray, B. S.; Dickinson, E. Factors Affecting the Perception of Creaminess of Oil-In-Water Emulsions. Food Hydrocolloids 2005, 19, 521–526. DOI: 10.1016/j.foodhyd.2004.10.017.
- Mackie, A.; Wilde, P. The Role of Interactions in Defining the Structure of Mixed Protein-Surfactant Interfaces. Advances in Colloid and Interface Science 2005, 117, 3–13. DOI: 10.1016/j.cis.2005.04.002.
- Moore, P. B.; Langley, K.; Wilde, P. J.; Fillery-Travis, A.; Mela, D. J. Effect of Emulsifier Type on Sensory Properties of Oil-In-Water Emulsions. Journal of the Science of Food and Agriculture 1998, 76, 469–476. DOI: 10.1002/(ISSN)1097-0010.
- Mackie, A. R.; Ridout, M. J.; Moates, G.; Husband, F. A.; Wilde, P. J. Effect of the Interfacial Layer Composition on the Properties of Emulsion Creams. Journal of Agricultural and Food Chemistry 2007, 55, 5611–5619. DOI: 10.1021/jf0636925.
- Chen, J. S.; Dickinson, E. Time-Dependent Competitive Adsorption of Milk-Proteins and Surfactants in Oil-In-Water Emulsions. Journal of the Science of Food and Agriculture 1993, 62, 283–289. DOI: 10.1002/(ISSN)1097-0010.
- Ambwani, D. S.; Fort, T. Pendant Drop Technique for Measuring Liquid Boundary Tensions. In Surface and Colloid Science (Vol II); Good, R. J.; Stromberg, R. R.; Eds.; Plenum: New York, 1979.
- Nelson, P. V.; Povey, M. J. W.; Wang, Y. T. An Ultrasound Velocity and Attenuation Scanner for Viewing the Temporal Evolution of a Dispersed Phase in Fluids. Review of Scientific Instruments 2001, 72, 4234–4241. DOI: 10.1063/1.1408936.
- Myrvold, M.; Hansen, H. Surface Elasticity and Viscosity from Oscillating Bubbles Measured by Automatic Axisymmetric Drop Shape Analysis. Journal of Colloid and Interface Science 1998, 207(1), 97–105. DOI: 10.1006/jcis.1998.5745.
- Valle-Delgado, J. J.; Molina-Bolivar, J. A.; Galisteo-Gonzalez, F.; Galvez-Ruiz, M. J.; Feiler, A.; Rutland, M. W. Interaction Forces between BSA Layers Adsorbed on Silica Surfaces Measured with an Atomic Force Microscope. Journal of Physical Chemistry B 2004, 108, 5365–5371. DOI: 10.1021/jp0374197.
- Tcholakova, S.; Denkov, N. D.; Ivanov, I. B.; Campbell, B. Coalescence Stability of Emulsions Containing Globular Milk Proteins. Advances in Colloid and Interface Science 2006, 123–126, 259–293. DOI: 10.1016/j.cis.2006.05.021.
- Wilde, P.; Mackie, A.; Husband, F.; Gunning, P.; Morris, V. Proteins and Emulsifiers at Liquid Interfaces. Advances in Colloid and Interface Science 2004, 108–109, 63–71. DOI: 10.1016/j.cis.2003.10.011.
- Mezger, T. G.; The Rheology Handbook; Vincentz Network: Hannover, Germany, 2006.
- Dimitrova, T. D.; Gurkov, T. D.; Vassileva, N.; Campbell, B.; Borwankar, R. P. Kinetics of Cream Formation by the Mechanism of Consolidation in Flocculating Emulsions. Journal of Colloid and Interface Science 2000, 230, 254–267. DOI: 10.1006/jcis.2000.7075.
- Magdassi, S.; Kamyshny, A. Introduction: Surface Activity and Functional Properties of Proteins. In Surface Activity of Proteins: Chemical and Physicochemical Modifications; Magdassi, S., Ed.; Marcel Dekker, Inc.: New York, Basel, Hong Kong, 1996.
- Damodaran, S. Interfaces, Protein Films, and Foams. Advances in Food and Nutrition Research 1990, 34, 1–79.
- Sethuraman, A.; Vedantham, G.; Imoto, T.; Przybycien, T.; Belfort, G. Protein Unfolding at Interfaces: Slow Dynamics of α-helix to β-sheet Transition. Proteins: Structure, Function and Bioinformatics 2004, 56, 669–678. DOI: 10.1002/prot.20183.