ABSTRACT
The aim of this study was to investigate the effect of superchilling at −2°C in comparison with refrigerated storage at 4°C on the protein degradation of olive flounder (Paralichthys olivaceus) muscle. Flounder muscle softened and shear force value decreased markedly (P < 0.05) with prolonged storage time, while values of electrical conductivity, TCA-soluble peptide, free amino acids, and proteolysis index increased (P < 0.05). The changes were slowed down significantly in samples superchilled at −2°C (P < 0.05). The fracture of muscle fibre and formation of cracks were accelerated in the samples refrigerated at 4°C, and intercellular spaces were observed after 9 days of storage. Moreover, protein bands of myosin heavy chain (MHC), actin, tropomyosin, 97 kDa, 50 ~ 60 kDa and 35 ~ 36 kDa occurred in varying degrees of degradation with storage time. The results demonstrated that significant postmortem degradation of muscle proteins occurred with extending storage time, while the changes were retarded obviously in samples during superchilled storage.
Introduction
Olive flounder (Paralichthys olivaceus) is widely cultured in China for its high economic value, as well as delicious taste and nutritional properties. The flounder is traditionally sold alive as a whole or stored in ice in the market. Nevertheless, raw fish are highly perishable and fish muscle tissue deteriorates rapidly by successive autolytic modifications, microorganisms and biochemical reactions.[Citation1] Postmortem changes generally appear in fish after slaughter, including protein degradation, lipid oxidation, changes in histological texture, and so on.[Citation2] All of these can lead to disorganization of muscular structure and loss of flesh quality of fish.
Protein degradation is one of the most important biochemical reactions in fish muscle, which is mainly attributed to the action of indigenous proteases and exogenous proteases from microorganisms.[Citation3,Citation4] It affects both texture and flavour of fish muscle owing to the formation of several low molecular weight compounds, including peptides, free amino acids, and amines. During the storage, autolysis of cephalothorax could take place, releasing the active proteases into the muscle, followed by fish muscle softening associated with the development of mushy texture along with decreased shear force and disintegration of microstructure.[Citation5] At the early postmortem stage, degradation of myofibrillar proteins and changes in texture are mainly attributed to endogenous proteolytic activity like lysosomal cathepsins and calpain. [Citation6–Citation8] Cathepsins such as cathepsins B and L can give rise to the degradation of myosin heavy chain and autolyze fish muscle during postmortem storage.[Citation9] Calpains are neutral Ca2+ activated cysteine endopeptidases in sarcoplasm, which can initiate the proteolytic degradation of myofibrils to produce new fragmented chains.[Citation6] With the prolongation of storage time, the proteins to decompose into small peptides and free amino acids could be much more enhanced in the presence of microorganisms and various endogenous proteases.[Citation9,Citation10]
Muscle proteolytic degradation appears simultaneously with cell death, attributing to the production of protein fragments, which influences the quality of fish muscle. Compared with terrestrial animal, fish muscle protein degraded faster after postmortem storage, which altered considerably depending on various factors such as fish species, handling and killing methods, the temperatures of their normal living and storage. [Citation11–Citation13] One of the most closely related aspects is postmortem storage temperature, and since fish is more highly perishable than meat, the temperature is even more important. Therefore, to meet the extending demand for high muscle quality in the supermarket, fish should be stored at low-temperature immediately after capture or death. Refrigeration can slow down bacterial growth and enzymatic activity, and has traditionally been used for fish preservation. Superchilling which is positioned between refrigeration and freezing has been suggested as a promising method to prolong shelf-life of fish. It can present a lower degree of proteins denaturation and less mechanical damages to the muscle tissue structure.[Citation11,Citation14] However, studies about the influence of superchilling on fish muscle quality are still limited.
The olive flounder is a very important and economic marine fish species in China. However, to our knowledge, little information is available on the postmortem protein degradation of flounder during chilled storage. Therefore, in the present work, we investigated the effect of superchilling at −2°C and refrigeration at 4°C on the protein degradation of flounder muscle during postmortem storage. We achieved this goal by evaluating electrical conductivity, shear force, TCA-soluble peptide, free amino acids, and proteolysis index, as well as measuring histological properties. We also determined the myofibrillar and sarcoplasmic proteins patterns by SDS-PAGE.
Materials and methods
Fish sampling
Fresh olive flounder with an average weight of 700 ± 50 g were obtained from Linxi Aquatic Market (Jinzhou, Liaoning province, China). Fish were transported to the Food Processing Laboratory of Bohai University within 30 min, kept alive before being processed. Upon arrival, the fish were stunned by a sharp blow to the head and kept at 0°C before being used. Each sample was packaged whole body in polyethylene bag and stored at 4°C and −2°C for 15 days, respectively. Samples were taken out for subsequent quality analysis at 3-day intervals.
Electrical conductivity (EC) determination
The electrical conductivity of olive flounder was measured according to the method by Yao et al.[Citation15] with a little change. Minced fish muscle of 10 g was dissolved with 90 mL of distilled water. The mixture was homogenized for 30 min via a magnetic stirrer, and then filtered through a fluted filter paper. The filter liquor was measured with a portable digital EC meter (DDSJ-308A, Shanghai, China).
Shear force measurement
Five cylindrical cores of 1 cm diameter and 2 cm long were removed from each raw dorsal muscle parallel to the muscle fibres orientation. Each of the cylinders was then measured using a Tenderness meter (C-LM4, Northeast Agricultural University, Harbin, China) and maximal force during shearing was used as a shear force value. Shear force measurements on raw fish muscle were calculated as the arithmetical mean of the 5 cylinder maximum forces and expressed as kg.
TCA-soluble peptide determination
Three grams of minced fish muscle was homogenized with 27 mL of cold 5% (w/v) Trichloroacetic acid (TCA). The homogenate was obtained and kept in ice for 1 h and centrifuged at 10000 g for 5 min at 4°C. The TCA-soluble peptides content in the supernatant was determined by the Lowry method[Citation16] and expressed as μmol tyrosine/g muscle.
Free amino acid analysis
Two grams of minced fish sample were homogenated with 15 mL 10% cold TCA followed by standing for 2 h, and then centrifuged at 10000 g for 15 min. The pH of the supernatant was adjusted to 2.0 with 0.02 M HCl, and diluted with distilled water to 50 mL. Then, 5 mL of the solution was ultrafiltered through a 0.22 μm membrane, and the filtrate was analysed by an automated amino acid analyser (L-8900, Hitachi, Japan). The concentration of free amino acids was calculated by calibrating with standard amino acids and expressed as mg/100 g sample.
Proteolysis index (PI) determination
Non-protein nitrogen (NPN) was carried out with a Kjeltec™ 8400 Auto Analyzer Unit. Minced fish sample (5 g) was homogenized with 25 mL of 10% (w/v) TCA using a homogenizer (3 × 20 s at 5000 rpm), followed by standing at 4°C for overnight. The homogenate was centrifuged at 5000 rpm for 10 min at 4°C. The supernatant was filtered through neutral filter paper and diluted with 10% TCA to 50 mL. Then, 20 mL solution was obtained to digestive for 1.5 h, and the NPN content was determined. Total nitrogen (TN) content was measured by the Kjeldahl method, and the PI was calculated as the percentage ratio between NPN and TN.[Citation17]
Microstructure observation
Paravertebral muscle samples were excised and frozen in a refrigerator at −80°C for 2 h. Then, the muscle sample with 5μm thickness was obtained by semi-automatic freezing microtome (CM1850, Leika, Germany). Consecutively, the sections were transferred onto glass slides, stained with haematoxylin and eosin, followed by air-dried, and then observed and photographed using electron microscope (80i, Nikon eclipse, Japan).
Myofibril protein (MFP) and sarcoplasmic protein (SPP) preparation
Myofibrillar proteins were extracted according to the method of Park et al.[Citation18] with slight modifications. Five grams of fish muscle diluted with 5 times 20 mM Tris-HCl buffer (pH 7.2) were homogenized, and the homogenate was centrifuged at 5000 g for 20 min at 4°C. Then, removing the supernatant, and 5 times extraction buffer (0.6 M NaCl, 20 mM Tris-HCl, pH 7.2) were added into the precipitate for homogenizing. The homogenate was centrifuged at 4500 g for 20 min at 4°C. The obtained supernatant was the myofibrillar protein solution and stored at −80°C until further analysis.
Sarcoplasmic proteins were extracted by a modification of the method of Chen et al.[Citation19] Five grams of minced fish muscle diluted with 5 times 50 mM Tris-HCl buffer (pH 7.5) were homogenized. The homogenate was centrifuged at 5000 g for 30 min at 4°C. The obtained supernatant was the sarcoplasmic protein solution, and then stored at −80°C until further analysis.
SDS-page
Protein solutions include MFP and SSP (1 mL, 4 mg/mL) were mixed with an equal volume loading buffer (0.5 M Tris-HCl, pH 6.8, containing 20% glycerol, 4% SDS, and 10% β-ME) respectively. The samples (10 μL of protein) were performed on the polyacrylamide gel, which consisted of 10% separating gel and 4% stacking gel. Gels were run at a constant voltage of 80 V for 20 min, and finished work at a constant voltage of 120 V using a Mini Protein II unit (Bio-Rad, USA). After electrophoresis, the gels were stained with 0.25% (w/v) coomassie blue R-250 in 10% (v/v) acetic acid and 40% (v/v) ethanol, and then destained in a solution comprising 10% (v/v) acetic acid and 40% (v/v) ethanol. Proteins molecular weight was estimated by wide molecular weight marker.
Statistical analysis
Analyses were carried out in triplicate using randomly selected samples. Statistical analysis was performed using the software SPSS version 20.0. Data were subjected to one-way ANOVA and bivariate correlation analysis. Differences at P < 0.05 were considered significant.
Results and discussion
Electrical conductivity (EC)
EC is widely used as an index of the electrolytes concentration in the muscle tissues, which can impact body-fluid balance, survival, and meat quality.[Citation15] The fish muscle tissues get decomposed and fluids flow out during the storage, leading to the increase of electrical conductance. It has been suggested that a completely intact muscle tissue will have the low value of EC which rises with an increase of fluids within the muscle.[Citation20] As presented in , the initial EC value was approximately 952 μs/cm and EC values increased progressively as the storage time increased (P < 0.05). This phenomenon can be attributed to the degradation and metabolic products of fish muscle produced by the growth of bacteria during storage. And these products are ionic substances, which can alter the electrical conductance.[Citation21] Furthermore, it was obviously observed that samples kept at 4°C had significantly higher EC values than that of samples superchilled at −2°C (P < 0.05). The EC value of fish sample during 4°C storage increased rapidly, which reached 1172 μs/cm on the 3rd day, while the sample stored at −2°C reached 1188 μs/cm on the 9th day of storage. This fact was indicative of either a significant less bacterial population in the samples or the muscle tissues got decomposed more slowly due to the effect of low temperature.
Shear force
As shown in , shear force of flounder muscle decreased gradually with the extension of storage time (P < 0.05), and a sharp decrease observed in the initial period, especially for the early 3 days of storage. This decrease might be connected with the destruction of muscle fibres and reduction of fish flesh textural properties. The degradation of fish muscle tissue caused by hepatopancreatic enzymes started from the endomysium, perimysium, Z line and H zones with concurrent degradation of the connective tissue, collagen protein and myofibrillar protein, which resulted in destruction of muscle fibres and reduction of shear force.[Citation22] Decrease in textural properties of fish muscle during storage mainly owing to both the action of endogenous proteases and microbial activity, the former consisted of cathepsins, calpains and collagenases resulting in the proteins degradation, and the latter thereafter was accelerated along with the breakdown of proteins.[Citation23] Meanwhile, there was no significant decrease of shear force value observed after 12 days of storage (P > 0.05), which could be explained by the cell permeability reached the maximum under the stronger action of proteases enzymes. In addition, the shear force value of the fish samples stored at 4°C was lower than that of super chilled samples (P < 0.05), which was in agreement with the higher TCA-soluble peptide. This indicated that the destruction of muscle fibres and connective tissues could be inhibited and the texture of fish was better maintained during storage at lower temperature.
TCA-soluble peptide
The TCA-soluble peptide is considered to be an effective indicator for evaluating proteins degradation of fish muscle. Changes in TCA-soluble peptide contents as a function of storage time are shown in . The result presented that the content of TCA-soluble peptide increased with storage time and lower increase was observed in the period of day 0–9, followed by the marked increase after 9 days of storage (P < 0.05). The increase of TCA-soluble peptide throughout the storage is primarily attributed to the activity of endogenous and microbial proteases. In the early storage period, proteinases could be more released from cephalothorax to flesh as a consequence of autolysis. Thus, small peptides produced by the activity of indigenous proteinases and could serve as nutrients for the growth of microorganisms.[Citation24]
Figure 3. Changes in TCA-soluble peptides of olive flounder during chilled storage for 15 days. Data are the mean of triple replicates and vertical bars indicate ± SD.
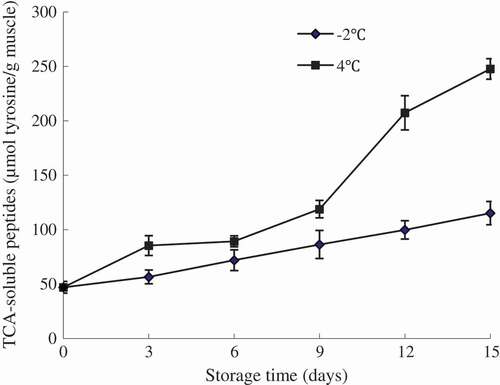
With the extension of storage time, the degradation of protein could be much more enhanced by increasing microorganisms and the highest TCA-soluble peptide content at the later of storage period was mainly attributed to the activity of exogenous enzymes.[Citation25] In general, only a slight increase in TCA-soluble peptide contents was observed for fish samples stored at −2°C, while a higher rate of increase was observed for the samples kept at 4°C (P < 0.05), suggesting the continuous occurrence of proteolysis during storage. This was in agreement with the lower shear force value of the sample at 4°C. The result suggested that the sample stored at lower temperature might contain lower activity of proteases, especially for trypsin-like proteases, which gave rise to a decrease in muscle-derived nitrogenous degradation products, thereby inhibiting the proliferation of bacteria and delaying the protein degradation.[Citation5,Citation26]
Free amino acids
Free amino acids (FAAs) have been used as immediate substrates for growing microorganisms in chilled fish, which lead to quality changes of the fish. There is a dynamic balance between the production and destruction of FAAs in fish muscle, and this balance is associated with muscle enzymes.[Citation27] The composition of FAAs in flounder muscle and their evolution during storage are given in . Some of these FAAs including Ala, Val, Glu, and Leu were the predominant free amino acids in flounder muscle. These amino acids were also highlighted as being more abundant in hake, rainbow trout and so on.[Citation27,Citation28] Ala is an extremely important amino acid, and an increase of which might improve the fish flesh flavour and taste, while this improvement would go unnoticed at the end of storage because it would be masked by other deterioration compounds such as ammonia and TMA.[Citation27]
Table 1. Changes in FAA (mg/100g sample) content of olive flounder during chilled storage for 15 days.
The initial FAAs content was 33.99 mg/100g, with the increasing of storage time, the content of FAAs increased significantly (P < 0.05). There were noticeable increases in the concentration of Glu, Ala, Met, Ile, Leu, Phe and Lys, and slight increases in the content of Asp, Cys, Ser, and His in fish muscle throughout the storage. The result indicated that significant proportions of the amino acids released during storage are hydrophobic. Hughes et al.[Citation4] also found large increases in the concentration of hydrophobic amino acids throughout the ripening of sausages. Increase of FAAs in fish muscle during storage may be caused by the prevailing chemical and enzymatic reactions, especially the activity of amino acid decarboxylase produced by surviving microorganisms.[Citation29] The content of Val increased in flounder sample stored at −2°C in the initial 9 days of storage, followed by the marked decrease. Decrease in FAA concentration may be connected with greater consumption of this amino acid by the microorganisms that use it as a growth substrate, and hence with greater spoilage.[Citation30] Moreover, the fish stored at 4°C had a significantly higher content of the total FAAs compared with the samples at −2°C (P < 0.05) at the same storage period, which reached maximum levels of 729.75 and 244.76 mg/100g at the end of storage period, respectively. The concentrations of NH3 in samples stored at −2°C and 4°C were 0.1 and 0.29 mg/100g on the 15th day of storage, respectively. In the present study, the FAA content was in agreement with the PI and TCA-soluble peptide.
Proteolysis index
The degree of proteolysis (proteolysis index, PI) was calculated as the percentage ratio of Non-protein nitrogen to Total nitrogen.[Citation17] Non-protein nitrogen mainly consists of the total content of peptide nitrogen, free amino acid nitrogen, nucleic acid nitrogen, and volatile basic nitrogen. It has been reported that proteolysis of flesh during storage was influenced by the increase of NPN concentration.[Citation31] As shown in , the PI of fish samples stored at 4°C increased progressively throughout the storage (P < 0.05). However, a slight increase of proteolysis index in the samples kept at −2°C were noted at initial 9 days of storage, but a marked increase was observed after 9 days of storage (P < 0.05). At early storage period, the protein degradation is mainly caused by the activity of indigenous proteinases,[Citation25] and with the extension of storage time, the protein degradation was accelerated in the action of exogenous enzymes caused by the growth of spoilage bacteria.[Citation24] Additionally, at the same storage period, fish samples superchilled at −2°C had a significantly lower proteolysis index compared with the samples at 4°C (P < 0.05), which reached maximum levels of 8.2% and 9.2% at the end of the storage period, respectively. The results from this study indicated that superchilling could have an obvious inhibitory effect on the proteases activities, and therefore retard the protein degradation.[Citation3]
Microstructure observation
The histological feature is an important indicator for evaluating the fish quality, and can reflect the protein degradation of fish muscle indirectly. The texture of fish muscle depends on inherent biological factors, including the content of collagen and fat, and the autolytic processes and microbiological activity, which could generate degradation of myofibrillar protein integration and eventual softening of the muscle.[Citation32] Degradation of myosin and actin disrupts the formation of actomyosin and results in the damage of structural integrity. [Citation33]
As shown in , fresh fish muscle tissue showed integrity structure and uniform muscle fibre. With the extension of storage time, the texture properties of muscle deteriorated, and varying degrees of deformation and fracture appeared. The increased spacing or gaping between the muscle fibres was noticeable from day 9 to 15 at both storage temperatures. For samples at −2°C, the number of detachments between muscle cells increased and the cracks within muscle cells developed significantly after 9 days of storage, and some loose fibres gathered leading to the muscle structure more irregular at day 15. However, the fracture of muscle fibre and formation of cracks within cells were accelerated obviously in the samples refrigerated at 4°C, and the poorer intercellular connection was observed in fish muscle after 9 days of storage. At an earlier stage of storage, integrity of muscle cells could resist the contracting force, while with the decline of binding force, muscle cells would separate from each other.[Citation34] Collagen has been reported as a major element for muscle cellular connection, and a slight component of which disintegrates with increasing storage time associated with muscle softening.[Citation35] The result of the present work suggested that the lower storage temperature could have a certain inhibitory effect on proteinases activity, disintegration of protein and deterioration of muscle tissue structure. This observation was consistent with the findings by Ando et al.[Citation34]
SDS-page
The electrophoretic profiles of MFP and SPP in flounder muscle stored at different temperatures are shown in . The molecular weight was in the range of 6.5 ~ 200 kDa. Protein patterns of muscle in all samples remained no obvious changes during the first 6 days of storage. However, the proteins band intensities decreased progressively throughout the later storage period, which coincided with the other protein degradation parameters measured above. Besides, downtrends of fragments in fish muscle stored at 4°C were more significant than that of sample kept at −2°C.
Figure 6. SDS-PAGE pattern of olive flounder muscle proteins during chilled storage for 15 days (a: MFP samples at −2°C; b: SSP samples at −2°C; c: MFP samples at 4°C; d: SSP samples at 4°C).
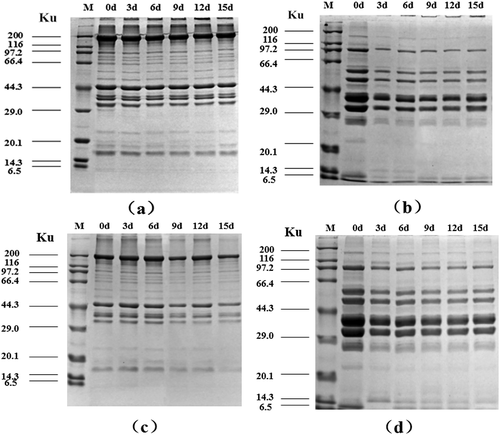
Myosin heavy chain (MHC, 200 kDa), actin (43 kDa), and tropomyosin (36 kDa) were the most predominant protein bands of MFP in flounder muscle, and similar protein bands were observed in other fishes (sea bass, carp).[Citation12,Citation36] As seen in a, c, the bands intensities of MHC, tropomyosin and actin of MFP decreased markedly during the whole storage, especially after 6 days of storage at 4°C. The degradation of MHC and actin may be possibly attributed to the postmortem cathepsins B and L activity,[Citation37] and the recent study reported that µ-calpain could hydrolyse MHC and actin at suitable condition (pH around 5.5 and Ca2+ concentration around 1mM).[Citation38] The intensities of tropomyosin and troponin T also decreased gradually during the whole storage, which could prove that muscle degradation rate was rising up with the increasing storage time. Troponin T is regarded as another indicator of postmortem proteolysis, degradation of which has been proved to be highly connected with the postmortem muscle tenderization.[Citation38] Sarcoplasmic protein comprises approximately 20–40% of total fish muscle protein, and probably includes more enzymes involved in protein catabolism and proteolysis. [Citation12] The SDS-PAGE patterns of SPP in flounder muscle contained 6 major protein bands with molecular weights ranged from 6.5 to 180 kDa ( b, d). There was one band of approximately 26 ~ 27 kDa, two of 35 ~ 40 kDa, one of 50 kDa, 60 kDa and 97 kDa. The protein bands with molecular weights of 36 and 39 kDa were assumed to be glyceraldehyde-3-phosphate dehydrogenase and aldolase in sarcoplasmic protein of threadfin bream (Nemipterus sp.),[Citation39] and the band with molecular weights of 97 kDa was reported to represent phosphorylase.[Citation37] The research presented a slight decrease in the intensity of the bands of about 97 kDa, 60 kDa, 50 kDa, and 35 ~ 36 kDa, which demonstrated the degradation of sarcoplasmic protein.
Conclusion
Within the 15 days of storage, the postmortem degradation of muscle protein was observed obviously with increasing storage time, but lower storage temperature showed significantly more preservative effect. The changes in EC, shear force, TCA-soluble peptide, FAAs and PI with the storage time were slowed down significantly in fish samples superchilled at −2°C, as well as the inhibited damages of muscle tissue structure. Moreover, the gradual degradation of protein bands of MHC, actin, tropomyosin, 97 kDa, 60 kDa, 50 kDa, and 35 ~ 36 kDa appeared in varying degrees during storage. The results suggested that superchilling can retard the protein degradation of flounder muscle during postmortem storage, and can be applied in fish processing industry or aquatic market to keep the quality of fish.
Acknowledgments
We gratefully acknowledge the Food Safety Key Lab of Liaoning Province for providing experimental conditions.
Additional information
Funding
References
- Caballero, M. J.; Betancor, M.; Escrig, J. C.; Montero, D.; Espinosa De Los Monterosa, A.; Castro, P.; Ginés, R.; Izquierdo, M. Post Mortem Changes Produced in the Muscle of Sea Bream (Sparus Aurata) during Ice Storage. Aquaculture 2009, 291, 210–216.
- Xu, Y.; Liu, Y.; Jiang, C.; Zhang, C.; Li, X.; Zhu, D.; Li, J. Determination of Volatile Compounds in Turbot (Psetta Maxima) during Refrigerated Storage by Headspace Solid-Phase Microextraction and Gas Chromatography-Mass Spectrometry. Journal of the Science of Food and Agriculture 2014, 94, 2464–2471.
- Gaarder, M. Ø.; Bahuaud, D.; Veiseth-Kent, E.; Mørkøre, T.; Thomassen, M. S. Relevance of Calpain and Calpastatin Activity for Texture in Super-Chilled and Ice-Stored Atlantic Salmon (Salmo Salar L.). Fillets. Food Chemistry 2012, 132, 9–17.
- Hughes, M. C.; Kerry, J. P.; Arendt, E. K.; Kenneally, P. M.; Mcsweeney, P. L.; O’neill, E. E. Characterization of Proteolysis during the Ripening of Semi-Dry Fermented Sausages. Meat Science 2002, 62, 205–216.
- Sriket, C.; Benjakul, S.; Visessanguan, W.; Hara, K.; Yoshida, A. Retardation of Post-Mortem Changes of Freshwater Prawn (Macrobrachium Rosenbergii) Stored in Ice by Legume Seed Extracts. Food Chemistry 2012, 135, 571–579.
- Ahmed, Z.; Donkor, O.; Street, W. A.; Vasiljevic, T. Calpains- and Cathepsins- Induced Myofibrillar Changes in Post-Mortem Fish: Impact on Structural Softening and Release of Bioactive Peptides. Trends in Food Science and Technology 2015, 45, 130–146.
- Lu, H.; Liu, X.; Zhang, Y.; Wang, H.; Luo, Y. Effects of Chilling and Partial Freezing on Rigor Mortis Changes of Bighead Carp (Aristichthys Nobilis) Fillets: Cathepsin Activity, Protein Degradation and Microstructure of Myofibrils. Journal of Food Science 2015, 80, C2725–C2731.
- Yang, F.; Rustad, T.; Xu, Y.; Jiang, Q.; Xia, W. Endogenous Proteolytic enzymes-A Study of Their Impact on Cod (Gadus Morhua) Muscle Proteins and Textural Properties in a Fermented Product. Food Chemistry 2015, 172, 551–558.
- Wang, P. A.; Vang, B.; Pedersen, A. M.; Martinez, I.; Olsen, R. L. Post-Mortem Degradation of Myosin Heavy Chain in Intact Fish Muscle: Effects of pH and Enzyme Inhibitors. Food Chemistry 2011, 124, 1090–1095.
- Chen, X.; Wu, G. P.; Cai, Q. F.; Liu, G. M.; Osatomi, K.; Su, W. J.; Cao, M. J. Biochemical Characterisation of an Aminopeptidase with Highest Preference for Lysine from Japanese Flounder Skeletal Muscle. Food Chemistry 2012, 130, 679–686.
- Liu, D.; Liang, L.; Xia, W.; Regenstein, J. W.; Zhou, P. Biochemical and Physical Changes of Grass Carp (Ctenopharyngodon Idella) Fillets Stored at −3 and 0°C. Food Chemistry 2013, 140, 105–114.
- Li, Q.; Zhang, L.; Lu, H.; Song, S.; Luo, Y. Comparison of Postmortem Changes in ATP-related Compounds, Protein Degradation and Endogenous Enzyme Activity of White Muscle and Dark Muscle from Common Carp (Cyprinus Carpio) Stored at 4°C. LWT-Food Science and Technology 2017, 78, 317–324.
- Hultmann, L.; Rustad, T. Iced Storage of Atlantic Salmon (Salmo Salar)-Effects on Endogenous Enzymes and Their Impact on Muscle Proteins and Texture. Food Chemistry 2004, 87, 31–41.
- Digre, H.; Erikson, U.; Aursand, I. G.; Gallart-Jornet, L.; Misimi, E.; Rustad, T. Rested and Stressed Farmed Atlantic Cod (Gadus Morhua) Chilled in Ice or Slurry and Effects on Quality. Journal of Food Science 2011, 76, S89–S100.
- Yao, L.; Luo, Y.; Sun, Y.; Shen, H. Establishment of Kinetic Models Based on Electrical Conductivity and Freshness Indictors for the Forecasting of Crucian Carp (Carassius Carassius) Freshness. Journal of Food Engineering 2011, 107, 147–151.
- Lowry, O. H.; Rosebrough, N. J.; Farr, A. L.; Randall, R. J. Protein Measurement with the Folin Phenol Reagent. Journal of Biological Chemistry 1951, 193, 265–275.
- Schivazappa, C.; Degni, M.; Costa, L. N.; Russo, V.; Buttazzoni, L.; Virgili, R. Analysis of Raw Meat to Predict Proteolysis in Parma Ham. Meat Science 2002, 60, 77–83.
- Park, D.; Xiong, Y. L.; Alderton, A. L.; Ooizumi, T. Biochemical Changes in Myofibrillar Protein Isolates Exposed to Three Oxidizing Systems. Journal of Agricultural and Food Chemistry 2006, 54, 4445–4451.
- Chen, Q.; Liu, Q.; Sun, Q.; Kong, B.; Xiong, Y. Flavour Formation from Hydrolysis of Pork Sarcoplasmic Protein Extract by a Unique LAB Culture Isolated from Harbin Dry Sausage. Meat Science 2015, 100, 110–117.
- Byrne, C. E.; Troy, D. J.; Buckley, D. J. Postmortem Changes in Muscle Electrical Properties of Bovine M. Longissimus Dorsi and Their Relationship to Meat Quality Attributes and pH Fall. Meat Science 2000, 54, 23–34.
- Ekanem, E. O.; Achinewhu, S. C. Effects of Shucking Method on Opening, Meat Yield and Selected Quality Parameters of West African Clam, Galatea Paradoxa. Journal of Food Processing and Preservation 2000, 24, 365–377.
- Sáez, M. I.; Suárez, M. D.; Cárdenas, S.; Martínez, T. F. Freezing and Freezing-Thawing Cycles on Textural and Biochemical Changes of Meagre (Argyrosomus Regius, L) Fillets during Further Cold Storage. International Journal of Food Properties 2015, 18, 1635–1647.
- Cai, L.; Wu, X.; Dong, Z.; Li, X.; Yi, S.; Li, J. Physicochemical Responses and Quality Changes of Red Sea Bream (Pagrosomus Major) to Gum Arabic Coating Enriched with Ergothioneine Treatment during Refrigerated Storage. Food Chemistry 2014, 160, 82–89.
- Sriket, C.; Benjakul, S.; Visessanguan, W. Post-Mortem Changes of Muscle from Fresh Water Prawn (Macrobrachium Rosenbergii) as Influenced by Spawning Stages. LWT-Food Science and Technology 2010, 43, 608–616.
- Pacheco-Aguilar, R.; Lugo-Sánchez, M.; Robles-Burgueño, M. Postmortem Biochemical and Functional Characteristic of Monterey Sardine Muscle Stored at 0°C. Journal of Food Science 2000, 65, 40–47.
- Cai, L.; Wang, Y.; Cao, A.; Lv, Y.; Li, J. Effect of Alginate Coating Enriched with 6-Gingerol on the Shelf Life and Quality Changes of Refrigerated Red Sea Bream (Pagrosomus Major) Fillets. RSC Advances 2015, 5, 36882–36889.
- Capillas, C. R.; Moral, A. Changes in Free Amino Acids during Chilled Storage of Hake (Merluccius Merluccius L.) In Controlled Atmospheres and Their Use as a Quality Control Index. European Food Research and Technology 2001, 212, 302–307.
- Özden, Ö.;. Changes in Amino Acid and Fatty Acid Composition during Shelf-Life of Marinated Fish. Journal of the Science of Food and Agriculture 2005, 85, 2015–2020.
- Virgili, R.; Saccani, G.; Gabba, L.; Tanzi, E.; Bordini, C. S. Changes of Free Amino Acids and Biogenic Amines during Extended Ageing of Italian Dry-Cured Ham. LWT-Food Science and Technology 2007, 40, 871–878.
- Capillas, C. R.; Moral, A. Free Amino Acids and Biogenic Amines in Red and White Muscle of Tuna Stored in Controlled Atmospheres. Amino Acids 2004, 26, 125–132.
- Wu, H.; Zhang, Y.; Long, M.; Tang, J.; Yu, X.; Wang, J.; Zhang, J. Proteolysis and Sensory Properties of Dry-Cured Bacon as Affected by the Partial Substitution of Sodium Chloride with Potassium Chloride. Meat Science 2014, 96, 1325–1331.
- Li, T.; Li, J.; Hu, W.; Zhang, X.; Li, X.; Zhao, J. Shelf-Life Extension of Crucian Carp (Carassius Auratus) Using Natural Preservatives during Chilled Storage. Food Chemistry 2012, 135, 140–145.
- Wang, D.; Dong, H.; Zhang, M.; Liu, F.; Bian, H.; Zhu, Y.; Xu, W. Changes in Actomyosin Dissociation and Endogenous Enzyme Activities during Heating and Their Relationship with Duck Meat Tenderness. Food Chemistry 2013, 141, 675–679.
- Ando, M.; Nakamura, H.; Harada, R.; Yamane, A. Effect of Super Chilling Storage on Maintenance of Freshness of Kuruma Prawn. Food Science and Technology Research 2004, 10, 25–31.
- Jiang, X.; Xu, Y.; Ge, L.; Xia, W.; Jiang, Q. The Impact of Collagen on Softening of Grass Carp (Ctenopharyngodon Idella) Fillets Stored under Superchilled and Ice Storage. International Journal of Food Science and Technology 2015, 50, 2427–2435.
- Boulares, M.; Mankai, M.; Belaam, Z.; Hassouna, M. Effect of Inoculation of Lactic Acid Bacteria on Proteolytic Activity of Psychrotrophic Gram-Negative Bacteria in Fresh Farmed Sea Bass (Dicentrarchus Labrax) Fillets during Storage at 4°C under Vacuum-Packed Conditions. Annals of Microbiology 2013, 63, 1493–1500.
- Xu, Y.; Ge, L.; Jiang, X.; Xia, W.; Jiang, Q. Inhibitory Effect of Aqueous Extract of Allium Species on Endogenous Cathepsin Activities and Textural Deterioration of Ice-Stored Grass Carp Fillets. Food and Bioprocess Technology 2015, 8, 2171–2175.
- Li, Z.; Li, M.; Du, M.; Shen, Q. W.; Zhang, D. Dephosphorylation Enhances Postmortem Degradation of Myofibrillar Proteins. Food Chemistry 2018, 245, 233–239.
- Yongsawatdigul, J.; Hemung, B. O. Structural Changes and Functional Properties of Threadfin Bream Sarcoplasmic Proteins Subjected to pH-shifting Treatments and Lyophilization. Journal of Food Science 2010, 75, C251–C257.