ABSTRACT
The cryoprotective effects on surimi matrix of hydrolysates with different hydrolyzing times prepared from silver carp using Protamex were compared with commercial sorbitol-sucrose cryoprotectants. Cryoprotective assays showed that FPH-30 (hydrolysate with 30 min hydrolyzing) was a more effective cryoprotectant than other hydrolysates, because the FPH-30 group displayed lower salt-soluble protein extractability loss (29.90 ± 0.84%), less actomyosin Ca2+-ATPase activity decrease (48.85 ± 2.56%) and unfrozen water content decrease (10.39 ± 0.63%) after six freeze-thaw cycles. To exploit industrial utilizations, FPH-30 was further purified with ultrafiltration membranes. The cryoprotective activity of the fraction (<3 kDa) was superior to the others by reducing the salt-soluble protein extractability loss (27.21 ± 0.98%) and impeding the actomyosin Ca2+-ATPase activity decrease (30.54 ± 1.21%). Mass spectrometry analysis showed that the fraction (<3 kDa) was mainly composed of three peptides of the sequences GVDNPGHPFIM(T) and IITNWDDMEK. The results clearly support that silver carp could be a potential source of cryoprotectant peptides for industrial applications.
Introduction
Annually, approximately 4.9 million metric tons of silver carp (Hypophthalmichthys molitrix) are produced globally.[Citation1] Silver carp is the most common carp species used to produce frozen surimi in China. Cryoprotectants are often used to avoid deterioration of surimi quality induced by freezing and/or fluctuating temperatures. A sorbitol and sucrose blend (SuSo) are a commonly used commercial cryoprotectant. However, it imparts sweetness to the product and cannot meet the needs of many consumers and certain market segments with dietary restrictions such as diabetics who cannot accept the sweetness or additional caloric and glycemic values of surimi products.[Citation2,Citation3]
Some protein hydrolysates and peptides are effective cryoprotectants for frozen fish surimi that experiences repeated freeze-thaw treatments. Importantly the cryopreservation effects are equal or superior to that of commercial SuSo.[Citation2,Citation4–Citation11] Therefore, the use of protein hydrolysates as cryoprotectants in surimi would be preferable. However, protein hydrolysates are composed of a wide variety of peptides with different molecular weights and free amino acids.[Citation12] This makes it difficult to define the cryoprotective mechanism of hydrolysates in surimi quality preservation and how hydrolysate properties and cryoprotectant efficiency are related. In addition, considering other cryoprotective applications of hydrolysates, such as being cryoprotective agents for uses in cells[Citation10] or ice cream[Citation13], chromatography and separation and purification methods can be used to further fractionate the hydrolysate on a small laboratory scale.[Citation13]
Currently, there are huge challenges in the exploitation of peptide isolation and recovery procedures on a industrial scale, which are desirable for application in food processing. And there are always economic needs to explore low-cost processes. Our studies reveal that controlled enzymatic hydrolysis produces protein hydrolysates with different structures and functional properties from silver carp surimi by-products, which are low-cost materials.[Citation14] However, questions remain about the standardization and consistency of the by-products. The by-products are mixed with viscera, which contain a lot of proteolytic enzymes.
There is limited information about the cryoprotective ability of protein hydrolysates prepared from freshwater fish, such as silver carp. Therefore, in this study silver carps were digested into hydrolysates using Protamex based on our previous findings[Citation14] and the cryoprotective activities of the hydrolysates were characterized using surimi matrices and cryoprotection assays. Furthermore, to exploit the industrial uses, ultrafiltration separation, more easily and commonly applied to the industrial production of bioactive substances, was used to isolate the hydrolysates, which demonstrated the best cryoprotective activities based on the cryoprotection assays. Mass spectrometry was further used to identify the cryoprotective peptides, providing supporting data for the industrial application of this new cryoprotective agent.
Materials and methods
Materials
Fresh live silver carp (Hypophthalmichthys molitrix) at 2.0 ± 0.5 kg/fish from a local farmers’ market near Changsha city (China) were transported to the laboratory alive within 30 min. The enzymes Protamex (the minimum activity of 1.5 AU/g) was purchased from Novozymes Inc. (Suzhou, China). Chemicals used in all experiments were analytically pure.
Preparation of fish protein hydrolysates (FPH)
Fresh silver carp were scaled, beheaded and gutted, and washed thoroughly. The meat was manually carved away from the bones and immediately minced to uniformity. The minced fish meat was hydrolyzed using a modified method of Liu et al .[Citation14] The meat was suspended in deionized water with a solid:liquid ratio of 1:5 (w/v). After heating at 90°C for 10 min to inactivate endogenous enzymes, Protamex was added to the suspensions at 3.0% (w/w) enzyme to a substrate, and incubated for 15, 30, 60, 120, or 240 min (pH 6.5 and 50ºC). Inactivation of Protamex was performed by heating at 80°C for 10 min. Then, the slurries were centrifuged at 8000 g for 10 min (4°C). The obtained supernatants were dialyzed, and freeze-dried ultimately yielding the fish protein hydrolysates (FPH-15, FPH-30, FPH-60, FPH-120, and FPH-240).
Characterization of FPH
The degree of hydrolysis (DH) of the FPHs was confirmed by the pH-stat method.[Citation15] 1 mol/L NaOH was used to maintain a constant pH value during hydrolysis. The relative molecular weight distribution of the FPHs (0.5%, w/v) was estimated with size exclusion chromatography. A TSK-gel G3000 PWXL column with an exclusion limit of 500–800,000 Da was applied, and the average retention time of five standards (bovine serum albumin, peroxidase, ribonuclease A, glycine tetramer and p-Aminobenzoic acid) (Sigma, USA) in the column were used to obtain a molecular weight calibration curve.
Characterization of the cryoprotective ability of FPH
Preparation of freeze-thaw surimi for cryoprotection analysis
Fresh silver carp were scaled, beheaded and gutted, and washed thoroughly. The fish meat was picked carefully and washed twice with two volumes of chilled water. After centrifugation at 3000 g (5 min, 4°C) to remove the surface water, the meat was cut and minced at 4°C for 1 min by a food processor (FP580, Kenwood, UK). The resulting pastes were mixed with the hydrolysates to obtain the following samples: FPH-15, FPH-30, FPH-60, FPH-120 and FPH-240 groups (100 g paste with the addition of 2 g FPH-15, FPH-30, FPH-60, FPH-120, and FPH-240, respectively). SuSo (100 g paste with 4% sorbitol + 4% sucrose) and control (100 g paste without cryoprotectant) groups were also prepared for comparison. All samples were prepared in triplicate and called “surimi”. The samples were all separated into two parts to be analyzed immediately and after freeze-thaw treatments. Six cycles of freeze-thaw treatments were carried out (−25 ± 1°C for 12 h and 4 ± 1°C for 12 h per cycle).
Salt-soluble protein (SSP) extractability: SSPs were extracted from unfrozen and freeze-thaw surimi samples. The hydrolysates or SuSo in each sample was removed before extraction. About 30 g of surimi was fully dispersed into 10 volumes of chilled distilled water (4°C) by homogenization for 1 min at 10,000 r/min using an IKA T10 homogenizer (Germany). After centrifuging at 8000 g (10 min, 4°C), the obtained precipitate was dispersed thoroughly in 10 volumes of 100 mmol/L sodium phosphate buffer (0.6 mol/L NaCl, pH 7.5). The extraction procedure was conducted twice. The two supernatants were combined and the protein concentration was measured by the Folin–phenol method.[Citation16] The percentage of the protein concentration after each freeze-thaw cycle to that of the initial unfrozen surimi was expressed as salt-soluble protein extractability.
Actomyosin Ca2+-ATPase activity: The actomyosin was extracted from the surimi samples using the method of Kittiphattanabawon et al.[Citation7] Actomyosin Ca2+-ATPase activity was tested according to the method of Wang et al.[Citation17] Each sample was suspended in 10 volumes of 20 mmol/L phosphate buffer (pH7.0, with KCl 0.6 mol/L), and Ca2+-ATPase measurement kits (Nanjing Jiancheng Bioengineering Institute, China) were used with the detective wavelength fixed at 636 nm.
Unfrozen water content
Unfrozen water was tested by DSC (differential scanning calorimeter, Q2000, TA Instruments, USA). Unfrozen and freeze-thaw surimi samples (accurately weighed about 5 mg, equivalent content of moisture in all samples) were placed in aluminum cells, hermetically sealed and reweighed, and a sealed blank cell was used as a reference. The testing procedures were as follows: 1) decrease temperature from 5 to −10°C and equilibrate for 10 min; 2) continuously cool to −20°C and equilibrate for 10 min; 3) increase temperature to −10°C and equilibrate for 10 min; 4) continuously heat to 5°C and equilibrate for 10 min. The cooling and heating speeds were fixed at 1°C/min. The content of the unfrozen (bound) water in the unfrozen and freeze-thaw surimi was calculated according to the method of Mueller et al.[Citation8] The ΔH value was 314.00 J/g, which is not much different from the reported value (337.33 J/g).[Citation18] The water mass of the samples were calculated by moisture analysis.
Screening of the best cryoprotective components
The hydrolysate with the best cryoprotective effect based on the results of cryoprotection assays was separated by ultrafiltration Centrifugal Filter Devices with 10 kDa and 3 kDa Ultracel-PL regenerated cellulose membranes (Amicon Ultra-15, Millipore, USA). Fractions with different molecular weights (<3 kDa, 3–10 kDa, <10 kDa and >10 kDa) were collected for further cryoprotective ability assessments. The relative molecular weight distributions of the fractions were verified by size exclusion chromatography. The SSP extractability and actomyosin Ca2+-ATPase activity of the surimi samples added with different FPH fractions and SuSo were used to screen the best cryoprotective fraction. The specific methods were described as above.
Analysis of peptide sequence
Membrane separated fractions were dissolved in 20 uL of water/acetonitrile mobile phase A (98%, +0.1% formic acid). Ten microliterwas applied to a Triple TOF 5600 LC-MS/MS (AB SCIEX, USA) mass spectrometer for analysis. The samples were first enriched and desalted at a flow rate of 2.5 µL/min on a Trap column for 10 min and then the gradient was eluted on an analytical column (3 µm 120 Å Eksigent ChromXP C18) with 5% to 40% acetonitrile/water mobile phase B (98%, + 0.1% formic acid) for 40 min. Electrospray ionization was performed with a Turbo V™ ionization source in positive ion mode (spray voltage of 2300 V, atomizing gas of 5 psi, and heating temperature of 150°C). The Information Dependent Analysis (IDA) mode was used. In the sensitivity mode, the cycle time for each first and second stage was 2.5 s, and the cumulative scan time was 0.25 s. The maximum number of second stages for each cycle was 25, and the cumulative time was 90 min. Parent ions with intensity greater than 150 cps were selected for secondary mass spectrometric analysis, with a parent ion exclusion time of 16 s. Obtained MS/MS data were analyzed by ProteinPilot 4.5 software (AB SCIEX, USA).
Statistical analysis
Tests in this study were all conducted no less than three independent times. Standard deviations of the data were obtained. Duncan’s multiple-range test was carried out in the one-way analysis of variance by SPSS 13.0 software (Chicago, USA) with a significant difference set at P < .05.
Results and discussion
Characterization of hydrolysates (FPHs)
The proximate composition and DH values of the FPHs are shown in . Our previous studies have shown that Protamex could efficiently hydrolyze silver carp processing by-products.[Citation14] In this study, there were no significant differences in protein, ash or moisture content of the hydrolysates produced with different hydrolyzing times (P > .05). It can be observed that with hydrolyzing time increased from 15 to 240 min, the DH values of the FPHs were substantially raised from 9.68 ± 0.40% to 29.10 ± 1.33% (P < .05), showing that Protamex is suitable for enzymatic hydrolysis of silver carp protein.
Table 1. Characterization of the FPH
Calibration curve of five standard substances on the TSK-gel G3000 PWXL column was generated as a reference for data interpretation (data not shown). The FPHs had wide varieties of fractions with molecular weights (Mw) ranging from 112 Da to 370 kDa, indicating that the FPHs contained peptides of different sizes and small amounts of free amino acids (). With increased DHs, the amount of small-molecular-weight peptide fragments or free amino acids increased. Peptides constituted the dominant proportions of the FPHs. The molecular weight of the hydrolysates or peptides added to frozen-minced fish has significant impacts on the formation and growth of ice crystals, and on their interactions with fish myofibrillar proteins.[Citation2,Citation4,Citation19]
Assessment of the FPHs as cryoprotectants
Salt-soluble protein extractability (SSP)
Protein extractability is indicative of protein denaturation and aggregation in frozen samples.[Citation20] Myofibrillar proteins lose the native conformation upon frozen storage or freeze-thaw cycles and form insoluble aggregates that cannot be recovered in salt solutions.[Citation21,Citation22] Salt-soluble protein extractability (SSP) decreased with freeze-thaw treatments for all samples (). However, the salt-soluble protein extractability of the control group decreased significantly faster than that of samples with added FPH or SuSo (P < .05). The control group had a 37.63 ± 2.05% loss after six freeze-thaw cycles, while the salt-soluble protein extractabilities of FPH-15, FPH-30, FPH-60, FPH-120, FPH-240, and SuSo groups only decreased by 30.97 ± 1.06, 29.90 ± 0.84, 32.52 ± 1.33, 31.45 ± 1.21, 34.54 ± 1.46 and 28.64 ± 1.01%, respectively. This indicates that added FPH can prevent salt-soluble protein extractability loss (P < .05) similar to commonly used commercial SuSo, and can retard muscle protein denaturation and aggregation caused by temperature abuse during freeze-thaw treatments.[Citation2]
Figure 2. Cryoprotective ability of the FPH during freeze-thaw cycles. (a) Salt-soluble protein extractability from freeze-thaw surimi samples added with the FPHs, SuSo, and control. (b) Ca2+-ATPase activity of actomyosin from different surimi samples during freeze-thaw cycles. The reported data are mean values from three replicates. Bars represent standard deviations
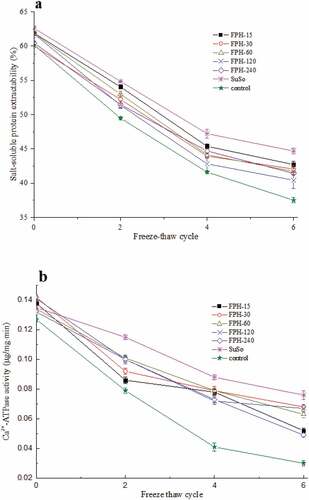
Ca2+-ATPase activity of actomyosin
Actomyosin is a main component of muscle protein[Citation23] that is degraded upon frozen storage or freeze-thaw treatments. Therefore, actomyosin Ca2+-ATPase activity is a good indicator of protein denaturation after freezing.[Citation24] All samples displayed decreased actomyosin Ca2+-ATPase activity at varying rates (). The activity of the control group initially decreased quickly and decreased by 76.38 ± 3.18% over six freeze-thaw cycles, while the activities of the FPH and SuSo groups were greater than that of the control group (P < .05). Therefore, like SuSo, FPH helped preserve Ca2+-ATPase activity. For the FPH-15, FPH-30, FPH-60, FPH-120, FPH-240 and SuSo groups, the Ca2+-ATPase activity decreased by 62.32 ± 1.99, 48.85 ± 2.56, 52.11 ± 1.21, 52.99 ± 1.34, 62.88 ± 2.79 and 43.70 ± 3.01%, respectively, after six freeze-thaw cycles. The FPH-30 group displayed the slowest decrease in the Ca2+-ATPase activity among all hydrolysate groups (P < .05), which further indicates that FPH-30 is the most effective protein structure stabilizer during freeze-thaw treatments. The decreases in Ca2+-ATPase activity correlated well with the salt-soluble protein extractability results. Furthermore, the amount of hydrolysate added to the surimi was important for maintaining Ca2+-ATPase activity. In our previous studies, the optimal amount of hydrolysate was 2% w/w, and higher hydrolysate percentages (4% and 6% w/w of hydrolysates, data not shown) could influence the formation of stable structures of the surimi, leading to increased protein–protein interaction, which also induced Ca2+-ATPase activity loss. However, Korzeniowska et al.[Citation3] found that addition of 8% w/w of Pacific hake protein hydrolysate was necessary to impede the structural deterioration of natural actomyosin during freeze-thaw treatments, while 2% w/w of hydrolysate did not reach the minimum level required for optimal cryoprotection, the observation that could potentially be attributed to differences in the nature of the hydrolysates.
Unfrozen water content
DSC-determined unfrozen water content in the freeze-thawed surimi was used to evaluate the water-binding capacity of the FPHs (). The control group had a 39.19 ± 2.01% decrease of unfrozen water content after six freeze-thaw cycles. While those of FPH-15, FPH-30, FPH-60, FPH-120, FPH-240 and SuSo groups decreased by 18.22 ± 0.39, 10.39 ± 0.63, 22.21 ± 1.87, 20.34 ± 1.28, 15.63 ± 0.78 and 14.29 ± 0.98%, respectively, after six freeze-thaw cycles. The FPH and SuSo groups showed significantly slower decreases in unfrozen water contents than the control group over six freeze-thaw cycles (P < .05), and the FPH-30 group showed the slowest decreases in unfrozen water content (P < .05). In this study, the surimi with FPH exhibited a higher initial unfrozen water content than the SuSo group, and significantly higher than the control group. The added FPH also helped to maintain the amount of unfrozen water during freeze-thaw treatments. Compared to the Ca2+-ATPase activity () of each surimi sample, SuSo retarded inactivation of Ca2+-ATPase activity and freeze-induced protein denaturation significantly, while the unfrozen water content of the SuSo-containing group was a little lower than those of the hydrolysate-containing groups. Sucrose and sorbitol effectively protected myofibrillar protein against freeze-inducing denaturation by stabilizing structures of the surrounding water. Similarly, FPH (with more polar residues exposed and free amino acid released during hydrolyzation) might inhibit water molecules from contacting ice crystals by binding to the surfaces of the ice crystals, thus maintaining the unfrozen water content. On the other hand, the protein hydrolysates, including the present FPHs, stabilized protein structure by binding water in three-dimensional structures of protein during frozen storage.[Citation18] Therefore, the results suggest that FPH and SuSo might adopt different mechanisms of cryoprotection. In this study, FPH-30 was the most effective cryoprotectant on the surimi and myofibrillar protein samples. In the subsequent experiments, FPH-30 was further separated and purified and the specific cryoprotective fractions of the hydrolysate were carefully identified.
Cryoprotective activities of membrane-separated fractions
Ultrafiltration separation technology is industrially friendly due to its low cost and high efficiency.[Citation25] In this study, FPH fractions (<3 kDa, 3–10 kDa, <10 kDa and >10 kDa) were satisfactorily prepared by ultrafiltration membrane separation and the relative molecular weight distributions determined (). The cryoprotective activities of the four fractions were investigated by characterizing the SSP extractability and Ca2+-ATPase activity of surimi samples added with the fractions and the SuSo group was used for comparison.
Figure 4. Relative molecular weight distributions of the fractions obtained by membrane separation from FPH-30 on a TSK G3000 PWXL column at 220 nm
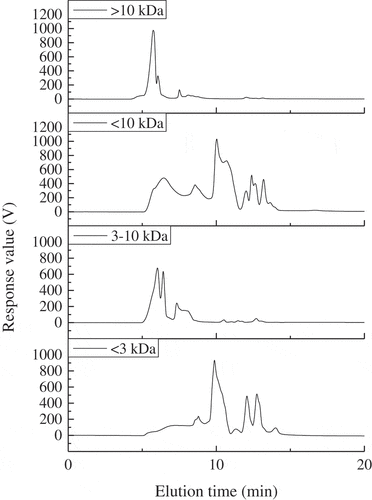
After six freeze-thaw cycles, the salt-soluble protein extractability of the four fractions (<3 kDa, 3–10 kDa, <10 kDa and >10 kDa) and Suso group decreased by 27.21 ± 0.98, 34.71 ± 1.62, 29.64 ± 1.14, 37.93 ± 0.80 and 27.90 ± 1.84%, respectively (). In addition, after six freeze-thaw cycles, the Ca2+-ATPase activities of the four fractions (<3 kDa, 3–10 kDa, <10 kDa and >10 kDa) and Suso group decreased by 30.54 ± 1.21, 37.17 ± 1.02, 32.94 ± 0.80, 45.52 ± 3.14 and 30.56 ± 1.60%, respectively (). The <10 kDa and <3 kDa fractions showed significantly better protection to myofibrillar proteins against freezing-induced denaturation than the other fractions (P < .05). The results also clearly support that the <3 kDa fraction demonstrated the same cryoprotective effects on the surimi samples against freeze-thaw abuse as commercial SuSo. This is in agreement with the previous conclusion that peptide chains of less than 2000 Da have sufficient hydrophilicity and flexibility, and could be better at binding to the surfaces of ice crystals and inhibiting their growth.[Citation26]
Figure 5. Cryoprotective ability of the fractions obtained by membrane separation from FPH-30 during freeze-thaw cycles. (a) Salt-soluble protein extractability from freeze-thaw surimi samples added with separated fractions and SuSo; (b) Ca2+-ATPase activity of actomyosin from different surimi samples during freeze-thaw cycles. The reported data are mean values from three replicates. Bars represent standard deviations
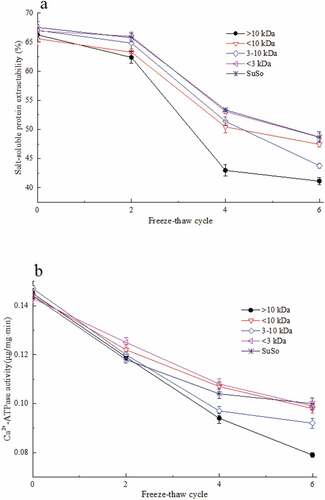
Identification of membrane separated fractions
The peptides from the selected fractions (<3 kDa, 3–10 kDa, <10 kDa and >10 kDa) were analyzed by LC-MS/MS and the amino acid sequences of the peptides were identified (). The <10 kDa and <3 kDa fractions had better cryoprotective abilities than the other two fractions (>10 kDa and 3–10 kDa). The primary peptide sequences of the <10 kDa fraction were GVDNPGHPFIM, GVDNPGHPFIMT, and IITNWDDMEK, which made up 15.41% of the fraction content. In the <3 kDa fraction, the peptide (GVDNPGHPFIM, GVDNPGHPFIMT, and IITNWDDMEK) content accounted for more than 46% of the fraction. These three peptides were concentrated after further ultrafiltration by 3 kDa Ultracel-PL regenerated cellulose membranes.
Table 2. The amino acid sequences of the identified peptides and content in four membranes separated fractions
The peptides (GVDNPGHPFIM, GVDNPGHPFIMT, and IITNWDDMEK) contained 11, 12 and 10 amino acid residues, and had molecular weights of 1345 Da, 1526 Da and 1425 Da, respectively. These short peptides can easily adsorb to the surface of ice and inhibit ice crystallization, thus acting as effective cryoprotective agents, which agrees well with previous conclusions.[Citation26]
The peptides (GVDNPGHPFIM and GVDNPGHPFIMT) contained more Gly- and Pro-residues and -Gly-X-Pro- sequences. Two antifreeze peptides from snow fleas were also rich in Gly, and (–Gly–X–X–) tripeptide repeat sequences facilitated hydrogen bond formation by providing molecules with high degrees of flexibility.[Citation27,Citation28] Gly-Pro-X is also a key peptide sequence offering cryoprotective activity,[Citation10] that can offer crucial information for the structure–efficiency relationship of antifreeze peptides. Whether the (Gly-X-Pro-) sequence in our purified peptides contributed to the cryoprotective effect, like Gly-Pro-X, requires further analysis.
Furthermore, the peptide (IITNWDDMEK) contained more acidic amino acids (Glu and Asp), basic amino acid (Lys) residues, and Ile residues which have a long side chain and can cause steric hindrance. These make the hydrogen bonding between the peptide and the ice crystal more stable.
Further studies will be focused on the synthesis of the three peptides sequences and characterization of the cyroprotective ability and mechanism. Whether the membrane separated fraction (<3 kDa) can be used to replace purified peptides in industrial uses is worth consideration for economic reasons.
Conclusion
The results have revealed that adding FPH-30 effectively protects the surimi matrix during freeze-thaw treatments by a different cryoprotective mechanism from that of SuSo. After further purification of FPH-30 using ultrafiltration membranes of 10 kDa and 3 kDa, the obtained <3 kDa fraction showed the best cryoprotective effects. The main peptide sequences of this fraction were GVDNPGHPFIM(T) with a molecular weight of 1345 Da (1526 Da) and IITNWDDMEK with a molecular weight of 1425 Da. The results strongly support that silver carp are a potential source of cryoprotectant peptides for industrial applications. Further studies will be focused on the synthesis of the three peptide sequences discovered here and characterization of the cyroprotective ability and mechanism.
Additional information
Funding
References
- FAO. FAO-yearbook Of Fishery Statistics: Aquaculture Production; Food and Agriculture Organization of the United Nations: Rome, 2016; pp 30.
- Cheung, I. W. Y.; Liceaga, A. M.; Li-Chan, E. C. Y. Pacific Hake (Merluccius Productus) Hydrolysates as Cryoprotective Agents in Frozen Pacific Cod Fillet Mince. J. Food Sci. 2009, 74(8), C588–C594. DOI: 10.1111/j.1750-3841.2009.01307.x.
- Korzeniowska, M.; Cheung, I. W. Y.; Li-Chan, E. C. Y. Effects of Fish Protein Hydrolysate and Freeze–Thaw Treatment on Physicochemical and Gel Properties of Natural Actomyosin from Pacific Cod. Food Chem. 2013, 138, 1967–1975.
- Damodaran, S.;. Inhibition of Ice Crystal Growth in Ice Cream Mix by Gelatin Hydrolysate. J. Agric. Food Chem. 2007, 55, 10918–10923. DOI: 10.1021/jf0724670.
- Harnedy, P. A.; FitzGerald, R. J. Bioactive Peptides from Marine Processing Waste and Shellfish: A Review. J. Funct. Foods. 2012, 4, 6–24. DOI: 10.1016/j.jff.2011.09.001.
- Karnjanapratum, S.; Benjakul, S. Cryoprotective and Antioxidative Effects of Gelatin Hydrolysate from Unicorn Leatherjacket Skin. Int. J. Refrig. 2015, 49, 69–78. DOI: 10.1016/j.ijrefrig.2014.09.016.
- Kittiphattanabawon, P.; Benjakul, S.; Visessanguan, W.; Shahidi, F. Cryoprotective Effect of Gelatin Hydrolysate from Blacktip Shark Skin on Surimi Subjected to Different Freeze-Thaw Cycles. LWT - Food Sci. Technol. 2012, 47, 437–442. DOI: 10.1016/j.lwt.2012.02.003.
- Mueller, J. P.; Liceaga, A. M. Characterization and Cryoprotection of Invasive Silver Carp (Hypophthalmicthys Molitrix) Protein Hydrolysates. J. Aquat. Food Prod. Technol. 2014. DOI: 10.1080/10498850.2013.832452.
- Nikoo, M.; Benjakul, S.; Ehsani, A.; Li, J.; Wu, F. F.; Yang, N.; Xu, B. C.; Jin, Z. Y.; Xu, X. M. Antioxidant and Cryoprotective Effects of a Tetrapeptide Isolated from Amur Sturgeon Skin Gelatin. J. Funct. Foods. 2014, 7, 609–620. DOI: 10.1016/j.jff.2013.12.024.
- Wang, S. Y.; Zhao, J.; Chen, L.; Zhou, Y. F.; Wu, J. H. Preparation, Isolation and Hypothermia Protection Activity of Antifreeze Peptides from Shark Skin Collagen. LWT - Food Sci. Technol. 2014, 55(1), 210–217. DOI: 10.1016/j.lwt.2013.07.019.
- Wu, J. H.; Rong, Y. Z.; Wang, Z. W.; Zhou, Y. F.; Wang, S. Y.; Zhao, B. Isolation and Characterisation of Sericin Antifreeze Peptides and Molecular Dynamics Modelling of Their Ice-Binding Interaction. Food Chem. 2015, 174, 621–629. DOI: 10.1016/j.foodchem.2014.11.100.
- Li, L.; Wu, J. H.; Zhang, L.; Chen, X.; Wu, Y.; Liu, J. H.; Geng, X. Q.; Wang, Z. W.; Wang, S. Y. Investigation of the Physiochemical Properties, Cryoprotective Activity and Possible Action Mechanism of Sericin Peptides Derived from Membrane Separation. LWT- Food Sci. Technol. 2017, 77, 532–541. DOI: 10.1016/j.lwt.2016.12.004.
- Cao, H.; Zhao, Y.; Zhu, Y. B.; Xu, F.; Yu, J. S.; Yuan, M. Antifreeze and Cryoprotective Activities of Ice-Binding Collagen Peptides from Pig Skin. Food Chem. 2016, 194, 1245–1253. DOI: 10.1016/j.foodchem.2015.08.102.
- Liu, Y. L.; Li, X. H.; Chen, Z. J.; Yu, J.; Wang, F. X.; Wang, J. H. Characterization of Structural and Functional Properties of Fish Protein Hydrolysates from Surimi Processing By-Products. Food Chem. 2014, 151, 459–465. DOI: 10.1016/j.foodchem.2013.11.089.
- Adler-Nissen, J.;. Enzymic Hydrolysis of Food Protein; Elsevier Applied Science: London, 1986; pp 113–169.
- Lowry, O. H.; Rosebrough, N. J.; Farr, A. L.; Randall, R. J. Protein Measurement with the Folin Phenol Reagent. J. Biol. Chem. 1951, 193, 265–275.
- Wang, L.; Xiong, G. Q.; Peng, Y. B.; Wu, W. J.; Li, X.; Wang, J.; Qiao, Y.; Liao, L.; Ding, A. Z. The Cryoprotective Effect of Different Konjac Glucomannan (KGM) Hydrolysates on the Glass Carp (Ctenopharyngodon Idella) Myofibrillar during Frozen Storage. Food Bioprocess. Tech. 2014, 7, 3398–3406. DOI: 10.1007/s11947-014-1345-3.
- Somjit, K.; Ruttanapornwareesakul, Y.; Hara, K.; Nozaki, Y. The Cryoprotectant Effect of Shrimp Chitin and Shrimp Chitin Hydrolysate on Denaturation and Unfrozen Water of Lizardfish Surimi during Frozen Storage. Food Res. Int. 2005, 38, 345–355. DOI: 10.1016/j.foodres.2004.10.017.
- Wang, W. L.; Chen, M. S.; Wu, J. H.; Wang, S. Y. Hypothermia Protection Effect of Antifreeze Peptides from Pigskin Collagen on Freeze-Dried Streptococcus Thermophiles and Its Possible Action Mechanism. LWT - Food Sci. Technol. 2015, 63(2), 878–885. DOI: 10.1016/j.lwt.2015.04.007.
- Lian, P.; Lee, C.; Hufnagel, L. Physicochemical Properties of Frozen Red Hake (Urophycis Chuss) Mince as Affected by Cryoprotective Ingredients. J. Food Sci. 2006, 65(7), 1117–1123. DOI: 10.1111/j.1365-2621.2000.tb10249.x.
- Careche, M.; Li-Chan, E. C. Y. Structural Changes in Cod Myosin after Modification with Formaldehyde or Frozen Storage. J. Food Sci. 1997, 62(4), 717–723. DOI: 10.1111/j.1365-2621.1997.tb15443.x.
- Herrera, J. J.; Pastoriza, L.; Sampedro, G. A DSC Study on the Effects of Various Maltodextrins and Sucrose on Protein Changes in Frozen-Stored Minced Blue Whiting Muscle. J. Sci. Food Agri. 2001, 81(4), 377–384. DOI: 10.1002/1097-0010(200103)81:4<377::AID-JSFA820>3.0.CO;2-0.
- Ko, W. C.; Shi, H. Z.; Chang, C. K.; Huang, Y. H.; Chen, Y. A.; Hsieh, C. W. Effect Of Adjustable Parallel High Voltage On Biochemical Indicators And Actomyosin Ca2+-ATPase From Tilapia (Orechromis Niloticus). LWT - Food Sci. Technol. 2016, 69, 417–423. DOI: 10.1016/j.lwt.2016.01.074.
- Ko, W. C.; Jao, C. L.; Hwang, J. S.; Hsu, K. C. Effect of High-Pressure Treatment on Processing Quality of Tilapia Meat Fillets. J. Food Eng. 2006, 77(4), 1007–1011.
- Wu, L. F.; Jiang, A. M.; Jing, Y. S.; Zheng, Y. G.; Yan, Y. P. Antioxidant Properties of Protein Hydrolysate from Douchi by Membrane Ultrafiltration. Int. J. Food Prop. 2017, 20(5), 997–1006. DOI: 10.1080/10942912.2016.1192644.
- Kim, J. S.; Damodaran, S.; Yethiraj, A. Retardation of Ice Crystallization by Short Peptides. J. Phys. Chem. A. 2009, 113, 4403–4407. DOI: 10.1021/jp8110748.
- Graham, L.; Davies, P. L. Glycine-Rich Antifreeze Proteins from Snow Fleas. Science. 2005, 310, 461. DOI: 10.1126/science.1115145.
- Lin, F. H.; Graham, L. A.; Campbell, R. L.; Davies, P. L. Structural Modeling of Snow Flea Antifreeze Protein. Biophys. J. 2007, 92, 1717–1723.