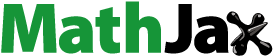
ABSTRACT
The widely-studied interaction between starch and phenolic compounds remains poorly understood. This study prepared three different starch–apple polyphenol (AP) complexes, and studied their rheological, pasting, and structural properties. The curves of starch-AP complexes and raw starches fitted the power law model, and R2 ranged from 0.968 to 0.999. AP addition significantly affected the dynamic viscoelasticity of starches. Creep and pasting properties further indicated the effect of APs on starches. The results indicated that APs affect the properties of starches at different levels. This paper provides a basis for the production of functional foods with starch-AP complexes.
Introduction
Apples are a rich source of polyphenols. Polyphenols are considered “essential to a long life”[Citation1] and have antioxidant, anti-cancer, and antiviral properties, can reduce blood pressure, relieve allergies, and prevent dental caries and heart attack. Apple polyphenols (APs) can be classified into flavonoids and phenolic acids. Flavonoids are further classified into dihydrochalcones, flavanols, and anthocyanins, while phenolic acids are classified into hydroxyl benzoic acids and hydroxycinnamic acids. Alonso-Salces[Citation2] used high-performance liquid chromatography mass spectrometry with diode-array detection and detected 30 types of polyphenols in apples. Lu and Yeap[Citation3] studied the composition and properties of freeze-dried Gala apple pomace and reported that it mostly consisted of chlorogenic acid, caffeic acid, catechin, epicatechin, quercetin glycoside, and phloretin glycoside.
Polyphenols are often used as additives in the food industry; moreover, the public is showing increasing interest in phytochemicals extracted from plants as nutritional supplements. Byproducts of the processing of almonds (used for baking products), have also been studied for their polyphenols.[Citation4] Olive extract has also been added to starch–gluten frying matrices to study the effect of the frying method on the change of both polyphenol contents and antioxidative capacity.[Citation5] Abdel-Aal[Citation6] added ferulic acid to bread dough since both controlled and fortified wholegrain bakery products contain considerable levels of free and bound phenolic acids. Overall, polyphenols are becoming increasingly popular as nutritional fortifiers and supplements.
Starch is the second-largest renewable resource and is widely distributed in nature. It is the major dietary component and the main source of energy for humans, and presents an important contribution to food texture and the resulting blood glucose response. Starch is a polysaccharide, consisting of glucose subunits, which are interconnected by α-1,4 and α-1,6 bonds. Under specific conditions, hydrogen bonding causes chains to coil and form complexes with other materials. This leads to the formation of a left-handed helical structure and the emergence of composite systems with unique functional properties, which improve the processing and quality characteristics.
Over recent years, the interaction between starch and phenolic compounds has become a research hotspot, due to its influence on food properties and nutrition.[Citation7] However, with regard to Aps in particular, this interaction remains poorly understood. Amylose and phenolic compounds interact with non-covalent bonds and form a V-type structure, which can be classified as either inclusion complex forming or non-inclusion complex forming.[Citation7] Hydrophobic interactions, hydrogen bonds, and both electrostatic and ionic interactions have been reported to be part of these structures.[Citation8] Different types of polyphenols not only alter the physical properties of starch such as gelatinization, gelling, and retrogradation (as measured by differential scanning calorimetry[Citation9–Citation13]), but also both in vivo and in vitro starch digestion.[Citation14–Citation17]
Therefore, the changes caused by of AP addition on the physicochemical properties of starch need to be explored. The present study focused on the impact of APs on native maize starch (NMS), native wheat starch (NWS), and native rice starch (NRS) to provide a basis for future research.
Materials and methods
Materials
APs (apparent purity: 95.0%) contained procyanidins (~43.3%), chlorogenic acid (~20.0%), phlorizin and phloretin (~11.7%), anthocyanins (~5.4%), p-coumaric acid (~4.5%), hydrochalcone (~4.3%), and [−]-epicatechin, [+]-catechin, and gallic acid (~5.8%), were purchased from Hubei Jusheng Technology Co., Ltd. (Wuhan, Hubei, China). NMS (moisture content: 18.07%, apparent amylose content: 30.2%), NWS (moisture content: 14.80%, apparent amylose content: 31.0%), and NRS (moisture content: 15.35%, apparent amylose content: 22.6%) were obtained from Dingguo Biological Technology Co., Ltd. (Shenyang, Liaoning, China). Methyl silicone oil and potassium bromide were both obtained from Sinopharm Chemical Reagent Co., Ltd. (Shanghai, China).
Rheological measurements
The experimental method followed Zhu and Wang.[Citation10] The tests were conducted using a rheometer (DHR; TA Instruments, New Castle, DE, USA), and a metal parallel plate with a radius of 20 mm was utilized. Samples were put on the platform of the rheometer and trimmed to ensure that they did not extend beyond the edge of the plate. The gap was set to 1000 μm. To minimize water evaporation during the experiments, methyl silicone oil was applied surrounding the sample.[Citation18] For every test, a fresh sample was used.
Steady shear viscosity measurements
Starch–AP samples were prepared from 60 mg of starch with different proportions of APs (60:0, 60:1, 60:3, 60:6, 60:12, 60:24, 60:36) and these samples were mixed with 1.2 mL H2O in beakers. All samples were stored by sealing the beakers with plastic wrap to prevent polluting samples with impurities. Temperature increased from 25°C to 95°C, and decreased from 95°C to 25°C, at a speed of 10°C/min, and the parallel plate was set to accelerate the shear rate from 0.1 to 300 s−1. The space between the parallel plate and the platform was programmed to 1000 μm. The shear stress was obtained as a function of the shear rate. The following power law model was utilized to express the flow behavior of starch:
The behavior of non-Newtonian fluids was expressed via EquationEquation (1)(1)
(1) [Citation19], where σ represents the shear stress (Pa), γ represents the shear rate (s−1), K represents the consistency index (Pa·sn), and n represents the flow behavior index.
Dynamic oscillatory analysis
Dynamic oscillatory analysis was used to obtain both the storage modulus (G’) and the loss modulus (G”) of samples. Starch–AP complexes were prepared using 200 mg of starch mixed with different proportions of APs (200:0, 200:0.5, 200:1, 200:2, 200:4, 200:8, and 200:16) in 1.0 mL H2O in beakers. All samples were stored by sealing the beakers with plastic wrap to prevent polluting samples with impurities. Temperature increased from 25°C to 95°C, and decreased from 95°C to 25°C, at a speed of 10°C/min. The samples were used to measure the frequency sweep as follows: temperature, 25°C; angular frequency, 0.1–10 Hz; strain, 2%.
Creep-recovery measurement
The samples were prepared as described in the section on dynamic oscillatory analysis. 30 pa of constant stress was applied to the samples for 120 sec and removing the stress to strain recovery for 180 sec, which was set to obtain the data of creep and recovery strain.[Citation19] The temperature for this test was 25°C.
Pasting properties
Samples were treated as described by Zhu[Citation9] with modifications. All measurements were taken by a rapid viscosity analyzer (RVA) (Perten Instruments, Hägersten, Sweden). Starch (3.0 g, dry basis) and APs, at amounts of 0, 30, 60, 90, 120, 150, and 200 mg, were placed in the RVA canister, and then, distilled water was added to arrive at 28 g of starch–AP complex. The mixtures were manually homogenized using a plastic board to prevent agglomeration before tests begin. Each sample was stored at 50°C for 1 min, then, the temperature was increased to 95°C for 3.75 min, kept at 95°C for 2.5 min, cooled to 50°C for 3.75 min, and lastly kept at 50°C for 2 min. The pasting properties are defined by the peak viscosity (PV), peak time (PT), hot paste viscosity (HPV), final viscosity (FV), breakdown (BD), and retrogradation value (RV).
Fourier transform infrared (FTIR) analysis
Samples were prepared using a rheometer with 200 mg of starch and three proportions of APs (200:0, 200:2, and 200:20) mixed with 1.0 mL H2O. Temperature increased from 25°C to 95°C, and decreased from 95°C to 25°C, at a speed of 10°C/min. The complexes were then freeze-dried and analyzed using a Nicolet IR200 FTIR spectrometer (Thermo Electron Corp, Waltham, MA, USA.). Then, 2 mg of the sample was stirred evenly with 198 mg of potassium bromide and the mixture was then compressed. Samples were measured from 4000 cm−1 to 400 cm−1.
Scanning electron microscopy (SEM)
With the aim to observe the three types of starches and their complexes with APs under micro conditions, SEM was conducted using an EVO MA 15 microscope (ZEISS, Jena, Germany). Samples were prepared as described in the section on FTIR analysis. The samples were fixed on thin, conductive, double-sided adhesive tape, and were gold-coated via vacuum sputtering. The magnification was 500 ×, and the acceleration voltage was 10 kV.
Statistical analysis
All the tests were performed in triplicate in a completely randomized design. The obtained data were analyzed using SPSS 18.0 (IBM, Armonk, New York, USA) and MATLAB 2014 Ra (Mathworks, MA, USA). P < .05 represents a significant difference between the means of samples.
Results and discussion
Flow behavior
The rheological model reflects the properties and regularities and accurately describes the stress-strain relationship of fluids, thus enabling the prediction of changes in the viscosity as a result of the application of any shear stress to a fluid.[Citation20] The power law formula (EquationEquation (1)(1)
(1) ) was used to express the rheological characteristics of the starch–AP complexes. When the rheological characteristics of starch–AP complexes and those of the raw starches were appropriate by power law (), R2 ranged from 0.968 to 0.999, indicating that the power law was appropriate in this situation. The shear stresses (σ) of the NMS, NWS, and NRS samples were significantly enhanced when a high concentration of APs powder was added (P < .05). However, no obvious rules were found for shear stress of starch with various concentrations of APs except for NWS. The curves of complexes and raw starches were non-linear, protruded past the shear stress axis, and exhibited typical non-Newtonian fluid characteristics.[Citation21] In , the rheological curve of the shear rate from 0 to 300 s−1 is called the “upward curve”, while that from 300 to 0 s−1 is called the “downward curve”. The flow behavior indices (n) of all samples ranged between 0 and 1 (). These results indicate that the samples fitted the property of shear-thinning, and were identified as “pseudoplastic fluid”, whereby a shear-induced molecular deformation is accompanied by changes in the hydrodynamic interactions as well as the rotation and deformation of starch-AP complexes. A decreasing value of n indicates a stronger internal structure of the fluid. According to and , the n values of the complexes were lower than those of raw starches, and AP addition increased the K values of the complexes. This may be due to the addition of polyphenols in starch, which changes the arrangement of molecules and forms a more stable complex via the hydrogen bonding force. This results in a smaller degree of stretching deformation of the starch granular structure under external force. Therefore, the consistency coefficient increased and the rheological index decreased.
Table 1. Power law models for steady shear properties of three types of starch in the presence of APs
Dynamic viscoelastic properties
Dynamic viscoelastic measurements are typically used to illustrate the structure of viscoelastic materials.[Citation19] The G’ and G” of the complexes and raw starch increased with increasing frequency, and G’ clearly exceeded G”. Moreover, there was no cross between the two moduli in the test procedure of frequency (data and figures of loss modulus are not shown in this paper), indicating that all samples were elastic solids.
Overall, the addition of APs significantly affected the dynamic viscoelasticity of NMS, NWS, and NRS ( and ). Independent of heating and cooling, the storage moduli of NMS–AP and NWS–AP complexes were lower than those of raw starches, while those of NRS–AP complex were higher than those of raw starches. The APs also affected the TGʹmax value of starch, which increased for NMS, but decreased for both NWS and NRS. The polyphenol concentration exerted different effects on the storage moduli of complexes of various starches. This showed that the dynamic viscoelasticity of complexes not only depends on polyphenols, but also on the type of starch.[Citation22]
Table 2. Dynamic rheological properties of three types of starch in the presence of APs
Figure 2. Dynamic rheological curves of frequency sweep of three types of starches and their complexes with APs at 25°C
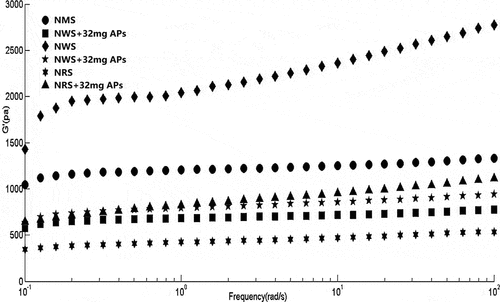
Both crosslinking of APs and starch and the effective linking of starch granules exerted a significant influence on the dynamic viscoelastic properties of complexes and raw starches.[Citation23] The increase in the Gʹ of NRS–AP complexes proved that the gel properties of the starch were enhanced by the interaction with APs, thus forming a stronger net. However, several studies reported contrasting results, with a decrease in the Gʹ value of the NMS–AP and NWS–AP complexes. Clearly, starches and starch–AP complexes are only slightly dependent on the frequency, but a higher frequency led to a larger G’. These results were similar to previous studies.[Citation9,Citation24] The effect of APs differed depending on the type of starch, likely because the structure of each starch determined the influence of APs on its dynamic viscoelasticity.
Creep
The results of creep-recovery experiments of APs on NMS, NWS, and NRS under constant stress conditions are shown in and . The first 180 sec form the stress-applied stage, and the second 120 sec form the stress-restored stage. shows that the creep of the complexes and raw starch can be divided into three stages under loading stress: the instantaneous deformation stage, the delayed creep stage, and the plastic deformation stage. This characteristic forms the typical viscoelastic creep-recovery experimental feature.[Citation25] According to , the creep strain values of the NMS–AP and NWS–AP complexes exceeded that of raw starch for high AP concentrations, while the creep strain of the NRS–AP complex was slightly lower than that of raw starch. The opposite was true at low APs concentrations. No apparent trends of APs on creep strain and recovery strain of starch were found, which indicated that the effect of polyphenols on starch is more complex.
Pasting
As shown in and , the addition of APs to NMS did not noticeably affect the PV, while a remarkable change in the PV of the NWS and NRS samples was found when APs were added (P < .05), from 2439 and 2356 cp to 2873 and 2590 cp, respectively. This is likely due to an increase of temperature, expansion of amylopectin, and molecular dissolution of amylose in NRS–AP and NWS–AP systems; the apparent viscosity increased rapidly. However, in the NMS–AP system, APs may have been inserted between NMS molecules, thus preventing intermolecular entanglement and weakening the intermolecular hydrogen bonds between starch molecules, which leads to a lower PV.
Table 3. Pasting properties of three types of starch in the presence of APs
The stability of the hot starch paste is reflected by the breakdown value, which is a metric for the difference between peak and trough viscosities ( and ). The higher the breakdown value, the lower the thermal stability.[Citation26] The breakdown value of the NMS–AP complex was lower at low AP concentrations and slightly higher at high AP concentrations compared to that of NMS. The breakdown values of the NWS–AP and NRS–AP complexes were both higher than those of the corresponding raw starches, which is likely due to the increase in the viscosity of the complex, which resulted in a higher shear force, which acts on the starch particles, indicating that the particles could be easily deformed and damaged.[Citation27]
The RV value represents the difference between the HPV and the FV. With increasing RV, aging has been shown to occur more easily.[Citation28] Retrogradation occurs due to the rearrangement of the amylose chain. The retrogradation value of the NMS–AP complex was lower than that of raw NMS, suggesting that the complex does not age easily. The NWS–AP and NRS–AP complexes both had RV higher than those of raw starch, suggesting that they age easily and are more gelatinous ( and ). These results further illustrate that APs exert a complicated effect on starch, which is not only dependent on the type of starch but also the concentration of APs.
FTIR analysis
Infrared (IR) spectra mainly reflect a strong polar group and a change of the dipole moment of the group vibration.[Citation29] With increasing strength of the group vibration, the dipole moment changes to a greater extent, and the infrared absorption peak shifts upward. The IR spectra of the starch–AP complexes are shown in . When raw starch is heated, water molecules enter starch granules, thus enhancing intramolecular hydrogen bonding. The band at 2929 cm−1 represents the -CH2 asymmetric stretching vibration, and that at 3400 cm−1 represents the -OH symmetric stretching vibration.[Citation30] Both absorption peaks of complexes and heated starch shifted toward higher wavenumbers.
Figure 5. Fourier transform infrared spectra of NMS + 10% APs (a1), NMS + 1% APs (a2), heated NMS (a3), NMS (a4), NWS + 10% APs (b1), NWS + 1% APs (b2), heated NWS (b3), NWS (b4), NRS + 10% APs (c1), NRS + 1% APs (c2), heated NRS (c3), NRS (c4), and APs (for abbreviations, please see legend of )
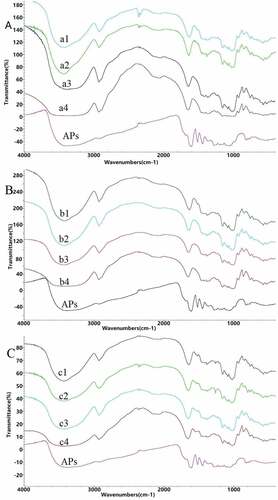
Comparing the spectra of raw starch and the heated starch showed that those of the three starch–AP complexes exhibited no new absorption peaks, indicating that no new covalent bonding had occurred. In the AP spectrum, the band at 1444 cm−1 corresponded to the asymmetric absorption of hydrocarbons, indicating the presence of -CH2 and -CH, while bands at 1515 cm−1 and 1605 cm−1 corresponded to the C-C skeleton stretching vibration absorption.[Citation31] An obvious reduction affected these absorption peaks in the complex, indicating non-covalent binding may be attributed to a combination of APs and starch via both hydrophobic interaction and hydrogen bonding. Starch chains have a helical structure, in which hydrophobic groups form helical hydrophobic cavities. The APs enter these cavities through hydrophobic interactions, then forming complexes, which, to some extent, inhibit the characteristic absorption peaks of APs.
SEM observations
The SEM images of gelatinized freeze-dried powders of the starch–AP complexes () verified the different influences of the APs on the micromorphologies of the three starches. NMS presented an irregular lumpy or flaky structure, while NWS and NRS both had net-like structures. With the addition of APs, however, the NMS also adopted a net-like structure, and the network structure tightened. In contrast, addition of APs to NWS resulted in a relatively loose structure, while the net structure of the NRS–AP complex was more compact. Previous research showed that starch and phenolic compounds can form V-type embedding compounds and non-embedding compounds.[Citation7,Citation24] It is likely that the interaction between starch and various polyphenols is different.[Citation8] Zhu et al.[Citation9] added phytochemical extracts to NWS and found that it formed a loose gel matrix with phytochemical extracts, while the control showed a more compact microstructure. The types of polyphenol may affect the positions and quantities of functional groups in the starch-polyphenol complex, thus hydrogen bond, hydrophobic bond, and van der Waals force are active between starch and polyphenols at varying degrees. In addition, the type, molecular weight, and crystalline form of starch may also affect the interaction between starch-polyphenol complex. Based on these assumptions, further experiments will be designed and conducted to verify the mechanism of the interaction between starch and polyphenols.
Conclusion
The addition of APs produced effects of different starches. For all three tested starches, the curves of starch-AP complexes and raw starches fitted the power law model. APs increased both the shear stress and consistency index, while decreasing the flow behavior index at appropriate concentration. Dynamic viscoelastic, creep, and pasting properties further showed that AP concentration affected different starches at various degrees. Structural characterizations and micrographs of the complexes were analyzed using FTIR spectroscopy and SEM. The results suggested that the APs interacted with the starch by noncovalent binding, and that the micromorphologies of starches were changed by the addition of APs, by altering their rheological and pasting properties. The conclusion is that not only concentrations of APs, but also the types of starch affected the properties of the starch-AP complex. Thus, an appropriate starch–AP complex should be selected depending on the need for commercialization.
Acknowledgments
This research was supported by the National Key R & D Program of China (2016YFD0400203).
Additional information
Funding
References
- Phan, A. D.; Netzel, G.; Wang, D.; Flanagan, B. M.; D’Arcy, B. R.; Gidley, M. J. Binding of Dietary Polyphenols to Cellulose: Structural and Nutritional Aspects. Food Chem. 2015, 171, 388–396. DOI: 10.1016/j.foodchem.2014.08.118.
- Alonso-Salces, R. M.; Ndjoko, K.; Queiroz, E. F.; Ioset, J. R.; Hostettmann, K.; Berrueta, L. A.; Gallo B.; Vicente F. On-line Characterisation of Apple Polyphenols by Liquid Chromatography Coupled with Mass Spectrometry and Ultraviolet Absorbance Detection. J. Chromatogr. A.2004, 1046(1–2), 89. DOI: 10.1016/j.chroma.2004.06.077.
- Lu, Y.; Foo, L. Y. Identification and Quantification of Major Polyphenols in Apple Pomace. Food Chem. 1997, 59(2), 187–194. DOI: 10.1016/S0308-8146(96)00287-7.
- Pasqualone, A.; Laddomada, B.; Spina, A.; Todaro, A.; Guzmàn, C.; Summo, C.; Mita G.; Giannone V. Almond By-products: Extraction and Characterization of Phenolic Compounds and Evaluation of Their Potential Use in Composite Dough with Wheat Flour. LWT - Food Sci. Technol. 2018, 89, 299–306. DOI: 10.1016/j.lwt.2017.10.066.
- Urzúa, C.; González, E.; Dueik, V.; Bouchon, P.; Giménez, B.; Robert, P. Olive Leaves Extract Encapsulated by Spray-drying in Vacuum Fried Starch-gluten Doughs. Food Bioprod. Process. 2017, 106, 171–180. DOI: 10.1016/j.fbp.2017.10.001.
- Abdel-Aal, E. S. M.; Rabalski, I. Effect of Baking on Free and Bound Phenolic Acids in Wholegrain Bakery Products. J. Cereal Sci. 2013, 57(3), 312–318. DOI: 10.1016/j.jcs.2012.12.001.
- Zhu, F.;. Interactions between Starch and Phenolic Compound. Trends Food Sci. Technol. 2015, 43(2), 129–143. DOI: 10.1016/j.tifs.2015.02.003.
- Bordenave, N.; Hamaker, B. R.; Ferruzzi, M. G. Nature and Consequences of Non-covalent Interactions between Flavonoids and Macronutrients in Foods. Food Funct. 2014, 5, 18–34. DOI: 10.1039/C3FO60263J.
- Zhu, F.; Cai, Y. Z.; Mei, S.; Harold, C. Effect of Phytochemical Extracts on the Pasting, Thermal, and Gelling Properties of Wheat Starch. Food Chem. 2009, 112(4), 919–923. DOI: 10.1016/j.foodchem.2008.06.079.
- Zhu, F.; Wang, Y. J. Rheological and Thermal Properties of Rice Starch and Rutin Mixtures. Food Res. Int. 2012, 49(2), 757–762. DOI: 10.1016/j.foodres.2012.09.031.
- Barros, F.; Awika, J. M.; Rooney, L. W. Interaction of Tannins and Other Sorghum Phenolic Compounds with Starch and Effects on in Vitro Starch Digestibility. J. Agric. Food Chem. 2012, 60, 11609–11617. DOI: 10.1021/jf3034539.
- Wu, Y.; Lin, Q.; Chen, Z.; Xiao, H. The Interaction between Tea Polyphenols and Rice Starch during Gelatinization. Food Sci. Technol. Int. 2011, 17, 569–577. DOI: 10.1177/1082013211430294.
- Chai, Y.; Wang, M.; Zhang, G. Interaction between Amylose and Tea Polyphenols Modulates the Postprandial Glycemic Response to High-amylose Maize Starch. J. Agric. Food Chem. 2013, 61, 8608–8615. DOI: 10.1021/jf402821r.
- McCue, P.; Vattem, D.; Shetty, K. Inhibitory Effect of Clonal Oregano Extracts against Porcine Pancreatic Amylase in Vitro. Asia Pac. J. Clin. Nutr. 2004, 13, 401–408. PMID: 15563448
- Vadivel, V.; Biesalski, H. K. Contribution of Phenolic Compounds to the Antioxidant Potential and Type Ii Diabetes Related Enzyme Inhibition Properties of Pongamia Pinnata, L. Pierre Seeds. Process Biochem. 2011, 46(10), 1973–1980. DOI: 10.1016/j.procbio.2011.07.007.
- Törrönen, R.; Kolehmainen, M.; Sarkkinen, E.; Poutanen, K.; Mykkänen, H.; Niskanen, L. Berries Reduce Postprandial Insulin Responses to Wheat and Rye Breads in Healthy Women. J. Nutr. 2013, 143(4), 430–436. DOI: 10.3945/jn.112.169771.
- Liu, J.; Wang, M.; Peng, S.; Zhang, G. Effect of Green Tea Catechins on the Postprandial Glycemic Response to Starches Differing in Amylose Content. J. Agric. Food Chem. 2011, 59, 4582–4588. DOI: 10.1021/jf200355q.
- Zhang, X.; Tong, Q.; Zhu, W.; Ren, F. Pasting, Rheological Properties and Gelatinization Kinetics of Tapioca Starch with Sucrose or Glucose. J. Food Eng. 2013, 114, 255–261. DOI: 10.1016/j.jfoodeng.2012.08.002.
- Meng, Y. C.; Sun, M. H.; Fang, S.; Chen, J.; Li, Y. H. Effect of Sucrose Fatty Acid Esters on Pasting, Rheological Properties and Freeze–Thaw Stability of Rice Flour. Food Hydrocolloids. 2014, 40(40), 64–70. DOI: 10.1016/j.foodhyd.2014.02.004.
- Anderson, M. C.; Shoemaker, C. F.; Singh, R. P. Rheological Characterization of Aseptically Packaged Pudding. J. Texture Stud. 2006, 37(6), 681–695. DOI: 10.1111/j.1745-4603.2006.00078.x.
- Chen, H. M.; Fu, X.; Luo, Z. G. Effect of Gum Arabic on Freeze-thaw Stability, Pasting and Rheological Properties of Tapioca Starch and Its Derivatives. Food Hydrocolloids. 2015, 51, 355–360. DOI: 10.1016/j.foodhyd.2015.05.034.
- Nagano, T.; Tamaki, E.; Funami, T. Influence of Guar Gum on Granule Morphologies and Rheological Properties of Maize Starch. Carbohydr. Polym. 2008, 72(1), 95–101. DOI: 10.1016/j.carbpol.2007.07.028.
- Biliaderis, C. G.; Tonogai, J. R. Influence of Lipids on the Thermal and Mechanical Properties of Concentrated Starch Gels. J. Agric. Food Chem. 1991, 39, 833–840. DOI: 10.1021/jf00005a003.
- Karunaratne, R.; Zhu, F. Physicochemical Interactions of Maize Starch with Ferulic Acid. Food Chem. 2016, 199, 372–379. DOI: 10.1016/j.foodchem.2015.12.033.
- Tian, Z.; Duan, L.; Wu, L.; Shen, L.; Li, G. Rheological Properties of Glutaraldehyde-crosslinked Collagen Solutions Analyzed Quantitatively Using Mechanical Models. Mater. Sci. Eng. C Mater. Biol. Appl. 2016, 63, 10–17. DOI: 10.1016/j.msec.2016.02.047.
- Qiu, C.; Li, X. J.; Ji, N.; Qin, Y.; Sun, Q. J.; Xiong, L. Rheological Properties and Microstructure Characterization of Normal and Waxy Corn Starch Dry Heated with Soy Protein Isolate. Food Hydrocolloids. 2015, 48, 1–7. DOI: 10.1016/j.foodhyd.2015.01.030.
- Achayuthakan, P.; Suphantharika, M. Pasting and Rheological Properties of Waxy Corn Starch as Affected by Guar Gum and Xanthan Gum. Carbohydr. Polym. 2008, 71, 9–17. DOI: 10.1016/j.carbpol.2007.05.006.
- Funami, T.; Kataoka, Y.; Omoto, T.; Goto, Y.; Asai, I.; Nishinari, K. Effects of Non-ionic Polysaccharides on the Gelatinization and Retrogradation Behavior of Wheat Starch. Food Hydrocolloids. 2005, 19, 1–13. DOI: 10.1016/j.foodhyd.2004.04.024.
- Liu, R.; Xu, C.; Cong, X.; Wu, T.; Song, Y.; Zhang, M. Effects of Oligomeric Procyanidins on the Retrogradation Properties of Maize Starch with Different Amylose/amylopectin Ratios. Food Chem. 2017, 221, 2010–2017. DOI: 10.1016/j.foodchem.2016.10.131.
- Xijun, L.; Haibo, S.; Li, L.; Hong, H.; Nan, Z. Characterizing the Chemical Features of Lipid and Protein in Sweet Potato and Maize Starches. Starch-Stärke. 2014, 66(3–4), 361–368. DOI: 10.1002/star.201300145.
- He, J. N.; (2014). Research on Polyphenols from Apple: Preparation, Identification and Antioxidant Activity. Doctoral dissertation, Jiangnan University, Wuxi. http://kns.cnki.net/kns/detail/detail.aspx?FileName=1014380931.nh&DbName=CMFD2015