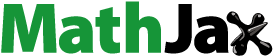
ABSTRACT
Pinctada martensii
protein isolates (PPIs) and polysaccharide complexes of different mixing ratios (10:1, 10:2, 10:3) were prepared at the optimal pH. The characteristics of PPIs and complexes were compared by determination of solubility, emulsifying properties, surface hydrophobicity, thermal properties, rheological behavior, and fourier transform infrared (FT-IR) spectroscopy. Compared with PPIs, the solubility of complexes changed to different degrees, and 10:3 PPI-pectin complex had the highest solubility (10.02 ± 0.68%). The emulsion properties of 10:2 and 10:3 PPI-alginate complexes were significantly higher than produced from other systems. The conjugation of PPI and pectin evidently reduced surface hydrophobicity of PPI. Denaturation temperatures of complexes were generally higher than that of PPIs. The gel network of PPI and complexes was formed within 70–80°C at pH 7.0 and G′ values of complexes were lower than that of PPIs. FT-IR spectroscopy showed O-H stretching of water absorption, C-C and C-O stretching and C-H bending of colloidal polysaccharides were improved in complexes.
Introduction
The pearl oyster (Pinctada martensii) is the main species of pearl culture around the South China Sea. In recent years, the culture scale of pearl oysters has been growing rapidly, and large amounts of oyster meat are left as a typically unused byproduct of the pearl industry.[Citation1] At present, only a small part of the meat is marketed fresh or processed into primary products, and the remainder is directly discarded.[Citation2] This results in the underutilization of oyster meat and environmental pollution. Pearl oyster meat is rich in protein (74.9% on a dry basis) and contains unsaturated fatty acids, trace elements and vitamins, which may represent a valuable protein source for human nutrition.[Citation2,Citation3] Therefore, it is of great economic significance to develop a processing technology to turn this low-value byproduct into food sources.
Aquatic proteins have high nutritional value, and proteins are usually isolated to apply in the food industry to make full use of fishery products. Because of bacterial decay, lipid oxidation and protein oxidation, the thermal and chemical stability of aquatic protein is poorer than that of vertebrate and plant-based proteins.[Citation4] In addition, after aquatic protein is denatured, its functional properties are decreased, which restricts the wide utilization of aquatic proteins in food applications.[Citation5,Citation6] Therefore, to broaden the application of aquatic proteins, some modification methods are used to strengthen or weaken some functional properties to facilitate better utilization in the food industry. Hydrophilic polysaccharide is commonly used as a food additive, which could effectively improve the functional properties of the proteins, such as the thermal stability, viscosity, gel properties, hardness, and emulsification.[Citation5–Citation7] Hydrophilic polysaccharides are mostly natural macromolecular compounds and their derivatives are widely distributed in nature. The polysaccharide structure contains many hydrophilic groups, such as hydroxyl, carboxyl, and amidogen, which can interact with water, proteins, starch, lipid, and other molecules to form a complex with a large molecular mass.[Citation8,Citation9] O’Sullivan et al.[Citation10] reported that the emulsion stability of emulsions prepared with potato protein isolate-κ-carrageenan (PoPI-κ-C) complexes possessed marginally enhanced long-term stability in comparison to emulsions prepared with PoPI alone. Almeida et al.[Citation11] investigated the effect of kappa carrageenan and salt on the thermoreversible gelation of methylcellulose and found that the gel properties of Alaskan cod could be enhanced by the combination of carrageenan and salt solution. Therefore, the protein-polysaccharide complexes present an advantageous performance over individual proteins and have a bright future for application.
The composition, structure, and properties of different polysaccharides vary greatly. Pectins are complex hydrophilic heteropolysaccharides that are typically used as gelling and thickening agents in the food industry.[Citation12] Carrageenans are sulfated polysaccharides extracted from red seaweed that have a strong electrolyte character due to their sulfate groups.[Citation13] Sodium alginate, a linear water-soluble polysaccharide consisting of (1-4)-linked β-D-mannuronic acid (M) and α-L-guluronic acid (G) residues, has been widely used as a thickening or a jellifying agent to improve the water-binding capacity in meat products based on its gelling, viscosity-enhancing and stabilizing properties.[Citation14,Citation15] Pectins, carrageenans, and alginates are anion polysaccharides, and their properties have some common features as well as different characteristics. The interactions between proteins and polysaccharides change the functional properties of the mixed system, which also depend on the protein/polysaccharide ratio. For example, regarding the simultaneous gelation of polysaccharides mixed with proteins, several authors have reported an increase in G’ due to the addition of polysaccharide as a result of a synergistic effect between the biopolymers.[Citation16,Citation17] However, when the concentration of polysaccharide increases above a critical level or there are changes in the pH, a decrease in G’ is observed. This behavior has been attributed to an antagonistic effect between proteins and polysaccharides. Therefore, it is necessary to investigate the effects of different hydrophilic polysaccharides on the functional properties of protein isolates.
Although extensive research has been carried out on protein–polysaccharide systems, most studies were on vertebrate and plant-based proteins. Nevertheless, little information has been reported on the functional properties of aquatic protein–polysaccharide systems. An increasing number of aquatic proteins are used as high-quality resources to serve human needs. However, after the aquatic protein is denatured, its functional properties are decreased, which could not satisfy the various demands of processing. PPI-polysaccharide complexes could improve the functional properties of aquatic proteins, and it is necessary to broadly apply the prospects of aquatic proteins in the food industry. Therefore, the objective of this study was to prepare Pinctada martensii protein isolates (PPIs) and PPI-hydrophilic polysaccharide (pectin, kappa-carrageenan, and alginate) complexes based on electrostatic interactions and to investigate the characteristics of PPIs and PPI-polysaccharide complexes by measuring the solubility, emulsifying properties, surface hydrophobicity, thermal properties, rheological behavior, and secondary structure.
Materials and methods
Materials and chemicals
Pearl oysters (Pinctada martensii, age 24–30 months, approximately 8 cm in length) were obtained from a pearl oyster farm in Leizhou, Guangdong Province, China. After collection, the pearl oysters were cleaned with distilled water and shucked. The meat wrapped in a polyethylene bag was stored at −20°C in the refrigerator until use. Pectin, kappa-carrageenan, and alginate (food-grade) were provided by Yuanda Food Additive Co. (Henan, China). Protease inhibitors were purchased from Sigma–Aldrich Co. (St. Louis, MO, USA). Other chemicals and reagents were of analytical grade or acceptable for human consumption.
Optimum preparation conditions of Pinctada martensii protein isolates (PPIs) and complexes
Pinctada martensii protein isolates (PPIs) were obtained using isoelectric solubilisation/precipitation method developed by Zheng et al.[Citation18] with slight modifications. Pearl oysters (250 g) were thawed in a 4°C refrigerator for 16 hours. Precooling distilled water was added in a proportion of 1:4 (w/v, oysters protein/water), and then the samples were homogenized using an Ultra-Turrax T8 polython homogenizer (IKA Works, Inc, Wilmington, NC, USA) at a speed of 10,000 rpm for 3 min. The whole extraction process was conducted in an ice bath, and protease inhibitors were added to attempt to minimize the effect of endogenous pearl oyster enzymes. The pH of the solution was titrated to pH 11.0 while stirring with 1 M NaOH; then, 5 mL of protease inhibitor (0.1%, w/w) was added to 1.25 kg of the above solution (protease inhibitor has little effect on protein properties duo to low concentration), and stirred for 30 min. The homogenate was centrifuged using the LYNX Superspeed Centrifuge (Thermo Scientific, Waltlham, MA) at 10000 × g, 4°C for 15 min, and then the supernatant (alkali soluble protein) was collected.
The supernatant was adjusted to pH 7.0 with 1 M HCl, and the crude protein content was determined in solution by a Bradford Protein Assay Kit (Cat# 500–0116, Bio-Rad Laboratories, Hercules, CA). The crude protein was prepared at a concentration of 1 mg/ml, and the polysaccharide solution was 2 mg/ml. According to the mass ratio (10:1, 10:2, 10:3 w/w) of crude protein and three hydrophilic polysaccharides, the two solutions were mixed and hydrated for 12 h at 4°C. At the same time, a protein solution without polysaccharide was prepared for the blank control.
Protein-polysaccharide complexes were titrated with different concentrations of HCl (0.05 M HCl > pH 6.00; 0.1 M HCl > pH 3.50; 0.5 M HCl > pH 3.00; 1 M HCl > pH 2.50; 2 M HCl > pH 1.50) to pH values of 3.5, 4.0, 4.5, 5.0, 5.5, 6.0, 6.5, 7.0, and 7.5. Turbidity curves were obtained using a method developed by Stone et al.[Citation19] with slight modifications. The turbidity was measured under 600 nm using a UV/vis spectrophotometer (Thermo Scientific, Waltham, MA), the pH titration curve was drawn, and a blank control without hydrophilic polysaccharide was prepared at the same time.
Preparation of PPI-hydrophilic polysaccharides (pectin, kappa-carrageenan and alginate) complexes
In this work, three PPI-polysaccharide complexes, namely PPI-pectin (PPI-P), PPI-kappa-carrageenan (PPI-κ-CG) and PPI-alginate (PPI-A), were prepared. The preparation of hydration of crude protein and hydrophilic polysaccharide was the same as above. According to the above turbidity curve, the optimum condition of acid precipitation (at the pH condition with the maximum turbidity) was determined to precipitate the protein-hydrophilic polysaccharide complex, precipitating at 4°C for 20 minutes. Then, the mixture was centrifuged at 10000 × g at 4°C for 20 min. The precipitate after centrifugation contained PPI-polysaccharide complexes, and the pH was adjusted to 7.0. At the same time, a blank control without hydrophilic polysaccharide was prepared and preserved under seal after freeze-drying (Eyela FD-5 N vacuum freeze drier, Tokya Rikakikai Co. Ltd., Tokyo, Japan).
Solubility
The solubility (S) of the PPIs and PPI-polysaccharide complexes (freeze-dried samples) was determined by the method of Stone et al.[Citation19] with some modifications. The samples were weighed to 300 mg in 20 mL 0.1 M (pH 7.5) phosphate buffer saline (PBS), and the mixture was adequately hydrated for 30 min using a mini vortexer (Thermo Fisher Scientific, Inc). The solution was centrifuged (2700 × g) for 15 min at room temperature, and the nitrogen content in the supernatant was measured using a micro-Kjeldahl Analyzer (Thermo Scientific, Waltham, MA). At the same time, the nitrogen content of samples was also measured. The solubility of the sample was calculated by the following formulae:
where A1 is the nitrogen content of the samples and A2 is the content of nitrogen in the supernatant.
Emulsifying activity index (EAI) and emulsion stability index (ESI)
The emulsifying activity index (EAI) and emulsion stability index (ESI) were used to measure the emulsification of PPIs and PPI-polysaccharide complexes by the procedure described by Klomklao & Benjakul.[Citation20] Arachis oil (3 ml) and sample solutions (1%, 9 mL, fresh samples) were homogenized (Model T25 basic; IKA-Labortecnik, Selangor, Malaysia) at a speed of 12,000 rpm for 1 min. The 20 μL emulsion was quickly taken from the bottom of the emulsion and added to a 0.1% (w/v, 5 mL) sodium dodecyl sulfate (SDS) solution and shaken well. The absorbance value at 0 min was measured immediately at a wavelength of 500 nm (blank at 0.1% SDS) (A0) using a spectrophotometer (UV-1800, Shimadzu, Kyoto, Japan). After 10 min, the absorbance value (Al) of the samples was measured. EAI and ESI were calculated by the following formulae:
where D is the dilution factor (250), L is the pathlength of the cuvette (1 cm), Φ is the oil volume fraction (1/4) and C is the protein concentration in aqueous phase (g/mL).
Surface hydrophobicity
The surface hydrophobicity of PPIs and PPI-polysaccharide complexes (freeze-dried samples) was measured using a method developed by Timilsena et al.[Citation21] with some modification. For this, 8-anilinno-1-napthalenesulfonic acid (ANS) was used as a hydrophobic fluorescent probe. The samples were diluted with 20 mM tris(hydroxymethyl)aminomethane)-HCl buffer (contained 0.6 M KCl), and the concentration was from 0.2 to 0.8 mg/mL. Twenty microliters of 8.0 mmol/L ANS was mixed with 1.0 mL of samples. The mixture was shaken and kept in the dark for 15 min to react completely. The relative fluorescence intensity of samples with ANS was measured at room temperature using a Hitachi F4500 fluorescence spectrometer (Hitachi, Tokyo, Japan). Excitation and emission wavelength were set at 375 and 485 nm, respectively, with slit widths of 5 nm. The initial slope of fluorescence intensity with increasing protein concentration was calculated as the surface hydrophobicity of the samples.
Thermal properties
Differential scanning calorimetry (DSC) measurements were performed with a Mettler Toledo DSC822 (Greifensee, Switzerland) calorimeter. Each sample (freeze-dried samples) was weighed to approximately 10 mg and placed in an airtight aluminum crucible (Netzsch, Selb, Germany). The samples were heated from 30°C to 120°C at a rate of 5°C/min, and the thermal analysis curve was drawn.
Rheological behavior
The rheological behaviors of PPI and PPI-polysaccharide complexes (not freeze-dried samples) were measured using a Kinexus Rheometer (Malvern Instruments Ltd., Worcestershire, UK) with parallel plates (35 mm). The gap between two plates was set to 1.5 mm. The temperature increased from 20°C to 90°C at a rate of 2°C/min with a test frequency of 1 Hz.[Citation22,Citation23] The storage modulus G’ of the samples was obtained, and the attention was paid to heat preservation in the course of the experiment. The sample was covered with a metal lid to prevent evaporation during the heating process.
Fourier Transform Infrared (FT-IR) spectroscopy
Secondary structures of PPIs and PPI-polysaccharide complexes (freeze-dried samples) were studied using a Thermo Scientific Nicolet 600 FTIR (Thermo Scientific, West Palm Beach, FL). FTIR detection of flakes prepared by mixing KBr with the sample was performed. The sample (approximately 1–3 mg) was mixed with KBr (approximately 100 mg) and then ground, dispersed, and pressed into a sheet. The samples were scanned from 4000 to 400 cm−1 at a data acquisition rate of 4 cm−1 per point. All measurements were performed at room temperature. Background interference was removed.
Statistical analysis
All measurements were performed three times with parallel samples. Statistical analysis was performed using SPSS 18.0 (SPSS Inc., USA). The data shown in the corresponding figures are the mean values with the standard deviation. All data were subjected to analysis of variance (ANOVA) and differences between means were evaluated by Duncan’s multiple range test.
Results and discussion
Optimum preparation conditions of PPIs and complexes
Isolating proteins from raw materials were usually performed by the method of “alkali extraction and acid precipitation,” which utilized the difference of protein solubility in acids and alkali, dissolved the protein in alkali solutions in specific pH and then adjusted the solution pH with acids until the isoelectric point was attained and then separated out the protein isolates.[Citation24] Mixtures of proteins and polysaccharides generally leaded to the formation of electrostatic complexes due to electrostatic attraction between the two.[Citation19] Isoelectric point (pI) of most proteins ranged of pH 4–6, and the conjugation of proteins and polysaccharides can cause varying changes. In this work, the optimal pH for acid precipitation of PPIs and complexes was determined by comparing the turbidity between pH values of 3.5 and 7.5 (-). In the case of PPI alone (), the turbidity curve followed a bell-shaped profile with a maximum value (0.21) at pH 4.5, which indicated that the yield of protein isolates was highest at pH 4.5. Klassen et al.[Citation25] found that the turbidity curve of salt-extracted canola protein isolate (CPI) followed a similar bell-shaped profile. The increase in the turbidity of the solution is thought to be associated with PPI–PPI aggregation, which is due to the reduction in electrostatic charge repulsion between neighboring molecules. The protein at the isoelectric point was present as amphoteric ions, whose total charges approached to zero. The solubility of the protein decreased due to the reduction in repulsive interaction between charges in the solution.
Figure 1. Comparison of the turbidity in PPI(a), PPI-P (b), PPI-κ-CG (c) and PPI-A (d) complexes under different pH conditions. Data represent the mean ± one standard deviation (n = 3).
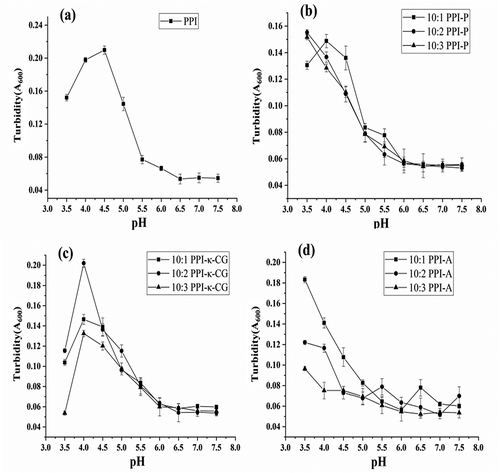
Compared with PPIs, critical pHs (maximum turbidity) of complexes shifted to more acidic pHs. For PPI-P complexes (), the turbidity maximum of the 10:1 PPI-P complex was observed at pH 4.0. At higher mixing ratios (10:2 and 10:3 PPI-P complexes), the turbidity maximum moved toward pH < 3.5. Considering that the solution with excessive acidity might affect the functional properties of complexes, the optimal pHs for acid precipitation of 10:1, 10:2, and 10:3 PPI-P complexes were 4.0, 3.5, and 3.5, respectively. For PPI-κ-CG complexes (), the turbidity maxima of samples with three mixing ratios were observed at pH 4.0, and the optimal pHs for acid precipitation of PPI-κ-CG complexes was 4.0. For PPI-A complexes (), the turbidity maximum entirely moved toward pH < 3.5. The optimal pHs for acid precipitation of PPI-A complexes was 3.5. It was appropriate to take the optimal pH of 3.5 for acid precipitation of PPI-A complexes. The shift in pI values by different complexes may stem from different reasons, such as the charge change of interactional biopolymers, and the change in thickness of electrical double layer around the complexes due to the change in ionic strength of solution, etc.
Solubility
Solubility is one of the main functional properties of proteins and has effects on other functional properties.[Citation19] Polysaccharides contain hydrophilic groups, and protein-polysaccharide conjugation is one of the most effective methods to improve the functional properties of proteins.[Citation26] The interaction between proteins and polysaccharides in food systems can show better performance than either used independently and can affect the flavor, nutrition, texture, and other qualities of the food system.[Citation20] The solubility of PPIs and PPI-polysaccharide complexes is presented in . The solubility of PPIs was (7.68 ± 0.54%), and compared to that of PPIs, the solubility of the complexes changed to different degrees. Among PPI-polysaccharide complexes, the 10:3 PPI-P complex has the highest solubility (10.02 ± 0.68%). Pectin is a hydrophilic colloid, and hydrophilic groups content in proteins was increased due to the conjugation of PPI and pectin.[Citation27] Therefore, the solubility of PPI-P increased compared with that of PPI. For PPI-κ-CG complexes, the solubility of the 10:1 and 10:2 PPI-κ-CG complexes was almost the same as that of PPI, and the solubility of the 10:3 PPI-κ-CG complex was decreased. The solubility of the 10:2 PPI-A complex was the same as that of PPI (P > .05), and the solubility of the 10:1 and 10:3 PPI-A complexes was lower than that of PPI. Some papers reported that alginate and κ-carrageenan could cover hydrophilic groups of the protein in soluble protein-polysaccharide complexes.[Citation28,Citation29] In addition, the bridging flocculation between protein and polysaccharides makes the soluble protein aggregate flocculation, and protein solubility generally decreased with the increasing of polysaccharide concentration. In addition, the stability of whey protein concentrate (WPC) solution can be remained by adding a certain concentration of sodium alginate (0.20%-0.30%), and too high or too low concentration of sodium alginate would produce flocculation duo to the improvement of protein surface hydrophobicity in complex systems, which reduced the protein solubility.[Citation30] The structure of protein molecules in complexes generally unfolded at alkaline pH, exposed more hydrophobic regions which buried inside the parent protein molecule. Following precipitation, its possibly partial refolding at neutral pH.
Figure 2. Solubility of PPI and PPI-polysaccharide complexes. Data represent the mean ± one standard deviation (n = 3). The data were subjected to ANOVA test and differences between means were evaluated by Duncan’s multiple range test. Different superscript letters indicate significant differences (P<0.05).
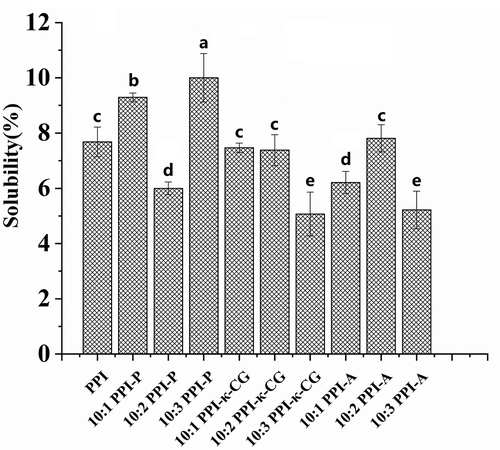
Emulsifying properties
shows the emulsifying activity index (EAI) and emulsion stability index (ESI) of the PPIs and PPI-polysaccharide complexes. The EAI and ESI of PPIs were 33.36 m2/g and 18.09 min. Compared to the values for PPIs, the EAI and ESI of PPI-polysaccharide complexes were changed to different degrees. Most PPI-polysaccharide complexes had higher EAI values than PPI. The EAI of 10:3 PPI-A was highest, and there was no significant difference (P > .05) between 10:3 PPI-κ-CG, 10:2 PPI-A, and 10:3 PPI-A. The values for 10:1 PPI-P, 10:2 PPI-P, and 10:2 PPI-κ-CG remained insignificant (P > .05) change, and the EAI of 10:1 PPI-A was lower than that of PPI. Emulsion stability is the ability of emulsion droplets to remain dispersed without coalescing, flocculating, or creaming and is the ability of an emulsion to remain stable and unchanged.[Citation31] Compared to the ESI of PPI, the ESI values of most of the PPI-polysaccharide complexes remained insignificant (P > .05). The ESIs of 10:2 and 10:3 PPI-A were highest. Overall, the emulsion properties of the PPI-A system were generally higher than those of the other systems.
Table 1. Emulsifying properties of PPI and PPI-polysaccharide complexes.
Surface hydrophobicity
The change of surface hydrophobicity is related to the alteration of protein structure. Surface hydrophobicity is also a vital factor in emulsifying and soluble capacities.[Citation21,Citation32] Surface hydrophobicity of PPI and PPI-polysaccharide complexes were shown in . Compared with PPI, the surface hydrophobicity of PPI-P complexes significantly reduced, with the largest proportional decreases (85.32 to 61.79) noted in the 10:1 PPI-P complex. For PPI-κ-CG complexes, surface hydrophobicity of the 10:1 and 10:2 PPI-κ-CG complexes were slightly lower than that of PPI while the 10:3 PPI-κ-CG complex remained substantially unchanged (p > .05). Surface hydrophobicity of the PPI-A complexes showed little change with no change of the 10:2 PPI-A complexes (p > .05) and an slight increase of the 10:2 and 10:3 PPI-A complexes, which supported the ESI improvement of 10:2 and 10:3 PPI-A complexes. Among three polysaccharides, the addition of pectin could saliently reduce surface hydrophobicity of PPI. The decrease may be ascribed to the shielding effect of a large pectin on proteins, which reduced PPIs binding to ligands. The results were consistent with some of the solution result.
Figure 3. Surface hydrophobicity of PPI and PPI-polysaccharide complexes. Data represent the mean ± one standard deviation (n = 3). The data were subjected to ANOVA test and differences between means were evaluated by Duncan’s multiple range test. Different superscript letters indicate significant differences (P<0.05).
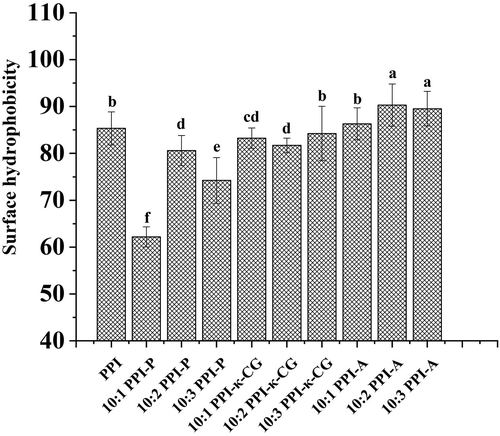
Thermal properties
The thermodynamic transition temperature represented the thermal stability of the protein. The thermal denaturation temperatures of myosin were mostly between 40°C and 60°C, the denaturation temperatures of collagen were between 60°C and 70°C, and actin had better thermal stability with denaturation temperatures of 70–80°C.[Citation33] shows the DSC thermograms of PPIs and PPI-polysaccharide complexes. For PPIs, the heat flow curve showed only one obvious peak at 60.4°C, which indicated that the peak may indicate the denaturation of mainly myosin in PPI.
Figure 4. DSC thermograms of PPI, PPI-P (a), PPI-κ-CG (b) and PPI-A (c) complexes with different mixing ratios.
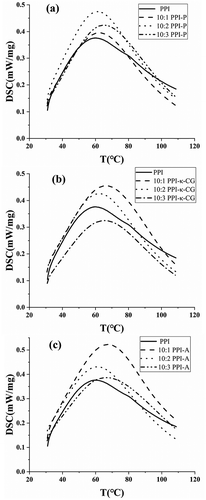
For PPI-polysaccharide complexes, protein and polysaccharides will interact with each other, which causes the functional properties of the protein to vary. From results as a whole (), the change in denaturation temperature for the mixed systems showed a similar pattern (decreased first and then increased) with an increasing number of polysaccharides. Among the PPI-P complexes, the maximums of onset temperature (T0) and denaturation temperature (Tp) were observed in 10:1 PPI-P (43.9°C) and 10:3 PPI-P (65.5°C). Among the PPI-κ-CG complexes, the maximums of T0 and Tp were observed in 10:1 PPI-κ-CG (45.0°C) and 10:3 PPI-κ-CG (66.7°C). Among the PPI-A complexes, the maximums of T0 and Tp were observed in 10:3 PPI-A (43.9°C) and 10:1 PPI-A (67.5°C). The results showed that the addition of polysaccharides could generally improve the thermal denaturation temperature of PPIs. Wagoner & Foegeding[Citation34] investigated the formation and thermal stability of whey protein isolate and high-methoxyl pectin, observed an increase in the thermal stability of complexes.
Table 2. Thermal transition properties of PPI and PPI-polysaccharide complexes.
Rheological properties
The rheological properties of proteins are useful in the food industry, such as in cheeses, yogurts, jellies, and meat sauces, to control mouth feel and texture. Different hydrophilic polysaccharides had different rheological properties, which have different effects on the dynamic gelation process of PPI. The behavior of PPIs and PPI-polysaccharide complexes was evaluated by monitoring the storage modulus (G′), as shown in , which indicated elastic gel-like properties. During the heating process, the change trend of the storage modulus of the system can be roughly divided into three stages. Between 0 and 25 min, the temperature rose from 0°C to 70°C, and the G′ value of the system had no significant change. Between 25 and 42.5 min, the G′ value of PPI increased rapidly from 75°C, continued to rise at a temperature maximum of 80°C, and reached a maximum when the system temperature dropped to 70°C. This result suggested that the network of PPI-PPI was formed within a high-temperature range of 70–80°C. At this stage, higher temperatures facilitated PPI denaturation and protein group association, which resulted in the formation of a three-dimensional polymer network structure. Subsequently, the G′ value of PPI decreased quickly after 42.5 min and eventually stabilized at approximately 3000 Pa. During this phase, proteins rapidly denatured, and most of the network structures were destroyed. This change was mainly related to the breakage of myosin chains.
Figure 5. Dynamic storage (G') of PPI, PPI-P (a), PPI-κ-CG (b) and PPI-A (c) complexes with different mixing ratios.
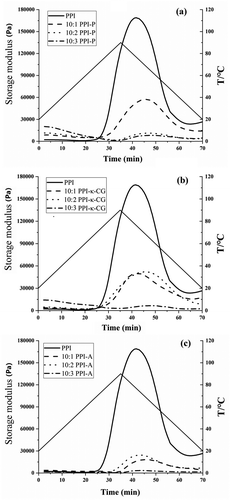
For PPI-polysaccharide complexes, the change trend of the G′ value showed a similar pattern for all PPI-polysaccharide systems (PPI-P, PPI-κ-CG, and PPI-A). Between 0 and 25 min, the conjugation of pectin, κ-CG, and PPI slightly improved the G′ value of PPI with increasing temperature, which indicated that pectin and κ-CG contributed to the formation of the PPI-PPI network at low temperatures. Between 25 and 70 min, the conjugation of PPI and hydrophilic polysaccharides reduced the G′ value of PPI, which indicated that the formation of the PPI-PPI network was suppressed. Simultaneously, the G′ value of PPI decreased with increasing polysaccharide mixing ratio at this stage. For PPI-P and PPI-κ-CG systems, compared to PPI, the G′ value of the 10:3 system experienced the largest reduction, followed by those of the 10:2 system and the 10:1 system. For the PPI-A system, the G′ value of the 10:3 system experienced the largest reduction, followed by those of the 10:1 system and the 10:2 system. The moisture content is one of the important factors which influence rheological properties of complexes. During the course of the experiment, it was observed that the conjugation of PPI and hydrophilic enhanced water holding capacity of systems, the fluidity increase might lead to the degradation of gelation ability.[Citation35] In addition, the formation of gel network was affected by polysaccharides in complexes. It was proved that simple polysaccharides could form a gel structure, and the gel capability of complexes with the addition of polysaccharides may be improved.[Citation36] In this work, the gel capability of complexes were not actually promoted. Some papers found that the final pH value of 7.0 might be not the optimum condition for the gel property of complexes.[Citation37,Citation38] According to the phenomenon, the effect of pH on the gel properties of complexes would be further studied in the future work.
FT-IR spectroscopy
Fourier transform infrared (FT-IR) spectroscopy is a powerful tool to investigate changes in the molecular structures of PPIs with added polysaccharides (-). The FTIR analysis of PPIs and PPI-polysaccharide complexes was based on the identification of bands related to the functional groups present in polysaccharides and pearl protein.[Citation39] Overall, the spectrum of PPIs and complexes were not much difference. It was indicated that the conjugation of PPI and polysaccharides produced no new functional groups and only electrostatic interaction existed between PPI and polysaccharides. shows typical peaks in different wavelength ranges representing the absorption of different functional groups. In the FT-IR spectra, the wavelength range of 800–1200 cm−1 (PK1) represents C-C and C-O stretching as well as C-H bending of colloidal polysaccharides.[Citation40,Citation41] PK2 was located at 1600–1700 cm−1 (representing C = O stretching/hydrogen bonding coupled with COO in the amide I region of proteins), PK3 was located at 2800–3000 cm−1 (representing C-H stretching of fatty acids), and PK4 was located at 3200–3400 cm−1 (representing O-H stretching of water absorption).[Citation18,Citation42] As shown in and , comparing with PPI, obvious shift of PK1 and PK4 was observed. Displacement of PK1 at the most of PPI-P and PPI-κ-CG complexes moved to lower wave-numbers (1085.0–1055.0 cm−1), displacement of PK4 at 10:2 and 10:3 PPI-P complexes moved to lower wave-numbers (3292.4–3217.9 cm−1) while PK2 and PK3 had no significant change (FT-IR resolution was about 4 cm−1). These phenomena imply a significant improvement in the force of hydrogen bonding in PPI due to the added polysaccharides.
Table 3. Main absorption peak positions of FT-IR spectroscopy of PPI and PPI-polysaccharide complexes.
Conclusion
The results of this study suggested that the conjugation of PPI and hydrophilic polysaccharides (pectin, kappa-carrageenan, and alginate) could improve some functional properties in comparison to PPI. Among five properties, emulsifying properties and thermal properties of PPI were obviously improved while other properties were improved in different degrees. PPI-polysaccharide complexes changed the solubility of PPI to different degrees, and the 10:3 PPI-P complex had the highest solubility. The emulsion properties of the 10:2 and 10:3 PPI-alginate complexes were significantly higher than other systems. The conjugation of PPI and pectin could reduce surface hydrophobicity of PPI in a certain degree. The thermal denaturation temperatures of complexes were generally higher than that of PPI, which is an important advantage, suggesting that complexes would be more stable during the heat process, preventing heat denaturation of the protein. FT-IR spectroscopy showed O-H stretching of water absorption and C-C and C-O stretching and C-H bending of colloidal polysaccharides were improved in PPI-polysaccharide complexes. The results presented here support the potential use of PPI-polysaccharide complexes in the food industry and broad application prospects of aquatic proteins in view of the improvement of some functional properties.
Conflict of interest
The authors have declared no conflict of interest.
Additional information
Funding
References
- Zheng, H.; Zhang, C.; Cao, W.; Liu, S.; Ji, H. Preparation and Characterisation of the Pearl Oyster (Pinctada Martensii) Meat Protein Hydrolysates with a High Fischer Ratio. Int. J. Food Sci. Technol. 2009, 44(6), 1183–1191. DOI: 10.1111/j.1365-2621.2009.01942.x.
- Zheng, H.; Zhang, C.; Qin, X.; Gao, J.; Li, T. Study on the Protein Fractions Extracted from the Muscle Tissue of Pinctada Martensii and Their Hydrolysis by Pancreatin. Int. J. Food Sci. Technol. 2012, 47(10), 2228–2234. DOI: 10.1111/j.1365-2621.2012.03093.x.
- Zhang, C.; Wu, H.; Hong, P.; Deng, S.; Lei, X. Nutrients and Composition of Free Amino Acid in Edible Part of Pinctada Martensii. J Fisheries of China (In Chinese). 2000, 24(2), 180–184.
- Wang, J.; Qian, W.; He, Y.; Xiong, Y.; Song, P.; Wang, R.-M. Reutilization of Discarded Biomass for Preparing Functional Polymer Materials. Waste Manage. 2017, 65, 11–21. DOI: 10.1016/j.wasman.2017.04.025.
- You, J.; Luo, Y.; Shen, H. Functional Properties of Water-Soluble Proteins from Silver Carp (Hypophthalmichthys Molitrix) at Different pHs. J. Aquat. Food Prod. T. 2013, 22(5), 487–495. DOI: 10.1080/10498850.2012.668159.
- Cha, Y.; Wu, F.; Zou, H.; Shi, X.; Zhao, Y.; Bao, J.; Du, M.; Yu, C. High-Pressure Homogenization Pre-Treatment Improved Functional Properties of Oyster Protein Isolate Hydrolysates. Molecules. 2018, 23(12), 3344. DOI: 10.3390/molecules23123344.
- Evans, M.; Ratcliffe, I.; Williams, P. A. Emulsion Stabilisation Using Polysaccharide-protein Complexes. Curr. Opin. Colloid Int. 2013, 18(4), 272–282. DOI: 10.1016/j.cocis.2013.04.004.
- Akhtar, M.; Ding, R. Covalently Cross-linked Proteins & Polysaccharides: Formation, Characterisation and Potential Applications. Curr. Opin. Colloid Int. 2017, 28, 31–36. DOI: 10.1016/j.cocis.2017.01.002.
- Warnakulasuriya, S. N.; Nickerson, M. T. Review on Plant Protein–polysaccharide Complex Coacervation, and the Functionality and Applicability of Formed Complexes. J. Sci. Food Agr. 2018, 98(15), 5559–5571. DOI: 10.1002/jsfa.9228.
- O’Sullivan, J. J.; Kurukji, D.; Norton, I. T.; Spyropoulos, F. Investigation of the Fabrication and Subsequent Emulsifying Capacity of Potato Protein isolate/κ-carrageenan Electrostatic Complexes. Food Hydrocolloid. 2017, 71, 282–289. DOI: 10.1016/j.foodhyd.2016.11.031.
- Almeida, N.; Rakesh, L.; Zhao, J. The Effect of Kappa Carrageenan and Salt on Thermoreversible Gelation of Methylcellulose. Polym. Bull. 2018, 75(9), 4227–4243. DOI: 10.1007/s00289-017-2256-z.
- Oduse, K.; Campbell, L.; Lonchamp, J.; Euston, S. R. Electrostatic Complexes of Whey Protein and Pectin as Foaming and Emulsifying Agents. Int. J. Food Prop. 2017, 20(sup3), S3027–S3041. DOI: 10.1080/10942912.2017.1396478.
- Sun, J.; Yin, G.; Chen, J.; Li, P. Gelling Properties of Myofibrillar Protein–soy Protein and K-carrageenan Composite as Affected by Various Salt Levels. Int. J. Food Prop. 2019, 22(1), 2047–2056. DOI: 10.1080/10942912.2019.1705482.
- Vijayalakshmi, K.; Devi, B. M.; Latha, S.; Gomathi, T.; Sudha, P. N.; Venkatesan, J.; Anil, S. Batch Adsorption and Desorption Studies on the Removal of Lead (II) from Aqueous Solution Using Nanochitosan/sodium Alginate/microcrystalline Cellulose Beads. Int. J. Biol. Macromol. 2017, 104, 1483–1494. DOI: 10.1016/j.ijbiomac.2017.04.120.
- Ding, W.; Zhou, J.; Zeng, Y.; Wang, Y. N.; Shi, B. Preparation of Oxidized Sodium Alginate with Different Molecular Weights and Its Application for Crosslinking Collagen Fiber. Carbohyd. Polym. 2017, 157, 1650–1656. DOI: 10.1016/j.carbpol.2016.11.045.
- Sha, X. M.; Tu, Z. C.; Liu, W.; Wang, H.; Shi, Y.; Huang, T.; Man, Z. Z. Effect of Ammonium Sulfate Fractional Precipitation on Gel Strength and Characteristics of Gelatin from Bighead Carp (Hypophthalmichthys Nobilis) Scale. Food Hydrocolloid. 2014, 36, 173–180. DOI: 10.1016/j.foodhyd.2013.09.024.
- Wei, Z.; Gao, Y. Physicochemical Properties of β-carotene Bilayer Emulsions Coated by Milk Proteins and chitosan–EGCG Conjugates. Food Hydrocolloid. 2016, 52, 590–599. DOI: 10.1016/j.foodhyd.2015.08.002.
- Zheng, H.; Beamer, S. K.; Matak, K. E.; Jaczynski, J. Effect of κ-carrageenan on Gelation and Gel Characteristics of Antarctic Krill (Euphausia Superba) Protein Isolated with Isoelectric Solubilization/precipitation. Food Chem. 2019, 278, 644–652. DOI: 10.1016/j.foodchem.2018.11.080.
- Stone, A. K.; Cheung, L.; Chang, C.; Nickerson, M. T. Formation and Functionality of Soluble and Insoluble Electrostatic Complexes within Mixtures of Canola Protein Isolate and (κ-, ι- and λ-type) Carrageenan. Food Res. Int. 2013, 54(1), 195–202. DOI: 10.1016/j.foodres.2013.06.009.
- Klomklao, S.; Benjakul, S. Protein Hydrolysates Prepared from the Viscera of Skipjack Tuna (Katsuwonus Pelmamis): Antioxidative Activity and Functional Properties. Turk. J. Fish. Aquat. Sci. 2018, 18(1), 69–79. DOI: 10.4194/1303-2712-v18_1_08.
- Timilsena, Y. P.; Adhikari, R.; Barrow, C. J.; Adhikari, B. Physicochemical and Functional Properties of Protein Isolate Produced from Australian Chia Seeds. Food Chem. 2016, 212, 648–656. DOI: 10.1016/j.foodchem.2016.06.017.
- Damin, M. R.; Minowa, E.; Alcantara, M. R.; Oliveira, M. Chemical and Viability Changes during Fermentation and Cold Storage of Fermented Milk Manufactured Using Yogurt and Probiotic Bacteria. In XIIIth World Congress of Food Science and Technology “FOOD IS LIFE”, Nantes, France, September, 2006 17-21.
- Adapa, S.; Dingeldein, H.; Schmidt, K. A.; Herald, T. J. Rheological Properties of Ice Cream Mixes and Frozen Ice Creams Containing Fat and Fat Replacers. J. Dairy Sci. 2000, 83(10), 0–2229. DOI: 10.3168/jds.S0022-0302(00)75106-X.
- Zhang, B.; Cui, Y.; Yin, G.; Li, X.; Zhou, X. Alkaline Extraction Method of Cottonseed Protein Isolate. Mod. Appl. Sci. 2009, 3(3), 77–82. DOI: 10.5539/mas.v3n3p77.
- Klassen, D. R.; Elmer, C. M.; Nickerson, M. T. Associative Phase Separation Involving Canola Protein Isolate with Both Sulphated and Carboxylated Polysaccharides. Food Chem. 2011, 126(3), 1094–1101. DOI: 10.1016/j.foodchem.2010.11.138.
- Schmitt, C.; Sanchez, C.; Desobry-Banon, S.; Hardy, J. Structure and Technofunctional Properties of Protein-polysaccharide Complexes: A Review. Crit. Rev. Food Sci. 1998, 38(8), 689–753. DOI: 10.1080/10408699891274354.
- Mu, L.; Zhao, M.; Yang, B.; Zhao, H.; Cui, C.; Zhao, Q. Effect of Ultrasonic Treatment on the Graft Reaction between Soy Protein Isolate and Gum Acacia and on the Physicochemical Properties of Conjugates. J. Agr. Food Chem. 2010, 58(7), 4494–4499. DOI: 10.1021/jf904109d.
- Du, Y.; Shi, S.; Jiang, Y.; Xiong, H.; Woo, M. W.; Zhao, Q.; Sun, W. Physicochemical Properties and Emulsion Stabilization of Rice Dreg Glutelin Conjugated with κ-carrageenan through Maillard Reaction. J. Sci. Food Agr. 2013, 93(1), 125–133. DOI: 10.1002/jsfa.5739.
- Zhao, Y.; Li, F.; Carvajal, M. T.; Harris, M. T. Interactions between Bovine Serum Albumin and Alginate: An Evaluation of Alginate as Protein Carrier. J. Colloid. Interf. Sci. 2009, 332(2), 345–353. DOI: 10.1016/j.jcis.2008.12.048.
- Perez, A. A.; Carrara, C. R.; Sánchez, C. C.; Patino, J. M. R.; Santiago, L. G. Interactions between Milk Whey Protein and Polysaccharide in Solution. Food Chem. 2009, 116(1), 104–113. DOI: 10.1016/j.foodchem.2009.02.017.
- Mena‐Casanova, E.; Totosaus, A. Improvement of Emulsifying Properties of Milk Proteins with κ or λ Carrageenan: Effect of pH and Ionic Strength. Int. J. Food Sci. Tech. 2011, 46(3), 535–541. DOI: 10.1111/j.1365-2621.2010.02536.x.
- Li, T.; Wang, C.; Li, T.; Ma, L.; Sun, D.; Hou, J.; Jiang, Z. Surface Hydrophobicity and Functional Properties of Citric Acid Cross-linked Whey Protein Isolate: The Impact of pH and Concentration of Citric Acid. Molecules. 2018, 23(9), 2383. DOI: 10.3390/molecules23092383.
- Brunton, N. P.; Lyng, J. G.; Zhang, L.; Jacquier, J. C. The Use of Dielectric Properties and Other Physical Analyses for Assessing Protein Denaturation in Beef Biceps Femoris Muscle during Cooking from 5 to 85 C. Meat Sci. 2006, 72(2), 236–244. DOI: 10.1016/j.meatsci.2005.07.007.
- Wagoner, T. B.; Foegeding, E. A. Whey Protein–pectin Soluble Complexes for Beverage Applications. Food Hydrocolloid. 2017, 63, 130–138. DOI: 10.1016/j.foodhyd.2016.08.027.
- Li, X.; Xia, W. Effects of Chitosan on the Gel Properties of Salt-soluble Meat Proteins from Silver Carp. Carbohyd. Polym. 2010, 82(3), 958–964. DOI: 10.1016/j.carbpol.2010.06.026.
- Moreira, H. R.; Munarin, F.; Gentilini, R.; Visai, L.; Granja, P. L.; Tanzi, M. C.; Petrini, P. Injectable Pectin Hydrogels Produced by Internal Gelation: PH Dependence of Gelling and Rheological Properties. Carbohyd. Polym. 2014, 103, 339–347. DOI: 10.1016/j.carbpol.2013.12.057.
- Çakır, E.; Khan, S. A.; Foegeding, E. A. The Effect of pH on Gel Structures Produced Using Protein–polysaccharide Phase Separation and Network Inversion. Int. Dairy J. 2012, 27(1–2), 99–102. DOI: 10.1016/j.idairyj.2012.03.013.
- Ching, S. H.; Bansal, N.; Bhandari, B. Alginate Gel particles–A Review of Production Techniques and Physical Properties. Crit. Rev. Food Sci. 2017, 57(6), 1133–1152. DOI: 10.1080/10408398.2014.965773.
- Beekes, M.; Lasch, P.; Naumann, D. Analytical Applications of Fourier Transform-infrared (FT-IR) Spectroscopy in Microbiology and Prion Research. Vet. Microbiol. 2007, 123(4), 305–319. DOI: 10.1016/j.vetmic.2007.04.010.
- Pawlak, A.; Mucha, M. Thermogravimetric and FTIR Studies of Chitosan Blends. Thermochim. Acta. 2003, 396(1–2), 153–166. DOI: 10.1016/S0040-6031(02)00523-3.
- Souza, C. J.; Garcia-Rojas, E. E. Effects of Salt and Protein Concentrations on the Association and Dissociation of Ovalbumin-pectin Complexes. Food Hydrocolloid. 2015, 47, 124–129. DOI: 10.1016/j.foodhyd.2015.01.010.
- Kobayashi, Y.; Mayer, S. G.; Park, J. W. FT-IR and Raman Spectroscopies Determine Structural Changes of Tilapia Fish Protein Isolate and Surimi under Different Comminution Conditions. Food Chem. 2017, 226, 156–164. DOI: 10.1016/j.foodchem.2017.01.068.