ABSTRACT
The swiftlet’s nest is an edible bird’s nest (EBN) produced from dried gelatinized saliva secreted by swiftlets during the breeding season. It is widely used in industries for its high nutritional values and health benefits. However, the utilization of ESN is restricted due to its physicochemical properties, such as insolubility. The recently invented bioactive ESN hydrolyzate is reported to improve the ESN’s solubility, functional and nutritional properties to broaden its application. This study has produced bioactive ESN hydrolyzates with different hydrolysis periods to investigate the optimum conditions to achieve maximized degree of hydrolysis (DH) and to compare the physicochemical differences. The result revealed that optimum ESN hydrolysis was at 90 minutes, in which the DH and enhanced solubility (three folds) indicated a complete breakdown of glycoprotein into bioactive glycopeptide. This result is in line with the physicochemical analyses result. The hydrolysis has significantly decreased (p ≤ .05) the ESN protein content, while the levels for peptide, glycopeptide, polysaccharides, and sialic acid showed the opposite. Interestingly, the amino acid from raw ESN and hydrolyzates in all incubation periods showed no significant difference (p ≤ .05). This result suggests that the ESN biological properties remained unchanged with alcalase hydrolysis. Therefore, the findings in this study confirm the feasibility of bioactive ESN hydrolyzate as a versatile product suitable for various industries in utilizing ESN in whole instead of as extracts.
Introduction
The edible swiftlet’s nest (ESN) or bird’s nest (EBN) is a highly valued traditional Chinese herbal material (CHM).[Citation1] It is a dried gelatinized salivary secretion produced by swiftlets during the breeding season.[Citation2] The nests are usually harvested from wild caves or dark swiftlet farm buildings with high moisture. The nests go through a cleaning process to remove feathers, dirt, dust and other inedible materials. The cleaning process usually involves soaking with water to make the ESN swell to ease the removal of foreign materials using tweezers.
There are two primary sources of consumable ESN: the white ESN are produced from Aerodramus fuciphagus, while the black ESN are from Aerodramus maximus. Traditionally, ESN is consumed by double-boiling with rock sugar and other herbal ingredients, such as ginseng, red dates, karaya gum, red seaweed, and Tremella fungus. Nowadays, many readily served ESN products in bottles and cans are available in the market. The ESN market price has been high and stable due to its limited supply and high demands worldwide. Hence, ESN is labeled as one of the most expensive animal-based protein product. This exclusiveness has even led to authenticity issues of ESN and the presence of fake swiftlet’s nests in the market.[Citation1]
Currently, the leading suppliers of swiftlet’s nest are Indonesia, Thailand, and Malaysia. The main market focuses on the Chinese community. The swiftlet’s nest has been widely used since the olden days, particularly in Traditional Chinese Medicine (TCM) dated as far as the Tang and Ming Dynasties of China.[Citation3] In the Chinese community, ESN is called “yanwo,” which means swiftlet bird’s nest.[Citation4,Citation5] Edible swiftlet’s nest has a notable reputation and value as the “Caviar of the East” due to the belief in its medicinal and nutritional properties as a CHM in TCM. It is used in treating health disorders and consumptive diseases, such as autoimmune diseases, coughs, tuberculosis, asthma, stomach ulcer, and gastrointestinal disorders. The ESN also promotes physical and mental strength, and anti-aging to maintain youthfulness.[Citation4,Citation6,Citation7] Today, these beliefs have been proven by science for its role as a nutraceutical food. Scientific evidence supports the claims of ESN in TCM. For example, there are evidence on the ESN’s immunity enhancing properties[Citation8]; epidermal growth factor (EGF) that promotes anti-aging effects[Citation9]; ganglioside and brain structuring by sialic acid[Citation10]; anti-influenza prevention properties[Citation2]; anti-hypertensive properties[Citation11]; and prebiotic ingredients that improve gut health.[Citation12,Citation13]
In the modern times, ESN has been used as food supplement, nourishment, medicine, and even cosmetic component.[Citation3,Citation14,Citation15] To ensure the highly valued ESN is fully utilized, many studies have been conducted to broaden its application in a broader range of industries. However, the applications of ESN are restricted due to its physicochemical properties such as low solubility and extraction rate.[Citation16] For instance, the swiftlet’s nest would swell like a sponge without any further changes even after many hours or days of heating and soaking.[Citation17] Thus, the applications of ESN in industries such as the pharmaceutical and cosmetic industries are restricted to only utilizing the ESN extract as a functional ingredient. This deficiency limits ESN from achieving its full potential for functionality and health benefits.
In recent years, downstream processing and research on ESN development have produced a patented product known as the bioactive ESN hydrolyzate.[Citation18,Citation19] This eco-friendly biotechnology has been applied on many plants and animal proteins to produce bioactive peptide (BAP) from milk, casein, whey, egg, meat, fish (e.g. atlantic cod and atlantic salmon), soy beans, sesame bran and oil by-products, peas, and even food waste like fish skin.[Citation20–28] There are also glycopeptide derived from commercial bovine whey protein,[Citation29] Ganoderma lucidum,[Citation30] and horseradish peroxidase.[Citation31] This protein enhancement as bioactive hydrolyzate allows industries to make the most of underutilized protein, protein by-products, and even specifically utilized protein with specific peptide sequences or amino acid residues.[Citation22] The ESN micro-particles invented by Babji in 2014 hydrolyzes the ESN SiaMuc-glycoprotein into simpler and soluble bioactive SiaMuc-glycopeptide and free peptides.[Citation18] In which, the enzyme converts insoluble protein into a soluble form that promotes a high rate of hydrolysis. In the second step, the soluble protein is hydrolyzed from a complex macro-protein form into simpler bioactive peptide. This technology is reported to enhance ESN in terms of nutrition, functionality, and solubility. As a result, more than 90% of ESN recovery is achieved. Also, the bioactivity of ESN such as the anti-hypertensive,[Citation32] anti-diabetic,[Citation33] and prebiotic acitivity[Citation13] are enhanced due to the application of enzymatic hydrolysis. In short, this enhancement improves the ESN application feasibility and allows industries to wholly utilize ESN in various products. As a versatile ingredient, a small quantity of ESN is sufficient to achieve maximum functionality and benefits.
Studies conducted on bioactive ESN hydrolyzate discovered evidence of improvement as an anti-hypertension,[Citation11] anti-oxidative,[Citation34] and prebiotic potential for gastrointestinal gut health.[Citation13] However, the effect of enzymatic hydrolysis on the physicochemical properties and nutritional bioavailability of ESN is still limited. Currently, there is only few studies regarding to hydrolysis of ESN SiaMuc-glycoprotein. That includes study of Amiza et al.[Citation35] on the optimization of the hydrolysis of ESN in terms of degree of hydrolysis and; study of Khushairay et al.[Citation36] on hydrolysis of ESN in relation to the peptide content. There is no study reported on the hydrolysis of ESN by means of degree of hydrolysis, nutritional bioavailability, and physicochemical properties. In addition, the optimization is yet to be reported, particularly on the optimized incubation period to produce ESN hydrolyzate with maximized nutritional bioavailability. Therefore, this study highlighted the production of ESN hydrolyzates with different hydrolysis periods using enzymatic biotechnology on raw ESN in order to investigate the degree of hydrolysis (DH) that determined the maximized hydrolysis period by alcalase with maximized nutritional bioavailability and fucntionality. Furthermore, a comparison of nutritional bioavailability and physicochemical properties, including solubility, protein, peptide, glycoprotein/peptide, polysaccharide, reducing sugar, sialic acid, and amino acid profile of raw ESN and all hydrolyzates were also ascertained. This information may be crucial and valuable for various industries as different ESN applications require specific nutrient, criteria, and conditions.
Materials and methods
Materials
House farmed ESN samples from the swiftlet species Aerodramus fuciphagus were obtained from Mobile Harvester Malaysia Sdn. Bhd. in Shah Alam, Selangor, Malaysia. These samples were supplied in clean conditions as they had been subjected to a cleaning process in accordance with the company’s standard operating procedure to remove foreign materials such as feathers and other inedible impurities. The samples were crushed and ground using a homogenizer and then stored in an air-tight container at room temperature for subsequent analysis. The samples were also hydrolyzed using protease from Bacillus licheniformis to produce ESN hydrolyzate as study objects. Samples investigated includes a double-boiled raw ESN (i.e. ER) and four different ESN hydrolyzates produced from the hydrolysis of 1, 2, 3, and 4 hours respectively (i.e. EH1, EH2, EH3, EH4). The chemicals used in this study were purchased from Sigma-Aldrich, UK.
Proximate nutritional analysis
A proximate analysis of crude protein content, fat content, ash content, moisture content and carbohydrate content was carried out in accordance with the Association of Official Analytical Chemistry methods.[Citation37] The proximate study was conducted before the ESN hydrolyzate production, as the hydrolysis process was protein-based content. The Kjeldahl method was used to determine the total crude protein content in ESN, using 6.25 as a conversion factor.[Citation38,Citation39] The Soxhlet method was used to determine fat content in the sample using hexane (C6H14).[Citation40,Citation41] The ash content was determined using “dry ashing” method at 450–550°C overnight.[Citation42] The ESN moisture content was determined through oven drying at 105°C for at least 16 hours until no changes of weight was detected. The carbohydrate content was obtained using the subtraction method, whereby 100% was subtracted from the total protein content, fat content, moisture content, fiber content, and ash content. The fiber content analysis was excluded as studies reported that the fiber content in ESN was insignificant with less than 0.01%.[Citation43–45]
Sample Preparation: Double-boiling, Enzymatic Hydrolysis, and Processing of Bioactive ESN Hydrolyzate
The ESN hydrolysis was carried out based on the Babji[Citation18] method (Patent No. WO2017034390A2). The ESN hydrolyzate production consists of the following stages: (i) Swelling process by overnight soaking, (ii) Double boiling process to facilitate the hydrolysis process, (iii) Enzymatic hydrolysis, (iv) Deactivation of hydrolysis, and (v) Freeze drying
Pre-treatment of raw ESN
The ESN double-boiling process was done according to the Garcia-Segura et al.[Citation46] study. Raw ESN was soaked in distilled water at a ratio of 1:100 and kept overnight in a chiller at 4°C for 16 hours. Next, the soaked material was double-boiled in a water bath at 90 ± 2°C for 30 minutes. After the double-boiling process, the sample was let to cool to room temperature for enzymatic hydrolysis.
Hydrolysis of ESN
The ESN hydrolysis was carried out based on Babji’s[Citation47] patented enzymatic process (Patent No. WO2017034390A2). The soaked ESN was placed into a pre-heated shaking water bath 15 minutes before enzyme incubation. Then, the ESN suspension was incubated with 1% alcalase enzyme at a ratio of 1:100 w/v from the total protein content. During this process, 0.1 N of NaOH was constantly added at every 15 minutes to maintain optimum pH level. The enzymatic incubation was carried out for 60, 120, 180, and 240 minutes to produce ESN hydrolyzate by four different incubation periods. After that, the hydrolysis process was deactivated with boiling water for 10 minutes. The hydrolyzate was then frozen overnight at −80°C and subsequently freeze-dried.
Degree of hydrolysis
The degree of hydrolysis (DH) was determined by the pH stat method from the Adler-Nissen[Citation48] experiment. The optimum period of ESN hydrolysis was determined when the ESN’s maximum DH was achieved. The sample’s enzymatic hydrolysis was carried out in a thermostatically controlled stirring batch reactor (Auto-titrator Horiba Scientific SZ-100). The sample’s temperature and pH were adjusted according to the enzyme requirement with 0.1 N NaOH. The hydrolysis process started when the 1% enzyme was added and the release of hydrogen in the hydrolysis process decreased the sample’s pH. The 0.1 N of NaOH was auto-titrated continuously to maintain the enzyme’s required conditions. The 0.1 N NaOH volume used during this process was recorded, and the process proceeded for 1, 2, 3, and 4 hours until a constant 0.1 N NaOH volume was reached. The DH formula was defined as the percentage ratio of the number of peptide bonds (h) broken to the total number of peptide bonds (htot) in the substrate.
where B is the amount of NaOH consumed (mL) during the hydrolysis; Nb is the normality of NaOH; MP is the mass (g) of the protein; α is the average degree of dissociation of the α-NH2 groups released during the hydrolysis process.
where pK is the average dissociation value for α-amino groups liberated during hydrolysis and dependent on temperature, peptide chain length, and the nature of the terminal amino acid. The total number of peptide bonds (htot) in whey protein was assumed to be 8.8 meq g−1.
Physicochemical analysis
Total Solid Solubility (TSS): The total solid solubility (TSS) test was performed by Brix refractometry method.[Citation49] An ESN sample solution of 2.5%, 5% and 10% (w/v) was prepared by mixing it with distilled water. A drop of the solution was placed onto the prism surface of a Brix refractometer (Mettler Toledo 0–39%) for 30 seconds. The °Brix reading was observed under bright light at room temperature and recorded as a concentration of soluble solid (%).
Protein Content Quantification; The Bradford method was used to measure the soluble protein content in the sample.[Citation50] Sample was prepared at a concentration of 1.0 mg/mL. Next, a total of 250 μL of Bradford reagent was mixed with 5.0 μL of sample and left for 2 min. The sample mixture’s absorbance was measured using an EpochTM Microplate Spectrophotometer (Bio Tek, US) under 595 nm wavelength. Bovine serum albumin (BSA) was used as a standard. The peptide content were measured using an o-phthaldialdehyde (OPA) spectrophotometric assay with a modified method.[Citation11] A fresh OPA reagent (50 mL) was prepared by mixing 25 mL of 100 mM sodium tetra-hydroborate, 2.5 mL of 20% (w/w) sodium dodecyl sulfate (SDS), 40 mg of OPA solution dissolved in 1 mL of methanol, and 100 μL of b-mercaptoethanol, and then topped up with deionized water to 50 mL. The reagent was kept in the dark to prevent degradation by light. Next, a mixture of 50 µL sample (containing 5–100 g protein) and 300 µL of OPA reagent was incubated for 2 minutes at room temperature. The sample’s absorbance was then analyzed under a spectrophotometer (Bio Tek, US) at 340 nm wavelength using L-Leucine as a standard to quantify the peptide content.
Amino acid content
The amino acid profile of ESN and hydrolyzate samples was analyzed using High-Performance Liquid Chromatography (HPLC) with three different hydrolysis stages prior to analysis.
Hydrochloric acid (HCl) hydrolysis
This analysis was performed to quantify the concentration of aspartic acid, threonine, serine, glutamic acid, proline, glycine, alanine, valine, isoleucine, leucine, tyrosine, phenylalanine, histidine, lysine, and arginine. Sample (0.2 g) was hydrolyzed with 5 mL of 6 N HCl in an enclosed tube at 110°C for 24 hours. After the sample had cooled down, 400 µL of 50 µmol α-aminobutyric acid (AABA) solution was added as an internal standard and then topped-up to 100 mL with deionized water.
Performic acid hydrolysis
The quantification of methionine and cysteine was done using the performic acid hydrolysis method. A sample of 0.1–0.2 g was mixed with 2 mL performic acid and stored in a chiller at 4°C for 16 hours. Next, 0.4 mL of chilled hydrogen bromide (HBr) was added, and the chilling process was continued for another 30 minutes. The sample was dried using water bath heating at 100°C, followed by HCl hydrolysis.
Alkaline hydrolysis
The tryptophan in the sample were quantified using alkaline hydrolysis. The sample (0.2 g) was mixed with 15 mL lithium hydroxide monohydrate (LiOH.H2O) and heated at 120°C for 16 hours before 9 mL 6.0 N HCl was added to the sample. The sample was adjusted to pH 4.5 using 0.1 N HCl, and then topped-up with deionized water to 100 mL in a volumetric flask.
Analysis by HPLC
All samples were filtered using a 0.22 µm syringe filter before analysis. For the sample from the hydrochloric acid and performic acid hydrolysis, the amino acids profile was analyzed using Seo’s[Citation51] AccQ Tag method with High-Performance Liquid Chromatography (HPLC) system (Waters 1525 Model, USA) with reverse phase of AccQ Tag Amino Acid Column (QAT052885, particle size of 4 μm, 3.9 x 150 mm). The analytes were analyzed with a fluorescence detector using 60% Acetonitrile as a mobile phase. The amount of sample injected was 10 μL at a flow rate of 1.0 mL/min with a heated column of 31–36°C isocratic elution for 12 minutes.
The tryptophan detection from the alkaline hydrolysis was conducted using a C18 Reverse phase column (Nova Pak, 5 µm, 25–30 cm) with a fluorescence detector. The system was incorporated with a mobile phase of 0.0085 M sodium acetate (pH 4.0) and methanol at a ratio of 21.7:3.3. The analysis was run at 1.0 mL/min in ambient condition with 10 µL sample injected.
Glycan content quantification
The phenol-sulfuric acid method was assayed to access the total polysaccharide content in sample.[Citation52] A quantity of 1.0 mL sample (1.0 mg/mL) was mixed with 0.50 mL of 5% phenol solution (w/w), and then added with 1.5 mL concentrated sulfuric acid (H2SO4). The mixture was mixed well and left to set for 10 minutes at room temperature and then transferred into a 96-well microplate for analysis. The absorbance was read at 490 nm using an EpochTM Microplate Spectrophotometer (Bio Tek, US). The standard used was 1.0 mg/mL of glucose monohydrate. The reducing sugar content was determined using the 3,5-dinitrosalicylic acid (DNS) method as described by Saqib and Whitney.[Citation53] The DNS reagent was prepared with 1.0 g of dinitrosalicylic acid (DNS) and 30 g of sodium potassium tartrate added into 40 mL of 2.0 N NaOH. Next, the reagent was warmed to mix well and topped-up to 100 mL with distilled water. A total volume of 1 mL sample (1.0 mg/mL) was mixed with 4 mL of DNS reagent in a test tube. The test tube sample was heated in a boiling water bath for 5 minutes, and then cooled in an ice water bath immediately. The mixture was then left to set at room temperature. The mixture’s absorbance was read using the EpochTM Microplate Spectrophotometer (Bio Tek, US) with a wavelength of 540 nm. Glucose monohydrate was used as a standard.
Total sialic acid content
The resorcinol method was employed to quantify the samples’ sialic acid content.[Citation54] A 100 mL resorcinol reagent was prepared by mixing 0.22 g of resorcinol in 10 mL of distilled water, 80 mL of concentrated hydrochloric acid (HCl), and 0.25 mL of 0.1 M copper sulfate (CuSO4) solution, and then topped up with distilled water. The reagent was kept in 5°C storage and became stable for analysis after 2 weeks. Sample of 500 µL was then mixed with 500 µL of resorcinol reagent in a test tube, and then covered with chilled marble for a 15-min incubation in 100°C water bath. An amount of 2.0 mL 1-butanol was then added into the solution, followed by vigorous vortex for at least 10 seconds. Next, the sample was chilled in a water bath for 10 minutes. The sample was transferred into a 96-well microplate and observed at 580 nm using EpochTM Microplate Spectrophotometer (Bio Tek, US). Sialic acid from Sigma was used as a standard.
Glycoprotein content
The quantification of glycoprotein content in the sample was determined by the Periodic Acid/Schiff (PAS) method.[Citation55] A sample of 5 to 100 μg glycoprotein content was prepared with distilled water. Sample (2.0 mL) underwent a periodate oxidation process with 0.2 mL periodic acid solution and then incubated for 2 hours at 37°C. The periodic acid solution was prepared by mixing 10 μL of 50% (w/vol) periodic acid with 10 mL of 7% acetic acid. After the incubation, 0.2 mL of Schiff fuchsin sulfite solution was added and then incubated for 30 minutes at room temperature. The sample’s absorbance was read using the EpochTM Microplate Spectrophotometer (Bio Tek, US) at 555 nm wavelength. Horseradish peroxidase was employed as the standard for the glycoprotein quantification.
Antioxidative activities: DPPH, FRAP, and ABTS analysis
DPPH Assay: The DPPH assay is carried out according to the study of Gan et al. [Citation56] DPPH reagent (0.1 mM) is prepared in methanol. Sample (100 µL) is incubated with 200 µL DPPH reagent for 30 minutes in dark, then read under a spectrophotometer (EpochTM, USA), at 517 nm. The free radical scavenging acitivity (%) is calculated using equation below.
where A1 is the absorbance of DPPH reagent and A2 is the absorbance of DPPH with sample.
Ferric reducing antioxidant power (FRAP) assay: The FRAP assay is conducted according to the study of Al-Laith et al.[Citation57] FRAP reagent is prepared by mixing 300 mM sodium acetate buffer, 10 mM of 2,4,6-tris (2-pyridyl)-s-triazine (TPTZ), and 20 mM Ferum Chloride hexahydrate at a ratio of 10:1:1. Sample (250 µL) is incubated with 1.25 mL of FRAP reagent in dark for 30 minutes, then read under spectrophotometer at 595 nm. Ascorbic acid is used as standard in this assay. The FRAP value for standard and sample are calculated as ascorbic acid equivalent (AAE) per mg of sample.
ABTS Assay: The ABTS assay is performed as described in Gan et al.[Citation56] ABTS reagent is prepared by mixing 7 mM ABTS and 2.45 mM of potassium persulfate. The solution is left in dark for 12–16 hours, then diluted with methanol until an absorbance of 0.70 ± 0.02 is achieved using a spectrophometer at 734 nm. Sample (50 µL) added with ABTS reagent (950 µL) then incubated in dark for 10 minutes before reading the absorbance. Ascorbic acid is used as standard in this assay.
Statistical analysis
All analyses were conducted in triplicates (n = 3). The one-way analysis of variance (ANOVA) was used to statistically analyze all means to determine whether there were any differences between the physicochemical properties of ESN and hydrolyzates at different hydrolysis period at 95% confidence level. The Tukey’s Multiple Range Test (p ≤ .05) was used to analyze means of treatment. The Statistical Analysis System (SAS) version 9.4 was employed as the statistical software for this study.
Result and discussion
Nutrient and composition of ESN
shows the proximate nutritional content of a cleaned raw ESN (ER). A number of studies have found that ESN contains 80–90% of unique glycoprotein as primary component. The glycoprotein made up the 60% protein and 20–30% carbohydrate in ESN.[Citation7,Citation43] The result in revealed similar results. Whereby, the major nutrient of ESN is protein at 60.93%, followed by carbohydrate at 20.98%. These were also reported in previous studies.[Citation7,Citation45,Citation58–60] The raw ESN’s protein, carbohydrate, moisture, fat, and ash content were determined as one of the crucial procedures for the hydrolysis process. In which, the hydrolysis of ESN was performed based on the protein content in ESN.
Table 1. Proximate analysis of total crude protein, fat, moisture, ash, and carbohydrate in raw ESN
Degree of hydrolysis and production of bioactive ESN hydrolyzate
The ESN underwent a double-boiling process to allow its structure to swell and open up to increase its bioaccessibility. The existing natural protein in food and diet is physiologically inactive within the parent protein molecule.[Citation61] Shylaja and Seshadri[Citation62] also reported a similar concept for glycoprotein, which exists in numerous forms with various functional properties in living organisms. The bioactive ESN hydrolyzate by Babji[Citation18] was developed by applying enzymatic technology to improve its micro-particulates in breaking down protein into its active form. Based on the past proximate results and , ESN has revealed a significantly low fat content.[Citation45,Citation59] This feature was desirable for the hydrolysis process which reduces the protein-lipid complex formation affecting the production of stable protein hydrolyzate.[Citation11,Citation63]
The enzymatic hydrolysis depends on the type of enzyme used that specifically cleave peptide bond in certain peptide chain and molecular weight and fractions.[Citation64,Citation65] Example of the commercially used enzyme in the food industry is likely to be alcalase and flavourzyme which can be obtained from plant, animal and microbes. They required specific conditions such as pH, temperature, concentration, and ratio for optimum hydrolysis process.[Citation66] According to See et al.,[Citation67] alcalase is an alkaline enzyme produced from Bacillus licheniformis in brown liquid form with a slight odor. The EBN protein exists in insoluble form even undergoing physical heat treatment during the double boiling process due to complicated core structure. In the process, the EBN protein involved two procedures with first step enzyme associated to the EBN insoluble macro protein, which produces soluble peptides and amino acids, followed by the second step of which the soluble and compact core protein is being hydrolyzed again.[Citation68] The alcalase applied in EBN break its protein at a nonspecific bond. According to Adler-Nissen,[Citation48] alcalase has the highest protein recovery in protein hydrolysis compare to papain and neutrase. At the same time, the taste of the food treated with alcalase have less bitterness compares to other enzymes. This benefits in further application of the target end product.[Citation69]
Alcalase is effective in optimum condition of 60°C and pH of 8.0, while the period of hydrolysis depends on the protein characteristics and quantity. Therefore, this study produced four different bioactive ESN hydrolyzates, each with different periods of hydrolysis process at 1, 2, 3, and 4 hours. The ESN enzymatic hydrolysis released bioactive SiaMuc-glycopeptides and free peptides from its core of SiaMuc-glycoprotein and protein origin.[Citation43,Citation70] According to Himonides et al.,[Citation71] degree of hydrolysis (DH%) of hydrolyzate is defined as the proportion of cleaved peptide bonds in a protein hydrolyzate and the key attribution for the protein hydrolyzate system. By evaluating the DH%, the hydrolytic degradation of ESN SiaMuc-glycoprotein at the different periods becomes measurable. In other words, a lower DH% meant a higher molecular weight fractions of hydrolyzates and vice versa.[Citation71] The pH-stat method used for evaluating DH% in this research detected pH changes during the hydrolysis process. The hydrolysis process broke down the SiaMuc-glycoprotein and released hydrogen ions which reduced the pH. In order to maintain the optimum pH throughout the hydrolysis process, a volume of sodium hydroxide (NaOH) was titrated for tracking trend of DH% for ESN.
displays an increasing ESN DH% that indicates an increasing cleaving of peptide bond and breakdown of ESN SiaMuc-glycoprotein into SiaMuc-glycopeptides during the incubation period (1–4 hours). The ESN DH% was statistically different for the first 1.5 hours (p ≤ .05). The hydrolysis rate increased vigorously for the first 1.5 hours, and then it entered a stationary phase at the next incubation period. In short, the ESN hydrolysis by alcalase had achieved its maximum rate at 1.5 hours (90 minutes).
Figure 1. Degree of hydrolysis (DH) for ESN hydrolyzed by alcalase for 1 hour (EH-1), 2 hours (EH-2), 3 hours (EH-3) and 4 hours (EH-4). a, b, c Superscript letters shows significant higher/lower DH comparing within the period (hour) of hydrolysis for the same sample at confidence level of 95% (n = 3). Note: EH-1, EH-2, EH-3 and EH-4 represent ESN hydrolyzates with specific hydrolysis period.
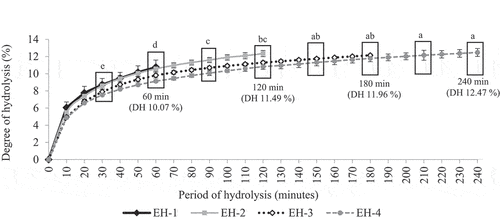
A previous study by Mackie[Citation72] on fish protein hydrolysis reported that the DH% rate initially decreased and eventually entered a stationary phase during incubation. Similar DH% findings were observed in the study of ESN. Protein, being the limiting factor, affects the availability and accessibility of peptide bond for hydrolysis and eventually inhibits the production of simpler peptides and amino acids.[Citation73] The mechanism of the enzyme reacting toward ESN protein also influences DH%. Based on Mohr’s[Citation68] study on fish protein, the insoluble protein requires two steps of hydrolysis. In the first step, the enzyme converts insoluble protein into a soluble form that promotes a high rate of hydrolysis. In the second step, the soluble protein is hydrolyzed from a complex macro-protein form into simpler bioactive peptide.
The same situation is believed to have occurred for the ESN SiaMuc-glycoprotein, whereby the ESN remained in an insoluble form even after long hours of double-boiling heat treatment. Hence, the ESN’s hydrolysis rate was high at the beginning and then gradually decreased. It was also observed that the ESN hydrolyzate has a lower DH% value (~4.8–6.0%) at the beginning of the hydrolysis process (i.e., EH-1 and EH-2) compared to the end (~10.0–12.0%). This is related to the abundance of substrate in the initial process. The high substrate concentration reduced water availability in the hydrolysis system and diffusion process, causing the substrate to become aggregated and inhibit the hydrolysis process.[Citation74] In short, the ESN hydrolysis reached its optimum state at 90 minutes with a DH% of approximately 10–11% by enzyme alcalase in optimum conditions.
Solubility of raw ESN and its improvement as ESN hydrolyzate
illustrates the total solid solubility of raw ESN (ER) and hydrolyzates of different hydrolysis period. The investigation is performed in three (3) different concentration (i.e. 2.5%, 5%, and 10% wt/vol) for accuracy. depicts ER’s cloudy appearance after soaking and heating during the double-boiling process. The exact solution for this condition is the soluble ESN hydrolyzate. The total solid solubility (TSS) in shows that ER has the lowest TSS value compared to ESN hydrolyzates. The double-boiling process in ER caused the insoluble ESN SiaMuc-glycoprotein to become partially soluble with the release of bioactive SiaMuc-glycopeptide and peptide. According to Halimi et al.,[Citation75] the ESN solubility increases with temperature and time. Solubility depends on the polarity distribution of amino acids on the protein surface and the thermodynamic interaction of protein-water molecules. The ESN SiaMuc-glycoprotein binds easily with water when the protein unfolds from the tertiary and secondary structures with a higher degree of hydrophilic and hydrogen network. Through physical heat treatment, the ESN molecules unfold into its simpler forms and appear to be partially soluble in water. In other words, the ESN bioaccessibility is increased with heat. However, Hui Yan et al.[Citation76] claimed that ER does not completely dissolve in water even after hours or days of heating and soaking. It would remain in the form of a swelled sponge without any further changes. Current research also observed a similar occurrence in ER which had been soaked overnight and double-boiled for 30 minutes. It is therefore concluded that ESN is naturally insoluble in water.
Figure 2. Total solid solubility (TSS) of raw ESN and hydrolyzates at concentration of 2.5%, 5.0% and 10.0% (wt/vol).The TSS of each sample is investigated in three (3) different concentration, i.e. 2.5%, 5.0% and 10%.a, b, c Superscript letters shows significantly higher/lower mean values comparing among samples for the same concentration at confidence level of 95% (n = 3). Note: ESN represents edible swiftlet’s nest, ER represents raw ESN and; EH-1, EH-2, EH-3 and EH-4 represent ESN hydrolyzates with specific hydrolysis period.
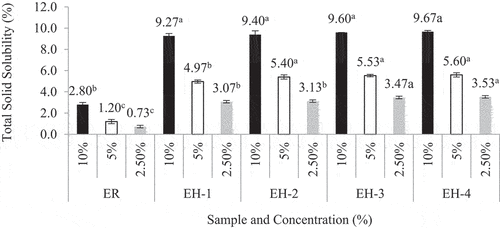
Figure 3. The appearance of double boiled raw ESN (ER) with overnight soaking followed by heat treatment (left) and; ESN hydrolyzate that is double boiled and continue with 1-hour hydrolysis incubation period (right).
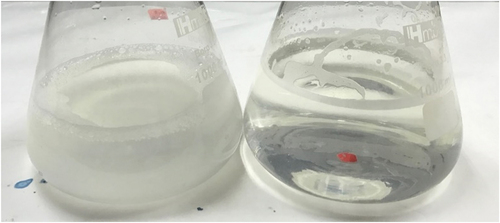
This conclusion is also a generally recognized property of secretary mucins.[Citation77,Citation78] Glycoprotein commonly exists with O-glycosidic properties.[Citation62,Citation79,Citation80] However, studies also reviewed that the glycan part of the glycoprotein provides water-holding capacity and assists in enzyme resistance. Thus, the glycoprotein is partially soluble and exists in high viscose state, for example, in saliva, egg white, and white plasma. As previously mentioned, ESN is produced from the swiftlets’ dried salivary mucins, which is partially soluble, and its viscose secretion. Naturally, ESN is also constructed mainly by glycoprotein.[Citation81] This feature explains the swelled ER after soaking and heating. It also describes the ESN hydrolysis’ ability to produce soluble hydrolyzate with a certain level of viscosity. reveals that the TSS of ESN hydrolyzates increases by three folds compared to ER. This relates to the protein structure, peptide, and amino acid within the ESN SiaMuc-glycoprotein. Studies by Brändén and Tooze,[Citation82] Creighton,[Citation83] Lesk,[Citation84] and Voet et al.[Citation85] support this finding. For example, simple protein and globular proteins are usually soluble or partially soluble and coagulable by heat. These proteins can be found in egg white, milk, blood serum, wheat leucosin, pea’s legumelin, and more. The same is applied for ESN sourced from secretary mucin that contain albuminous and globular proteins, which explains ESN’s solubility properties.[Citation86]
The protein solubility is also related to its peptides and amino acids. It also relies on the hydrophilic degree, the component folding,[Citation87] and the thermodynamic peptide–water interaction.[Citation88] The peptide solubility depends on its amino acids’ physical properties of peptide.[Citation89,Citation90] Amino acids can be categorized as basic, acidic, polar, and non-polar. Polar amino acids are hydrophilic and able to form hydrogen bond network with water molecules. These characteristics explain the polar hydrophilic amino acids, peptides, and especially peptide chain with less than 5 units of amino acids that can dissolve in aqueous solution such as sterile water. Moreover, hydrophilic peptide with less than 25% of hydrophobic amino acids and more than 25% charged residues such as D, K, R, H, and E are usually soluble. Hydrophilic amino acids include arginine, asparagine, aspartate, glutamine, glutamate, lysine, serine, threonine, hydroxyproline, tyrosine, histidine, cysteine, and tryptophan are polar and able to bond with water molecules at the hydrogen ends.[Citation91] The hydrophilic amino acids are similar in quantity with the hydrophobic amino acids from the amino acid profile of ESN and hydrolyzates () at approximately 31–34%. This explains the solubility of ESN toward its biological properties.
Table 2. Amino acid profile of ESN and hydrolyzates in percentage (%)
Raw ESN and hydrolyzates reveal a similar amount of amino acids among the samples but a significant difference in solubility. This feature is related to the glycoprotein structure and the folding of protein, peptide or amino acids present within the ESN. The insoluble ER has a complex macro-protein structure and limited exposure of hydrophilic peptide and amino acids. For the ESN hydrolyzates that underwent the enzyme hydrolysis process, the protein structure was unfolded and therefore exposed more hydrophilic peptides and amino acids that promoted the formation of hydrogen bond with water molecule, thus contributing to its solubility based on hydrolysis time. However, an absolute solubility will never be achieved due to the inhibition of peptide–peptide interaction and the existence of certain macro-glycoprotein that cannot be hydrolyzed.[Citation88]
Contents of ESN and hydrolyzates
show that ESN and hydrolyzate contain glycoprotein, glycopeptide, protein, peptide, polysaccharide, reducing sugar, and sialic acid. The assays were accessed based on 1.0 mg of sample at 1.0% mixture with water. Among these contents, glycoprotein, glycopeptide, and peptide have higher proportions, making them the primary content in ESN and hydrolyzates.
Figure 4. Glycoprotein, soluble protein, peptide, carbohydrate, reducing sugar and sialic acid content (mg/mL) of ESN and hydrolyzates.a, b, c Superscript with unlike letters shows significantly higher/lower in comparing among samples at confidence level of 95%. (n = 3). Note: The assays are carried out based on 1.0 mg/mL of ESN. ESN represents edible swiftlet’s nest, ER represents raw ESN and; EH-1, EH-2, EH-3 and EH-4 represent ESN hydrolyzates with specific hydrolysis period.
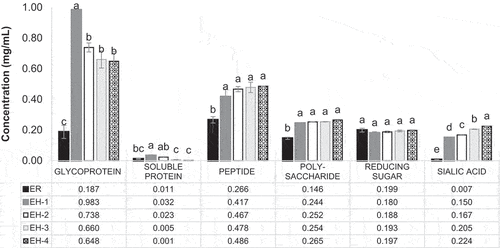
Glycoprotein
The ESN glycoprotein content is quantified by the periodic acid Schiff (PAS) method, and the result is shown in . The PAS method is widely used in quantification of macro-polysaccharides compound such as glycoprotein and glycopeptide.[Citation92] The PAS reagent oxidizes glyco-compound with periodic acid and stains with Schiff reagent, which creates a magenta pink liquid. In , raw ESN (ER) has the lowest glycoprotein content (p ≤ .05). This is related to ER’s insolubility that limits glycoprotein accessibility. Studies reported that glycoprotein accessibility is influenced by the solubility, polarity, and thermodynamic interaction.[Citation87,Citation88] For ER, the thermodynamic interaction in the overnight soaking and double-boiling process allowed the ESN structure to open up and swell in water, thus increasing the bioaccessibility of the glycoprotein content in ESN.[Citation76] However, a low glycoprotein content was observed due to the incomplete expression of complex macro-glycoprotein in the insoluble ER.
For ESN hydrolyzates, the glycoprotein content decreased as the hydrolysis period increased. Additionally, EH-1 exhibited the highest glycoprotein content compared to EH-2, EH-3, and EH-4. This is because EH-1 underwent an incomplete hydrolysis and created a longer chain soluble glycoprotein and glycopeptide. In contrast, EH-3 and EH-4 experienced a maximized hydrolysis state, therefore contain a higher quantity of glycopeptide instead of glycoprotein. A similar result was also obtained by Khushairay et al..[Citation19]
As the hydrolysis period increases, the glycopeptide content overtakes the glycoprotein content due to the complete hydrolysis process. Hur et al.[Citation93] claimed that the strength of glycosidic linkage in glycoprotein and glycopeptide determined the strength of the compound. The increasing hydrolysis period in EH-2, EH-3 and EH-4 that has reduced the chain length, and the structure of glycoprotein/peptide has weakened the glycosidic bonding within the glycoprotein/peptide. Thus, the glycoprotein content in ESN hydrolyzates decreased with an increasing hydrolysis period. In addition, the PAS assay was considered to be efficient for the quantification of glycoprotein as a macro-molecule of polysaccharides instead of the glycoepeptide. Nevertheless, the Schiff reagent binding was less compatible to the glycopeptide due to low molecular weight (MW), simpler peptide structure, shorter peptide chain length, and further attached with large glycan molecule.[Citation94] Thus, the decreasing glycoprotein content in represents a decreasing glycoprotein content albeit an increasing glycopeptide content. Conclusively, the ESN hydrolysis is directly proportional to the glycoprotein content, reflecting the effect on glycopeptide inversely. In other words, enzymatic hydrolysis increases the nutritional bioavailability of ESN in the form of SiaMuc-glycopeptide.
Protein, peptide, and amino acid
Soluble protein: Previously, proximate analysis reported that the ESN protein content was approximately 60%. However, the insoluble ER in reveals a relatively low soluble protein content at approximately 0.011 mg/mL. This was caused by the complex hydrophobic protein structure that had trapped the protein content within its macro-molecule. Because of this, the Bradford assay could not access the carboxyl and amino group of the soluble protein. Kruger[Citation95] stated that the Bradford assay does not react well with free amino acid and peptide with the molecular weight (MW) lower than 3 kDa. Previous reports claimed that ESN has a protein MW of 21.2–140.8 kDa, and glycoprotein a varied MW between 64.8 and 140.8 kDa.[Citation96] The ESN hydrolysis process enzymatically breaks down SiaMuc-glycoprotein and protein into SiaMuc-glycopeptides and free peptides with lower MW more than 31.4 kDa.[Citation97] This confirms the Bradford’s efficiency in measuring protein in ESN hydrolyzates. However, these proteins and peptides exist in the form of glycoprotein and glycopeptide. Studies reported that glycosylation interferes with the Bradford assay and consequently causes protein content underestimation.[Citation98]
also illustrates a significantly higher content of soluble protein in EH-1 and EH-2 than in other samples (p ≤ .05). Contrastingly, EH-3 and EH-4 show a statistically lower protein content than ER. This result ensures the effectiveness of the enzyme hydrolysis on the ESN SiaMuc-glycoprotein. As previously mentioned, the ESN hydrolyzates solubility increased throughout the hydrolysis period. The breakdown of ESN protein into peptides improved the protein solubility and bioaccessibility of the hydrophilic protein structure and its residues. This helped in binding the Coomassie dye in the Bradford reagent, therefore increasing the detection efficiency of the protein content in EH-1 and EH-2. Based on , ESN achieves the maximized DH at 1.5 hours (90 minutes) and above. This is in line with the decreasing trend of the soluble protein content throughout the increasing hydrolysis period, in which EH-3 and EH-4 contain glycopeptides and peptides with lower MW. Thus, a lower concentration of soluble protein content was detected due to long hydrolysis hours.
Peptide: The peptide content of ESN and hydrolyzate is summarized in . Overall, the ESN peptide content was relatively higher than the soluble protein content, especially after hydrolysis. Statistically, the peptide content in ER was lower than EH-1, EH-2, EH-3, and EH-4 (p ≤ .05). However, there was no significant difference found in the peptide content of EH-1, EH-2, EH-3 and EH-4 (p ≤ .05).
Based on Perez-Vilar and Hill,[Citation99] mucin is constructed of polypeptide chains with glycosidic linkage. It is the swiftlet’s salivary gland’s natural properties that produce the peptide in ESN. The raw ESN is usually soaked overnight and double boiled, which then expands its structure.[Citation11,Citation100] The complex and unfold hydrophilic protein in ESN breaks down into simpler peptides due to physiological factors, such as water and heat.[Citation88] This thermodynamic breakdown of ESN SiaMuc-glycoprotein resulted in the formation of SiaMuc-glycopeptide, free peptide, and amino acids, thus positively enhancing the OPA assay accessibility. Therefore, it is undeniable that the raw ESN has a significantly lower peptide content than the hydrolyzates. Whereas, the hydrolyzates have a significantly higher peptide content because of the enzymatic treatment that has produced simpler and soluble ESN SiaMuc-glycopeptides.
reveals that the ESN hydrolyzate’s peptide content increased with the hydrolysis period with a trend similar to the degree of hydrolysis (DH). A similar result is also reported by Tang et al.[Citation101] previously. The rise was caused by the changes in glycoprotein structure, molecular weight, solubility, and properties. The solubility increased with the hydrolysis period due to the unfolding structure and the glycopeptide and peptide exposure that enhanced the amine group accessibility in the peptide content.[Citation100,Citation102] Equivalently in the ESN hydrolyzate, the ESN SiaMuc-glycopeptide hydrophilic property promoted peptide–water interaction and enhanced solubility by the formation of hydrogen bonding.
Through the enzymatic technology, the physiologically inactive SiaMuc-glycoprotein was hydrolyzed and released into the bioactive ESN SiaMuc-glycopeptide and free peptide by alcalase. Similarly to the DH trend, the peptide content entered a stationary phase from 2 hours of hydrolysis at approximately 0.90 mg/mL. This is related to the achievement of maximized DH for ESN at 1.5 hours (90 minutes). The slower hydrolysis rate of 2 hours incubation and more was arguably caused by the inhibition of high substrate concentration (i.e., peptide) and the reduction of peptide bond availability and accessibility. These phenomena have led to a less increment of peptide content in EH-3 and EH-4. The sample EH-1 reviewed a slightly lower peptide content than other hydrolyzates (EH-2, EH-3 and EH-4). This can be explained with the incomplete breakdown of macro-protein in the first hour of hydrolysis that revealed a lower solubility and bioaccessibility in the OPA assay. Conclusively, the ESN peptide content was directly proportional to the hydrolysis period that reflects on the DH, solubility, and protein structure. In other words, enzymatic hydrolysis increases the nutritional bioavailability of ESN in the form of bioactive SiaMuc-glycopeptide and free peptide.
Amino acid profile: shows that ER and hydrolyzates consist of various amino acids, including 10 types of essential amino acids (EAA) and nine non-essential amino acids (NEAA). The results also revealed that ESN and hydrolyzates have a high concentration of aspartate, serine, valine, threonine, proline, and leucine. This confirms previous findings by Wu et al.[Citation103] and Chua et al.[Citation104] Furthermore, the World Health Organization (WHO) reported that EAA is a basic necessity for human health yet cannot be produced naturally by the human body.[Citation105,Citation106] Meanwhile, NEAA indicates the opposite. The NEAA usually can be naturally synthised by the human body; therefore, they are not mandatory in our diet.
The results in reveal the amino acid profile of ER and ESN hydrolyzates in terms of quantity and quality. In overall, the amino acids content of ER and ESN hydrolyzates are similar. This finding indicates that the ESN alcalase hydrolysis had no alteration and treatment effect on the ESN SiaMuc-glycopeptide amino acid sequences. This is related to the specificity of alcalase that acted on the specific peptide–peptide bond, thus reserving the amino acid profile and properties of the ESN. However, it was also observed that the quantity of certain amino acids have statistically increased with hydrolysis application. These include the essential amino acids, such as valine, tyrosine, phenylalanine, lysine, leucine, and the total amount of EAA. This is in consistent with Ng et al.[Citation107] who reported that the ESN enzymatic hydrolysis increased the quantity of essential amino acids. In which, the enzymatic biotechnology applied and the process that involved filtration and removal of impurities thus increased the amino acids composition and purity. As ESN and hydrolyzate is a potential processing step for “consumer product” development, where the ESN hydrolyzate is consumed as a whole material, the amino acids of ESN hydrolyzates play an important role as a matrix of its nutritional functionality.
As stated in , the EAA and NEAA of ESN is equipotential with other protein sources, such as milk, beef, egg, chicken, pork, and soybean. Edible swiftlet’s nest consists of all amino acids found in animal protein with an addition of hydroxyproline and tryptophan, which is typically absent in animal-type protein. Contrary to the protein in plants, such as soybean, ESN and hydrolyzates have high concentrations of numerous amino acids. These benefits make ESN a unique source of protein because of its complete amino acid nutrients that are beneficial to health and suitable for all consumers. Therefore, the study highlighted that ESN and hydrolyzates that existed in the form of glycopeptides is comparable to other pure protein sources. In fact, ESN and hydrolyzates contains specific sequence of amino acids that is bioactive particularly in antioxidant capacity.[Citation111] Aside from the amino acids, glycan part of ESN glycopeptides as a whole are also riched in sialic acid and epidermal growth factor (EGF). These key functionality components of ESN are significant value of ESN compared to other protein sources.
Table 3. Categories of amino acids in raw ESN and hydrolyzates
Table 4. Amino acid profile of ESN and hydrolyzate; and other food sources.[Citation108–110]
also reveals that the hydrophobic and hydrophilic amino acids in ESN and hydrolyzates are similar in concentration at approximately 31–35%, respectively. The hydrophilic amino acids in ESN and hydrolyzates include arginine, asparagine, aspartate, glutamate, lysine, serine, threonine, hydroxyproline, tyrosine, histidine, cysteine, and tryptophan with polar potential that assist in hydrogen networking in water.[Citation91] This explains ESN’s swelling capacity in water and its ability to be hydrolyzed into soluble bioactive hydrolyzate. The hydrophilic amino acids sequences and concentration influenced the degree of hydrophilic of the ESN component itself. These properties are the backbone of the ESN SiaMuc-glycoprotein structure, thermodynamic interaction, solubility, and degree of hydrolysis (DH), thus further determines the application of ESN and hydrolyzates in many industries.
The amino acid profile of ESN and hydrolyzate also revealed the hydroxyproline content, which is typically found in collagen and gelatin products. In the medical field, hydroxyproline is widely applied as a diagnostic marker in bone turnover, and in treating liver fibrosis and superficial wounds. This finding supports Chua et al.,[Citation104] Klein,[Citation112] and Hascoët et al.[Citation113] who reported that ESN contains collagen. Edible swiftlet’s nest is also well known for its antioxidant properties.[Citation111,Citation114] Studies reported that cysteine, methionine, tryptophan, histidine, tyrosine, glycine, alanine, serine, proline, and lysine are anti-oxidative amino acids.[Citation115–117] These anti-oxidative amino acids are one of the contributors for anti-oxidation ability. The presence of anti-hypertensive and anti-diabetic amino acids is also observed in ESN. They are cysteine, arginine, tryptophan, glutamate, and leucine. These amino acids weaken insulin resistibility in glucose metabolism and eventually reduce oxidative stress, decrease vascular intracellular calcium, and increase nitric oxide production, therefore enhances the endothelial function and reduces the peripheral vascular resistance. These would cause the lowering of the blood pressure, resulting in anti-hypertensive activity.[Citation118] This finding is in agreement with the positive result of anti-hypertensive study in ESN.[Citation11,Citation114] The amino acid profile also found that ESN consists of prebiotic potential amino acids, such as alanine, aspartate, glutamate, glycine, isoleucine, leucine, proline, serine, threonine, and valine.[Citation119–122] This result substantiates recent studies on ESN and hydrolyzate as a potential prebiotic ingredient.[Citation12,Citation13]
Glycan content quantification – polysaccharide, reducing sugar, and sialic acid
Polysaccharide: Carbohydrate is another primary content in ESN in a relatively abundant quantity. The carbohydrate from the ESN SiaMuc-glycoprotein, also known as glycan. In which, glycoprotein is derived from the glycosylation between glycan sugar and protein that naturally exist in the swiftlet’s salivary mucin.[Citation97] reveals that the ESN’s polysaccharides content increased significantly with alcalase hydrolysis (p ≤ .05), whereby ER exhibited the lowest content of carbohydrate compared to the soluble ESN hydrolyzates. Oritiz and Wagner[Citation123] stated that protein solubility relies on the exposure of its structure. The insoluble ER has its disturbance in exposing of its hydrophilic properties and functionality which contribute in lower quantity of polysaccharide content.
The ESN also consists of N- and O-glycosylated proteins with majority sialic acid-rich O-glycoproteins.[Citation81] According to Cole and Smith,[Citation124] glycoproteins are bonded covalently with sugar units known as glycan, which usually appear in the form of oligosaccharides. Just like other polysaccharide, oligosaccharide is also accessible with PHS assay.[Citation125,Citation126] These oligosaccharides in ESN typically includes hexoses like mannose, galactose, fucose, glucose, N-acetylhexosamine, as well as sialic acid (N-acetylneuraminic acid).[Citation104] The polysaccharide content in ESN is the contribution of the sialic acids, such as N-acetylglucosamine, N-acetylgalactosamine, oligosaccharides, and simple sugars, such as fucose and galactose. Similar results are reported in previous studies which ESN glycan consists of 9% sialic acid (N-acetylneuraminic acid); 7.2% of N-acetylgalactosamine; 5.3% of N-acetylglucosamine; oligosaccharides, 16.9% of galactose; 0.7% of fucose; and other monosaccharides, such as mannose; glucose, rhamnose; and xylose.[Citation127,Citation128]
For ESN hydrolyzates, the hydrolysis results in glycopeptide as primary content instead of glycoprotein. Glycan provides protection and resistance toward proteolysis.[Citation124] The alcalase in hydrolysis of ESN is a protease that leaves the sugar part remain untouched. However, the hydrolysis process has enhanced the exposure of glycan from the complex glycoprotein structure by strengthening the thermo-dynamic water interaction without hydrolyzing the glycan-oligosaccharide. With enhanced solubility, the polysaccharides in hydrolyzates were easily accessed with less disturbance and limitations due to its shorter peptide chain post-hydrolysis. Therefore, the ESN hydrolyzate revealed a higher polysaccharide content than the ER. Conclusively, ESN hydrolysis increases the bioavailability and bioaccessibility of polysaccharide content in ESN glycan.
Reducing Sugar: The reducing sugar content of ER and hydrolyzates in shows a similar quantity. This is the contribution of the oligosaccharides and monosaccharides, such as glucose, galactose, and fucose within the ESN glycan. Generally, reducing sugar comprises monosaccharides such as glucose, galactose, and fructose, and also certain disaccharides, oligosaccharides, and polysaccharides. Reducing sugar with free carbonyl group (C = O) consists of reducing functionality created by free aldehyde (-COH) or free ketone group, which then contribute in the formation of carboxyl group (-COOH).[Citation129] The reducing sugar content of ESN is brought by the ketone and aldehyde group within the glycan and protein of ESN SiaMuc-glycoprotein.
According to Shylaja and Seshadri[Citation62] and Zhao et al.,[Citation130] glycoproteins are compounds where glycan-sugar covalently bond to protein, and the glycan part is made of oligosaccharides.[Citation81,Citation131] Studies reviewed that most of the oligosaccharides, monosaccharides, glucose polymer, starch, cellulose, and copolymers (e.g chitin) possess reducing chain of aldehyde or ketone group.[Citation132,Citation133] The glycan contains ketone and aldehyde groups within its components, thus contributing to the reducing sugar content in ER and hydrolyzates.
Studies also proposed that the five monoses in ESN also contribute to reducing sugar content due to the presence of carbonyl carbon group.[Citation134] These mannoses are the dominant monosaccharides in N-linked glycosylation of ESN. They are the sialic acids (N-acetylneuraminic acid), N-acetylglucosamine, N-acetylgalactosamine, and some minor sugars, such as mannose and galactose. Nelson et al.[Citation134] stated that sialic acid is built by oligosaccharides which consists of an aldehyde group. The same occurs with N-acetylglucosamine and N-acetylgalactosamine. It is believed that these components also contribute to the reducing sugar content of ESN.
The amino acids in ESN also consists of both ketone and aldehyde groups. For instance, leucine and lysine are ketogenic. Isoleucine, phenylalanine, tyrosine, and tryptophan are partially ketogenic.[Citation135,Citation136] Tillery et al.[Citation137,Citation138] reported that amino acids are organic compounds consisting both the amino and carboxyl groups (-COOH) in which the carboxyl group consists of both carbonyl and hydroxyl groups that represent the existence of an aldehyde group (-COH) (Phschool, 2017). These ketone and aldehyde groups attach to the amino group of amino acids, therefore interfering in the DNS assay, and consequently detected as reducing sugar. Similar phenomenon is reported by Teixeira et al.[Citation139] on the interference of amino acids in DNS assay, in which tryptophan, cysteine, histidine, tyrosine, and hydroxyproline results in overestimation of reducing sugar content by 75%, 50%, 35%, 18%, and 10%; and methionine results in lowering the reducing sugar content by 5%. Glycan also contributes to the reducing sugar content of ESN.
For ESN hydrolyzates, the reducing sugar content had no significant difference throughout the hydrolysis period. The alcalase had not affected the glycan-peptide bonding but instead cleaved on the peptide–peptide bond.[Citation140] This made the glycan-oligosaccharide of ESN SiaMuc-glycopeptide remained untouched. In addition, the unchanged amino acid profile in all ESN hydrolyzates indicates that alcalase cleave specifically without breaking down the peptide into amino acids. This decrease the bioaccessibility of aldehyde and ketone group within the amino acid which interferes the DNS assay. Hence, the natural reducing sugar in ESN remained in a similar concentration regardless of the alcalase hydrolysis period.
Sialic Acid: shows that the hydrolysis had significantly increased the sialic acid content in ESN (p ≤ .05). According to Halimi et al.,[Citation75] the carbohydrate in ESN is contributed by sialic acid (N-acetylneuraminic acid), N-acetylgalactosamine, N-acetylglucosamine, galactose, and fucose. Sialic acid is the primary carbohydrate component within the ESN glycan at approximately 9%. The N-acetylneuraminic acid is an amino acid bonded with acetyl and glycolyl group.[Citation141] They function mainly in the brain’s neurological and intellectual aspects.[Citation127,Citation142]
Sialic acids exist within the glycoproteins and gangliosides of animal tissues, fungi, yeast, and some bacteria. They are mostly found bonded to the glycan part of glycoproteins. A similar phenomenon occurs for the sialic acid in ESN SiaMuc-glycoprotein, whereby the sialyl-sugar chains together with the protein molecule.[Citation104] From the result, ER revealed a significantly lower sialic acid content than the ESN hydrolyzates (i.e. EH-1, EH-2, EH-3, and EH-4). This indicates that the soaking and heating process merely expanded the ESN SiaMuc-glycoprotein structure, yet the process was less efficient in increasing the bioavailability of sialic acid within the compound.[Citation11,Citation100] Enzymatic hydrolysis broke down the ESN SiaMuc-glycoprotein at the peptide–peptide bond without destroying the glycan, leaving it remain untouched.[Citation114,Citation140] The glycopeptide released from hydrolysis process has a simpler structure with increased solubility, thus increasing the sialic acid’s bioaccessibility attached within the glycan.[Citation141,Citation143] Conclusively, the hydrolysis period was directly proportional to the sialic acid content in ESN.
Antioxidative activity: DPPH, FRAP, and ABTS scavenging activity
revealed that ESN and hydrolyzates has a free radical scavenging activity (DPPH) activity of 25–30%. Whereby, a significant higher DPPH activity is observed in the ESN hydrolyzate (EH1 & EH2). While, ER has a statistically lower DPPH activity. This indicates that application enzymatic action on ESN that produced ESN in the form of bioactive glycopeptides enhanced the antioxidative activity of ESN. This is probably related to the unfolding and breaking down of the insoluble macro-glycoprotein, thus increased the bioaccessibility of the hydrophobic bioactive peptides exists within the ESN molecules. Similar results was reported in previous study by Gan et al.[Citation56] and Ling et al.[Citation144] as well. However, the findings also revealed that EH3 and EH4 with longer hydrolysis period revealed a slight decrease in DPPH activity. In which, long hours of hydrolysis on ESN glycoprotein may negatively affect the antioxidative activity of ESN due to long hours of heating that leads to oxidization, denaturation, as well as further breaking down of anti-oxidative peptides by the enzymatic activity.
Figure 5. Antioxidative capacity (DPPH, FRAP, & ABTS) of ESN and hydrolyzates.a, b, c Superscript with unlike letters shows significantly higher/lower in comparing among samples within the same antioxidative assay at confidence level of 95%. (n = 3). Note: The assays are carried out based on 1.0 mg/mL of ESN. ESN represents edible swiftlet’s nest, ER represents raw ESN and; EH-1, EH-2, EH-3 and EH-4 represent ESN hydrolyzates with specific hydrolysis period.
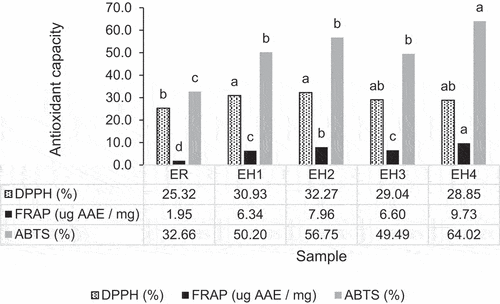
Overall, the ferric reducing antioxidant power (FRAP) value of ESN significantly increased after the application of enzymatic hydrolysis. In which, ER showed the lowest FRAP value and EH1, EH2, EH3, and EH4 have a significantly higher FRAP value. Among the ESN hydrolyzates, the findings also indicates that longer hydrolysis hour increased the FRAP value of ESN. Whereby, EH4 has the highest FRAP value; and EH1 has the lowest FRAP value. This is also reported in the previous studies where enzymatic hydrolysis released and exposed more electron-riched amino acids, thus increase the FRAP value of ESN after hydrolysis.[Citation56]
The results of ABTS in also revealed similar findings. Whereby, the ABTS scavenging activity of ESN is significantly enhanced with enzymatic hydrolysis. In terms of period, the longer period of enzymatic hydrolysis increases the ABTS activity of ESN. Thus, EH4 with 4 hours of hydrolysis incubation period revealed the highest ABTS activity at 64.02%. Meanwhile, ER showed the lowest ABTS activity due to insolubility and low bioaccessibility of hydrophilic amino acids in ESN glyco-molecules.
Conclusion
The application of enzymatic technology on ESN has resulted in the production of bioactive ESN hydrolyzate. Through hydrolysis, the ESN SiaMuc-glycoprotein was converted to simpler SiaMuc-glycopeptide and free peptide. The process released bioactive glycopeptide and peptide (BAGP, BAP) from its inactive core glycoprotein. The degree of hydrolysis (DH) concluded that a 60- to 90-min hydrolysis process was required to completely breakdown the ESN SiaMuc-glycoprotein to achieve maximum DH. Further hydrolysis was unnecessary due to insignificant changes in DH, solubility, protein, peptide, and glycoprotein content. Throughout the hydrolysis process, the physicochemical properties of ESN was influenced positively, which eases industry utilization. The solubility of ESN hydrolyzate (EH-1) was significantly improved by three folds (p ≤ .05) compared to the double-boiled raw ESN (ER). The ESN with hydrolysis period of 2 hours and more has similar solubility. The ESN physicochemical properties indicated that hydrolysis decreased glycoprotein and protein content; increased the content of bioactive glycopeptide and peptide; and allowed the bioaccessibility of glycan-polysaccharide, reducing sugar, and sialic acid. The glycopeptide and peptide content was directly proportional to the hydrolysis period until a maximized DH was achieved, while the ESN hydrolyzates’ glycan (polysaccharide, reducing and sialic acid) remained in a similar quantity regardless of the hydrolysis period. Interestingly, the ER amino acid profile and hydrolyzates proposed that the ESN hydrolysis had not influenced the quantity and quality of the amino acids in ESN. In other words, the ESN hydrolyzates provided a high concentration of beneficial amino acids with high solubility and increased nutritional bioavailability and bioaccessibility. These findings confirmed that ESN hydrolyzate improves nutritional functionality and physicochemical properties for a broader range of industrial applications. As a versatile product and material, a small quantity of ESN is required for a maximized and optimized functionality; therefore, the utilization of bioactive ESN hydrolyzate is more economical and affordable compared to ER. For a preliminary investigation of ESN hydrolyzate, further understanding of ESN hydrolyzate such as mineral content, cytotocity study, morphological study, and structural characteristics may be helpful in terms of industrilisation, utilization, and application.
Highlights
ESN was enzymatically hydrolyzed by alcalase for four different hours.
ESN hydrolysis produces bioactive ESN SiaMuc-glycopeptide hydrolyzate (ESNh).
Maximum degree of hydrolysis of ESN was achieved at 60 to 90 min.
Hydrolysis of ESN increases solubility and bioavailability of its nutrients.
Physicochemical of ESNh showed increased in glycopeptide, peptide, and sialic acid.
Credit authorship contribution statement
Tan Hui Yan: Experimentation, Writing.
Sue Lian Mun: Experimentation, Writing
Jia Lin Lee: Experimentation
Seng Joe Lim: Conceptualization, Supervision
Nur’ Aliah Daud: Experimentation
Abdul Salam Babji: Conceptualization, Supervision
Shahrul Razid Sarbini: Conceptualization, Writing, Supervision.
Acknowledgments
The authors would like to thank especially Dr. Muhamad Hanif Rawi, Joyce Morris Kapong, Su Rou Ng, Jing Yi Gan, and Ching Yee Chong who have contributed to this study. The authors are also grateful for the support from the Department of Crop Science, Faculty of Agricultural and Food Sciences, Universiti Putra Malaysia; Department of Food Sciences, Faculty of Science and Technology, Universiti Kebangsaan Malaysia; and Mobile Harvesters Malaysia Sdn. Bhd. in the completion of this study.
Disclosure statement
No potential conflict of interest was reported by the author(s).
Additional information
Funding
References
- Lee, M.-S.; Huang, J.-Y.; Lien, -Y.-Y., and Sheu, S.-C. The Rapid and Sensitive Detection of Edible Bird’s Nest (Aerodramus Fuciphagus) in Processed Food by a Loop-mediated Isothermal Amplification (LAMP) Assay. J. Food Drug Anal. 2019, 27, 154–163. DOI: 10.1016/j.jfda.2018.08.003.
- Guo, C.-T.; Takahashi, T.; Bukawa, W.; Takahashi, N.; Yagi, H.; Kato, K.; Kazuya, I.-P.; Miyamoto, D.; Suzuki, T.; Suzuki, Y. Edible Bird’s Nest Extract Inhibits Influenza Virus Infection. Antivir. Res. 2006, 70, 140–146.
- Lau, A. S.; Melville, D. S. International Trade in Swiftlet Nests with Special Reference to Hong Kong; Traffic International: Cambridge, United Kingdom, 1994.
- Chua, L. S.; Zukefli, S. N. A Comprehensive Review of Edible Bird Nests and Swiftlet Farming. J. Integr. Med. 2016, 14, 415–428. DOI: 10.1016/S2095-4964(16)60282-0.
- Gao, J.; Qiao, T.; Geng, J. The Nutritional Ingredients of Yanwo (Edible Birds Nest). Acta Pharmacol. Sin. 1988, 2, 019.
- Lim, C. K.; Cranbrook, G. G.-H.; Cranbrook, G. G.-H.; Zoologiste, G. B.; Cranbrook, G. G.-H., and Zoologist, G. B. Swiftlets of Borneo: Builders of Edible Nests; Kota Kinabalu, Malaysia: Natural History Publications Borneo, 2002; pp 171.
- Marcone, M. F. Characterization of the Edible Bird’s Nest the “Caviar of the East. Food Res. Int. 2005, 38, 1125–1134. DOI: 10.1016/j.foodres.2005.02.008.
- Ng, M. H.; Chan, K. H.; Kong, Y. C. Potentiation of Mitogenic Response by Extracts of the Swiftlet’s (Collocalia) Nest. Biochem. Int. 1986, 13, 521–531.
- Kong, Y.; Keung, W.; Yip, T.; Ko, K.; Tsao, S.; Ng, M. Evidence that Epidermal Growth Factor Is Present in Swiftlet’s (Collocalia) Nest, Comp. Biochem. Physiol. B. Comp. Biochem. 1987, 87, 221–226. DOI: 10.1016/0305-0491(87)90133-7.
- Chan, S. W. Review of Scientific Research on Edible Bird’s Nest; Hong Kong: Department of Applied Biology and Chemical Technology, Hong Kong Polytechnique University, 2006.
- Nurfatin, M.; Etty Syarmila, I.; Nur’Aliah, D.; Zalifah, M.; Babji, A. S.; Ayob, M. K. Effect of Enzymatic Hydrolysis on Angiotensin Converting Enzyme (ACE) Inhibitory Activity in Swiftlet Saliva. Int. Food Res. J. 2016, 23, 141–146.
- Daud, N. A.; Yusop, S. M.; Lim, S. J.; Babji, A. S. Physicochemical Properties of Glycan Extracted from Swiftlet’s Edible Bird Nest (Genus Collocalia) and its Potential as Prebiotic Component. In AIP Conference Proceedings, AIP Publishing LLC, Selangor, Malaysia, 2019; pp 050010. DOI: 10.1063/1.5111258.
- Daud, N. A.; Sarbini, S. R.; Babji, A. S.; Yusop, S. M.; Lim, S. J. Characterization of Edible Swiftlet’s Nest as a Prebiotic Ingredient Using a Simulated Colon Model, Ann. Microbiol. 2019, 69, 1235–1246. DOI: 10.1007/s13213-019-01507-1.
- Medway, L. The Antiquity of Trade in Edible Birds’ Nests. Federation Museum J. 1963, 8, 36–45.
- Sankaran, R. The Status and Conservation of the Edible-Nest Swiftlet (Collocalia Fuciphaga) in the Andaman and Nicobar Islands. Biol. Conserv. 2001, 97, 283–294. DOI: 10.1016/S0006-3207(00)00124-5.
- Wong, Z. C. F.; Chan, G. K. L.; Wu, L.; Lam, H. H. N.; Yao, P.; Dong, T. T.; Tsim, K. W. K. A Comprehensive Proteomics Study on Edible Bird’s Nest Using New Monoclonal Antibody Approach and Application in Quality Control. J. Food Compost. Anal. 2018, 66, 145–151. DOI: 10.1016/j.jfca.2017.12.014.
- Wang, C. C. The Composition of Chinese Edible Bird’s Nest and the Nature of their Proteins. J. Biol. Chem. 1921, 49, 429–439. DOI: 10.1016/S0021-9258(18)85979-8.
- Babji, A. S. Development of Downstream Products from Edible Bird Nest; Putrajaya, 2014.
- Khushairay, E. S. I.; Ayub, M. K.; Babji, A. S. Effect of Enzymatic Hydrolysis of Pancreatin and Alcalase Enzyme on Some Properties of Edible Bird’s Nest Hydrolysate. In AIP Conference Proceedings, AIP, 2014; pp 427–432.
- Capriotti, A. L.; Caruso, G.; Cavaliere, C.; Samperi, R.; Ventura, S.; Chiozzi, R. Z.; Laganà, A. Identification of Potential Bioactive Peptides Generated by Simulated Gastrointestinal Digestion of Soybean Seeds and Soy Milk Proteins. J. Food Compost. Anal. 2015, 44, 205–213. DOI: 10.1016/j.jfca.2015.08.007.
- Görgüç, A.; Özer, P.; Yılmaz, F. M. Simultaneous Effect of Vacuum and Ultrasound Assisted Enzymatic Extraction on the Recovery of Plant Protein and Bioactive Compounds from Sesame Bran. J. Food Compost. Anal. 2020, 87, 103424. DOI: 10.1016/j.jfca.2020.103424.
- Udenigwe, C. C.; Aluko, R. E. Food Protein-Derived Bioactive Peptides: Production, Processing, and Potential Health Benefits. J. Food Sci. 2012, 77, R11–R24. DOI: 10.1111/j.1750-3841.2011.02455.x.
- Fang, B.; Sun, J.; Dong, P.; Xue, C.; Mao, X. Conversion of Turbot Skin Wastes into Valuable Functional Substances with an Eco-Friendly Fermentation Technology. J. Clean. Prod. 2017, 156, 367–377. DOI: 10.1016/j.jclepro.2017.04.055.
- Dos S. Kimberle, P.; Carolina, M.-S.; Ana, I. S. B.; Luciana, R. B. G. Modifying Alcalase Activity and Stability by Immobilization onto Chitosan Aiming at the Production of Bioactive Peptides by Hydrolysis of Tilapia Skin Gelatin. Process Biochem. 2020, 97, 27–36. DOI: 10.1016/j.procbio.2020.06.019.
- Opheim, M.; Šližytė, R.; Sterten, H.; Provan, F.; Larssen, E.; Kjos, N. P. Hydrolysis of Atlantic Salmon (Salmo Salar) Rest Raw materials—Effect of Raw Material and Processing on Composition, Nutritional Value, and Potential Bioactive Peptides in the Hydrolysates. Process Biochem. 2015, 50, 1247–1257. DOI: 10.1016/j.procbio.2015.04.017.
- Shao, J.; Zhang, G.; Fu, J.; Zhang, B. Advancement of the Preparation Methods and Biological Activity of Peptides from Sesame Oil Byproducts: A Review. Int. J. Food Prop. 2020, 23, 2189–2200. DOI: 10.1080/10942912.2020.1849276.
- Li, N.; Lv, S.; Ma, Y.; Liu, N.; Wang, S.; Zhou, D. In Vitro Antioxidant and Anti-Aging Properties of Swim Bladder Peptides from Atlantic Cod (Gadus Morhua). Int. J. Food Prop. 2020, 23, 1416–1429. DOI: 10.1080/10942912.2020.1807565.
- Mohanty, D.; Jena, R.; Choudhury, P. K.; Pattnaik, R.; Mohapatra, S.; Saini, M. R. Milk Derived Antimicrobial Bioactive Peptides: A Review. Int. J. Food Prop. 2016, 19, 837–846. DOI: 10.1080/10942912.2015.1048356.
- Kurogochi, M.; Matsuda, A.; Mizuno, M. Preparation of O-Glycopeptides from Commercial Bovine Whey Proteins Using Offline Liquid chromatography–Mass Spectrometry. Carbohydr. Res. 2020, 491, 107981. DOI: 10.1016/j.carres.2020.107981.
- Ye, L.; Zhang, J.; Ye, X.; Tang, Q.; Liu, Y.; Gong, C.; Du, X.; Pan, Y. Structural Elucidation of the Polysaccharide Moiety of a Glycopeptide (GLPCW-II) from Ganoderma Lucidum Fruiting Bodies. Carbohydr. Res. 2008, 343, 746–752. DOI: 10.1016/j.carres.2007.12.004.
- Lee, B.-S.; Krisnanchettiar, S.; Lateef, S. S.; Lateef, N. S.; Gupta, S. Oligosaccharide Analyses of Glycopeptides of Horseradish Peroxidase by Thermal-Assisted Partial Acid Hydrolysis and Mass Spectrometry. Carbohydr. Res. 2005, 340, 1859–1865. DOI: 10.1016/j.carres.2005.04.018.
- Ramachandran, R.; Babji, A. S.; N.A. Sani. Antihypertensive Potential of Bioactive Hydrolysate from Edible Bird’s Nest, In AIP Conference Proceedings, AIP Publishing LLC, Selangor, Malaysia, 2018; pp 020099. DOI: 10.1063/1.5028014.
- Babji, A. S.; Sajak, A. A. B.; Daud, N. A.; Rahman, H. A.; Sermwittayawong, D., and Patninan, K. Potential Anti-Diabetic Activities from Edible Bird Nest and its Hydrolysates. Current Advances in Chemistry and Biochemistry. 2021, 3, 77–86. DOI:10.9734/bpi/cacb/v3/7819D.
- Nurul Nadia, M.; Babji, A. S.; Ayub, M. K.; Nur‘Aliah, D. Effect of Enzymatic Hydrolysis on Antioxidant Capacity of Cave Edible Bird’s Nests Hydrolysate. Int. J. Chemtech Res. 2017, 10, 1100–1107.
- Amiza, M. A.; Oon, X. X.; Norizah, M. S. Optimization of Enzymatic Hydrolysis Conditions on the Degree of Hydrolysis of Edible Bird’s Nest Using Alcalase® and Nutritional Composition of the Hydrolysate. Food Res. 2019, 3, 570–580.
- Khushairay, E. S. I.; Ayub, M. K.; Babji, A. S. Effect of Enzymatic Hydrolysis of Pancreatin and Alcalase Enzyme on Some Properties of Edible Bird’s Nest Hydrolysate. In AIP Conference Proceedings, AIP Publishing LLC, Selangor, Malaysia, 2014; pp 427–432. DOI: 10.1063/1.4895235.
- Horwitz, W., and George, W. L., Eds., Animal Feed. Official Methods of Analysis of AOAC International, 18th ed.; United State of America: Association of Official Analytical Chemistry International, 2005; pp 68.
- Kjeldahl, J. Neue Methode Zur Bestimmung Des Stickstoffs in Organischen Körpern. Zeitschrift Für Analytische Chemie. 1883, 22, 366–382. DOI: 10.1007/BF01338151.
- Julius, B., and Cohen, J. B. Kjeldal Method to Measure Nitrogen. Practical Organic Chemistry. 1910.
- Harwood, M. L., and Moody, C. J. Experimental Organic Chemistry: Principles and Practice; New Jersey: Blackwell Scientific Publications, 1989. pp 122–125.
- Soxhlet, F. Die Gewichtsaiialytische Bestimmung Des Milchfettes; Von. Dingler's Polytechnisches Journal. 1879; 232, 461–465.
- Humphries, E. C. Mineral Components and Ash Analysis. In Moderne Methoden der Pflanzenanalyse/Modern Methods of Plant Analysis; Berlin, Heidelberg: Springer, 1956; pp 468–502.
- Babji, A. S.; Nurfatin, M. H.; Etty Syarmila, I. K.; Masitah, M. Secrets of Edible Bird Nest, UTAR Agric. Sci. J. 2015, 1, 32–37.
- Zainab, H.; Nur Hulwani, I.; Jeyaraman, S.; Kamarudin, H.; Othman, H.; Lee, B. B. Nutritional Properties of Edible Bird Nest. J. Asian Sci. Res. 2013, 3, 600–607.
- Norhayati, M.; Azman, O., and Nazaimoon, W. Preliminary Study of the Nutritional Content of Malaysian Edible Bird’s Nest. Malays. J. Nutr. 2010, 16, 389–396.
- Garcia-Segura, L. M.; Martinez-Rodriguez, R.; Martinez-Murillo, R.; Bogonez, E.; Toledano, A. Glycoproteins and Polyanions in the Synapses of Rat and Mouse Central Nervous System. Acta Histochem. 1978, 61, 89–97. DOI: 10.1016/S0065-1281(78)80053-1.
- Babji, A. S. Bioactive swiftlet nest supplement, WO2017034390A2, 2017. https://patents.google.com/patent/WO2017034390A2/en?oq=WO+2017%2f034390+A2 (accessed May 2, 2019).
- Adler-Nissen, J. A Review of Food Hydrolysis Specific Areas; Enzymic Hydrolysis of Food Proteins., Elsevier applied science publishers, 1986.
- Garner, D.; Crisosto, C. H.; Wiley, P.; Crisosto, G. M. Measurement of Soluble Solids Content, Measurement of PH and Titratable Acidity. Cent. Valley Postharvest Newsletter. 2008, 17, 2–4.
- Bradford, M. M.; Williams, W. L. Protein-Assay Reagent and Method, 4,023,933. 1977. https://patents.google.com/patent/US4023933A/en.
- Seo, -S.-S. High Performance Liquid Chromatographic Determination of Homocysteine and Cystathionine in Biological Samples by Derivatization with 6-aminoquinolyl-n-hydroxylsuccinimidyl Carbamate (AQC). J. Korean Chem. Soc. 2005, 49, 278–282. DOI: 10.5012/jkcs.2005.49.3.278.
- Dubois, M.; Gilles, K. A.; Hamilton, J. K.; T Rebers, P.; Smith, F. Colorimetric Method for Determination of Sugars and Related Substances. Anal. Chem. 1956, 28, 350–356. DOI: 10.1021/ac60111a017.
- Saqib, A. A. N.; Whitney, P. J. Differential Behaviour of the Dinitrosalicylic Acid (DNS) Reagent Towards Mono-and Di-Saccharide Sugars. Biomass Bioenergy. 2011, 35, 4748–4750. DOI: 10.1016/j.biombioe.2011.09.013.
- Jourdian, G. W.; Dean, L.; Roseman, S. The Sialic Acids XI. A Periodate-resorcinol Method for the Quantitative Estimation of Free Sialic Acids and their Glycosides. J. Biol. Chem. 1971, 246, 430–435. DOI: 10.1016/S0021-9258(18)62508-6.
- Mantle, M.; Allen, A. A Colorimetric Assay for Glycoproteins Based on the Periodic acid/Schiff Stain; Portland Press Limited: United Kingdom, 1978.
- Gan, J. Y.; Chang, L. S.; Mat Nasir, N. A.; Babji, A. S.; Lim, S. J. Evaluation of Physicochemical Properties, Amino Acid Profile and Bioactivities of Edible Bird’s Nest Hydrolysate as Affected by Drying Methods. LWT. 2020, 131, 109777. DOI: 10.1016/j.lwt.2020.109777.
- Al-Laith, A. A.; Alkhuzai, J.; Freije, A. Assessment of Antioxidant Activities of Three Wild Medicinal Plants from Bahrain. Arabian J. Chem. 2019, 12, 2365–2371. DOI: 10.1016/j.arabjc.2015.03.004.
- Ma, F.; Liu, D. Sketch of the Edible Bird’s Nest and its Important Bioactivities. Food Res. Int. 2012, 48, 559–567.
- Noor, H. S. M.; Babji, A. S.; Lim, S. J. Nutritional Composition of Different Grades of Edible Bird’s Nest and its Enzymatic Hydrolysis. In AIP Conference Proceedings, AIP Publishing LLC, Selangor, Malaysia, 2018; pp 020088. DOI: 10.1063/1.5028003.
- Saengkrajang, W.; Matan, N.; Matan, N. Nutritional Composition of the Farmed Edible Bird’s Nest (Collocalia Fuciphaga) in Thailand. J. Food Compost. Anal. 2013, 31, 41–45. DOI: 10.1016/j.jfca.2013.05.001.
- Korhonen, H.; Pihlanto, A. Bioactive Peptides: Production and Functionality. Int. Dairy J. 2006, 16, 945–960. DOI: 10.1016/j.idairyj.2005.10.012.
- Shylaja, M.; Seshadri, H. S. Glycoproteins: An Overview. Biochem. Educ. 1989, 17, 170–178. DOI: 10.1016/0307-4412(89)90136-2.
- Goh, D. L. M.; Lau, Y. N.; Chew, F. T.; Shek, L. P. C.; Lee, B. W. Pattern of Food-Induced Anaphylaxis in Children of an Asian Community. Allergy. 1999, 54, 84–86. DOI: 10.1034/j.1398-9995.1999.00925.x.
- Silvestre, M. P.; Hamon, M.; Yvon, M. Analysis of Protein Hydrolyzates. 2. Characterization of Casein Hydrolyzates by a Rapid Peptide Quantification Method. J. Agric. Food Chem. 1994, 42, 2783–2789. DOI: 10.1021/jf00048a025.
- Silvestre, M. P.; Hamon, M.; Yvon, M. Analysis of Protein Hydrolyzates. 1. Use of Poly (2-Hydroxyethylaspartamide)-Silica Column in Size Exclusion Chromatography for the Fractionation of Casein Hydrolyzates. J. Agric. Food Chem. 1994, 42, 2778–2782. DOI: 10.1021/jf00048a024.
- Shahidi, F.; Zhong, Y. Bioactive Peptides. J. AOAC Int. 2008, 91, 914–931. DOI: 10.1093/jaoac/91.4.914.
- See, S. F.; Hoo, L. L., and Babji, A. S. Optimization of Enzymatic Hydrolysis of Salmon (Salmo Salar) Skin by Alcalase. Int. Food Res. J. 2011, 18, 1359–1365.
- Mohr, V. Enzymes Technology in the Meat and Fish Industries. Process Biochem. 1980, 15, 18–21.
- Hoyle, N. T.; Merritt, J. N. Quality of Fish Protein Hydrolysates from Herring (Clupea Harengus). J. Food Sci. 1994, 59, 76–79. DOI: 10.1111/j.1365-2621.1994.tb06901.x.
- Daud, N. A.; Yusop, S. M.; Babji, A. S.; Lim, S. J.; Sarbini, S. R., and Hui Yan, T. Edible Bird’s Best: Physicochemical Properties, Production, and Application of Bioactive Extracts and Glycopeptides. Food Rev. Int. 2019, 1–20. DOI: 10.1080/87559129.2019.1696359.
- Himonides, A. T.; Taylor, A. K.; Morris, A. J. A Study of the Enzymatic Hydrolysis of Fish Frames Using Model Systems. Food Nutr. Sci. 2011, 2, 575–585.
- Mackie, I. M. Fish Protein Hydrolysates. Process Biochem. 1982, 17, 26–28.
- Salwanee, S.; Aida, W. W.; Mamot, S.; Maskat, M. Y.; Ibrahim, S. Effects of Enzyme Concentration, Temperature, pH and Time on the Degree of Hydrolysis of Protein Extract from Viscera of Tuna (Euthynnus Affinis) by Using Alcalase. Sains Malays. 2013, 42, 279–287.
- Zhang, H.; Yu, L.; Yang, Q.; Sun, J.; Bi, J.; Liu, S.; Zhang, C.; Tang, L. Optimization of a Microwave-Coupled Enzymatic Digestion Process to Prepare Peanut Peptides. Molecules. 2012, 17, 5661–5674. DOI: 10.3390/molecules17055661.
- Halimi, N. M.; Kasim, Z. M.; Babji, A. S. Nutritional Composition and Solubility of Edible Bird Nest (Aerodramus Fuchiphagus). In AIP Conference Proceedings, AIP, 2014; pp 476–481.
- Hui Yan, T.; Lim, S. J.; Babji, A. S.; Rawi, M. H.; Sarbini, S. R. Enzymatic Hydrolysis: Sialylated Mucin (Siamuc) Glycoprotein of Edible Swiftlet’s Nest (ESN) and its Molecular Weight Distribution as Bioactive ESN SiaMuc-Glycopeptide Hydrolysate. Int. J. Biol. Macromol. 2021, 175, 422–431. DOI: 10.1016/j.ijbiomac.2021.02.007.
- Green, J. R. The Edible Bird’s-nest, or Nest of the Java Swift (Collocalia Nidifica). J. Physiol. 1885, 6, 40–45. DOI: 10.1113/jphysiol.1885.sp000182.
- Langley, J. N. On the Physiology of the Salivary Secretion. J. Physiol. 1885, 6, 71–92. DOI: 10.1113/jphysiol.1885.sp000184.
- Lagassé, P.; Goldman, L.; Hobson, A., and Norton, S. R. Biochemistry - Glycoprotein. In The Columbia Encyclopedia, 6th ed.; Columbia University Press: New York, 2000. pp 6–6.
- Strous, G. J.; Dekker, J. Mucin-Type Glycoproteins. Crit. Rev. Biochem. Mol. Biol. 1992, 27, 57–92. DOI: 10.3109/10409239209082559.
- Wieruszeski, J. M.; Michalski, J. C.; Montreuil, J.; Strecker, G.; Peter-Katalinic, J.; Egge, H.; Van Halbeek, H.; Mutsaers, J. H.; Vliegenthart, J. F. Structure of the Monosialyl Oligosaccharides Derived from Salivary Gland Mucin Glycoproteins of the Chinese Swiftlet (Genus Collocalia). Characterization of Novel Types of Extended Core Structure, Gal Beta (1—-3)[glcnac Beta (1—-6)] GalNAc Alpha (1—-3) GalNAc (-ol), and of Chain termination,[Gal Alpha (1—-4)] 0-1 [Gal Beta (1—-4)] 2GlcNAc Beta (1—-). J. Biol. Chem. 1987, 262, 6650–6657.
- Brändén, C.-I.; Tooze, J. Introduction to Protein Structure; New York, USA: Taylor & Francis, 2012.
- Creighton, T. E. Proteins: Structures and Molecular Properties; Macmillan Publisher: New York, 1993.
- Lesk, A. M. The Structural Biology of Proteins; Oxford University Press: Oxford, United Kingdom, 2000.
- Voet, D.; Voet, J. G., and Campbell, P. N. Biochemistry. In Trends in Biochemical Sciences, 2nd ed.; Cambridge, Massachusetts, United States: Cell Press: 1995; pp 330.
- Marfo, E. K.; Oke, O. L. Effect of Sodium Chloride, Calcium Chloride and Sodium Hydroxide on Denolix Regia Protein Solubility. Food Chem. 1989, 31, 117–127. DOI: 10.1016/0308-8146(89)90022-8.
- Trimpin, S.; Brizzard, B. Analysis of Insoluble Proteins. Biotechniques. 2009, 46, 409–419. DOI: 10.2144/000113168.
- Arihara, K. Functional Properties of Bioactive Peptides Derived from Meat Proteins. Adv. Technol. Meat Proc. 2006, 245–274. DOI: 10.1201/9781420017311.ch10.
- Arihara, K. Highly Purified Peptides. 2019. https://www.peptidesciences.com/glossary/peptide-solubility/ (accessed Nov 9, 2018).
- Wu, W. U.; Hettiarachchy, N. S.; Qi, M. Hydrophobicity, Solubility, and Emulsifying Properties of Soy Protein Peptides Prepared by Papain Modification and Ultrafiltration. J. Am. Oil Chem.’ Soc. 1998, 75, 845–850. DOI: 10.1007/s11746-998-0235-0.
- Wampler, J. E. The 20 Amino Acids: Hydrophobic, Hydrophilic, Polar and Charged Amino Acids, Structural Bioinformatics, Protein Crystallography, Sequence Analysis & Homology Modeling. 2010. https://proteinstructures.com/Structure/Structure/amino-acids.html (accessed Nov 11, 2018).
- Segrest, J. P. Molecular Weight Determination of Glycoproteins by Polyacrylamide Gel Electrophoresis in Sodium Dodecyl Sulfate. Methods Enzymol. 1972, 28, 54–63.
- Hur, S. J.; Lim, B. O.; Decker, E. A.; McClements, D. J. In Vitro Human Digestion Models for Food Applications. Food Chem. 2011, 125, 1–12. DOI: 10.1016/j.foodchem.2010.08.036.
- Wan, L.; van Huystee, R. B. Rapid Determination of Glycoproteins and Glycopeptides by Periodic Acid Schiff Reagent Dot-Blotting Assay on Nitrocellulose Membrane. J. Agric. Food Chem. 1993, 41, 896–898. DOI: 10.1021/jf00030a011.
- Kruger, N. J. The Bradford Method for Protein Quantitation. In The Protein Protocols Handbook; Totowa, NJ: Humana Press, 2009; pp 15–21. DOI:10.1007/978-1-59745-198-7_4.
- Utomo, B.; Rosyidi, D.; Radiati, L. E.; Puspaningsih, N. N. T.; Proborini, W. D. Protein Characterization of Extracted Water from Three Kinds of Edible Bird Nest Using SDS-PAGE CBB Staining and SDS-PAGE Glycoprotein Staining and LC-MS/MS Analyses. IOSR J. Agric. Vet. Sci. 2014, 7, 33–38. DOI: 10.9790/2380-07933338.
- Babji, A. S.; N. Aliah Daud, Physicochemical Properties of glycan within Swiftlet’s Nest (Aerodramus Fuciphagus) as Potential Prebiotic. Acta Sci. Med. Sci. 2019, 3, 9–13.
- Fountoulakis, M.; Juranville, J.-F.; Manneberg, M. Comparison of the Coomassie Brilliant Blue, Bicinchoninic Acid and Lowry Quantitation Assays, Using Non-Glycosylated and Glycosylated Proteins. J. Biochem. Biophys. Methods. 1992, 24, 265–274. DOI: 10.1016/0165-022X(94)90078-7.
- Perez-Vilar, J.; Hill, R. L. The Structure and Assembly of Secreted Mucins. J. Biol. Chem. 1999, 274, 31751–31754. DOI: 10.1074/jbc.274.45.31751.
- Pace, C. N.; Trevino, S.; Prabhakaran, E.; Scholtz, J. M. Protein Structure, Stability and Solubility in Water and Other Solvents, Phil. Trans. R. Soc. B. Biol. Sci. 2004, 359, 1225–1235. DOI: 10.1098/rstb.2004.1500.
- Tang, C.-H.; Wang, X.-S.; Yang, X.-Q. Enzymatic Hydrolysis of Hemp (Cannabis Sativa L.) Protein Isolate by Various Proteases and Antioxidant Properties of the Resulting Hydrolysates. Food Chem. 2009, 114, 1484–1490. DOI: 10.1016/j.foodchem.2008.11.049.
- Tanford, C. Contribution of Hydrophobic Interactions to the Stability of the Globular Conformation of Proteins. J. Am. Oil Chem.’ Soc. 1962, 84, 4240–4247. DOI: 10.1021/ja00881a009.
- Wu, Y.; Chen, Y.; Wang, B.; Bai, L.; Ge, Y.; Yuan, F. Application of SYBRgreen PCR and 2DGE Methods to Authenticate Edible Bird’s Nest Food. Food Res. Int. 2010, 43, 2020–2026.
- Chua, Y. G.; Chan, S. H.; Bloodworth, B. C.; Li, S. F. Y.; Leong, L. P. Identification of Edible Bird’s Nest with Amino Acid and Monosaccharide Analysis. J. Agric. Food Chem. 2015, 63, 279–289. DOI: 10.1021/jf503157n.
- Chua, Y. G.; Chan, S. H.; Bloodworth, B. C.; Li, S. F. Y.; Leong, L. P. Identification of Edible Bird’s Nest with Amino Acid and Monosaccharide Analysis. J. Agric. Food Chem. 2014, 63, 279–289. DOI: 10.1021/jf503157n.
- Foye, O. T.; Uni, Z.; Ferket, P. R. Effect of in Ovo Feeding Egg White Protein, β-hydroxy-β-methylbutyrate, and Carbohydrates on Glycogen Status and Neonatal Growth of Turkeys. Poult. Sci. 2006, 85, 1185–1192. DOI: 10.1093/ps/85.7.1185.
- Ng, S. R.; Mohd Noor, H. S.; Ramachandran, R.; Hui Yan, T.; Ch′ng, S.-E.; Chang, L. S.; Babji, A. S.; Lim, S. J. Recovery of Glycopeptides by Enzymatic Hydrolysis of Edible Bird’s Nest: The Physicochemical Characteristics and Protein Profile. J. Food Measure. Char. 2020, 14, 2635–2645. DOI: 10.1007/s11694-020-00510-4.
- Bujang, A.; N.A. Taib. Changes on Amino Acids Content in Soybean, Garbanzo Bean and Groundnut during Pre-Treatments and Tempe Making. Sains Malays. 2014, 43, 551–557.
- Ismail, M.; Mariod, A., and Pin, S. S. Effects of Preparation Methods on Protein and Amino Acid Contents of Various Eggs Available in Malaysian Local Markets. Acta Sci. Pol. Technol. Aliment. 2013, 12, 1, 21–31.
- Jorfi, R.; Mustafa, S.; Man, Y. B. C.; Hashim, D. B. M.; Sazili, A. Q.; Farjam, A. S.; Nateghi, L.; Kashiani, P. Differentiation of Pork from Beef, Chicken, Mutton and Chevon according to their Primary Amino Acids Content for Halal Authentication. Afr. J. Biotechnol. 2012, 11, 8160–8166.
- Ghassem, M.; Arihara, K.; Mohammadi, S.; Sani, N. A.; Babji, A. S. Identification of Two Novel Antioxidant Peptides from Edible Bird’s Nest (Aerodramus Fuciphagus) Protein Hydrolysates. Food Funct. 2017, 8, 2046–2052. DOI: 10.1039/C6FO01615D.
- Klein, C. J. Nutrient Requirements for Preterm Infant Formulas. J. Nutr. 2002, 132, 1395S–1577S. DOI: 10.1093/jn/132.6.1395S.
- Hascoët, J.-M.; Hubert, C.; Rochat, F.; Legagneur, H.; Gaga, S.; Emady-Azar, S.; Steenhout, P. G. Effect of Formula Composition on the Development of Infant Gut Microbiota. J. Pediatr. Gastroenterol. Nutr. 2011, 52, 756–762. DOI: 10.1097/MPG.0b013e3182105850.
- Babji, A. S.; Nurfatin, M. H.; Etty Syarmila, I. K.; Farahniza, Z.; Masitah, M. Bioactive Swiftlet Nest Capsule, Natural Antihypertensive & Antioxidant Relief Capsules, Safe Food Expo MAFSA; 13th–14th Sept, 2014.
- Aluko, R. E. Amino Acids, Peptides, and Proteins as Antioxidants for Food Preservation. In Handbook of Antioxidants for Food Preservation; Sawston, United Kingdom: Elsevier, 2015; pp 105–140. doi:10.1016/B978-1-78242-089-7.00005-1.
- Gülçin, I. Comparison of in Vitro Antioxidant and Antiradical Activities of L-Tyrosine and L-Dopa. Amino Acids. 2007, 32, 431. DOI: 10.1007/s00726-006-0379-x.
- Marcuse, R. The Effect of Some Amino Acids on the Oxidation of Linoleic Acid and its Methyl Ester. J. Am. Oil Chem.’ Soc. 1962, 39, 97–103. DOI: 10.1007/BF02631680.
- Vasdev, S.; Stuckless, J. Antihypertensive Effects of Dietary Protein and its Mechanism. Int. J. Angiol. 2010, 19, e7–e20. DOI: 10.1055/s-0031-1278362.
- Doi, N.; Kakukawa, K.; Oishi, Y.; Yanagawa, H. High Solubility of Random-Sequence Proteins Consisting of Five Kinds of Primitive Amino Acids, Protein Eng. Des. Selection. 2005, 18, 279–284.
- Longo, L. M.; Blaber, M. Protein Design at the Interface of the Pre-Biotic and Biotic Worlds. Arch. Biochem. Biophys. 2012, 526, 16–21. DOI: 10.1016/j.abb.2012.06.009.
- Longo, L. M.; Lee, J.; Blaber, M. Simplified Protein Design Biased for Prebiotic Amino Acids Yields a Foldable, Halophilic Protein, Arch. Biochem. Biophys. 2013, 110, 2135–2139.
- McDonald, G. D.; Storrie-Lombardi, M. C. Biochemical Constraints in a Protobiotic Earth Devoid of Basic Amino Acids: The “BAA (-) World. Astrobiol. 2010, 10, 989–1000. DOI: 10.1089/ast.2010.0484.
- Ortiz, S. E. M.; Wagner, J. R. Hydrolysates of Native and Modified Soy Protein Isolates: Structural Characteristics, Solubility and Foaming Properties. Food Res. Int. 2002, 35, 511–518.
- Cole, C. R.; Smith, C. A. Glycoprotein Biochemistry (Structure and Function)—a Vehicle for Teaching Many Aspects of Biochemistry and Molecular Biology. Biochem. Mol. Biol. Educ. 1989, 17, 179–189.
- Nielsen, S. S. Phenol-Sulfuric Acid Method for Total Carbohydrates. In Food Analysis Laboratory Manual; Boston, Massachusetts: Springer, 2010; pp 47–53.
- Rao, P.; Pattabiraman, T. N. Reevaluation of the Phenol-Sulfuric Acid Reaction for the Estimation of Hexoses and Pentoses. Anal. Biochem. 1989, 181, 18–22. DOI: 10.1016/0003-2697(89)90387-4.
- Kathan, R. H.; Weeks, D. I. Structure Studies of Collocalia Mucoid: I. Carbohydrate and Amino Acid Composition. Arch. Biochem. Biophys. 1969, 134, 572–576. DOI: 10.1016/0003-9861(69)90319-1.
- Yida, Z.; Imam, M. U.; Ismail, M.; Hou, Z.; Abdullah, M. A.; Ideris, A.; Ismail, N. Edible Bird’s Nest Attenuates High Fat Diet-Induced Oxidative Stress and Inflammation via Regulation of Hepatic Antioxidant and Inflammatory Genes. BMC Complementary Altern. Med. 2015, 15, 310. DOI: 10.1186/s12906-015-0843-9.
- Pratt, C. W., and Cornely, K. Carbohydrates. In: Essential Biochemistry; Yee Jennifer; New Jersey: Wiley Hoboken, 2004; pp 626.
- Zhao, Y.; Kent, S. B.; Chait, B. T. Rapid, Sensitive Structure Analysis of Oligosaccharides. Proc Natl. Acad Sci. 1997, 94 1629–1633.
- Yu-Qin, Y.; Liang, X.; Hua, W.; Hui-Xing, Z.; Xin-Fang, Z.; Bu-Sen, L. Determination of Edible Bird’s Nest and its Products by Gas Chromatography. J. Chromatographic Sci. 2000, 38, 27–32. DOI: 10.1093/chromsci/38.1.27.
- Melander, C.; Andersson, E.; Axelsson, S.; Gorton, L. Determination of Reducing Ends with Flow Injection Analysis with Amperometric Detection: Application to Enzyme-Hydrolysed Methyl Cellulose. Anal. Bioanal. Chem. 2007, 387, 2585–2593. DOI: 10.1007/s00216-006-1107-9.
- Negrulescu, A.; Patrulea, V.; Mincea, M. M.; Ionascu, C.; Vlad-Oros, B. A.; Ostafe, V. Adapting the Reducing Sugars Method with Dinitrosalicylic Acid to Microtiter Plates and Microwave Heating. J. Braz. Chem. Soc. 2012, 23, 2176–2182. DOI: 10.1590/S0103-50532013005000003.
- Nelson, D. L.; Lehninger, A. L., and Cox, M. M. Carbohydrate and Glycobiology. In Principles of Biochemistry 4th ed.; Macmillan Publisher: New York, 2008; 239–267.
- Berg, J. M, Tymoczko, J. L, Stryer, L. Protein Turnover and Amino Acids Catabolism. In Biochemistry 5th ed.; New York: W.H. Freeman, 2008.
- D’mello, J. P. F. Amino Acids as Multifunctional Molecules. Amino Acids in Animal Nutrition. 2003, 2, 1–14.
- Tillery, B. W.; Enger, E. D.; F., c Ross, Integrated Science. McGraw-Hill: New York, 2004.
- Phschool, C. The Carboxyl Group: Acids, Pearson - The Biology Place. 2017. http://www.phschool.com/science/biology_place/biocoach/biokit/carboxyl.html (accessed June 3, 2018).
- Teixeira, R. S. S.; Da Silvada Silva, A. S.; Ferreira-Leitão, V. S.; Da Silva Bon, E. P. Amino Acids Interference on the Quantification of Reducing Sugars by the 3, 5-Dinitrosalicylic Acid Assay Mislead Carbohydrase Activity Measurements. Carbohydr. Res. 2012, 363, 33–37.
- Adamson, N. J.; Reynolds, E. C. Characterization of Casein Phosphopeptides Prepared Using Alcalase: Determination of Enzyme Specificity. Enzyme Microbial. Technol. 1996, 19, 202–207. DOI: 10.1016/0141-0229(95)00232-4.
- Mandal, C. Sialic Acid Binding Lectins. Experientia. 1990, 46, 433–441. DOI: 10.1007/BF01954221.
- Wang, B.; Brand-Miller, J. The Role and Potential of Sialic Acid in Human Nutrition. Eur. J. Clin. Nutr. 2003, 57, 1351–1369. DOI: 10.1038/sj.ejcn.1601704.
- Svennerholm, L. Quantitative Estimation of Sialic Acids: II. A Colorimetric Resorcinol-Hydrochloric Acid Method. Biochim. Biophys. Acta. 1957, 24, 604–611. DOI: 10.1016/0006-3002(57)90254-8.
- Ling, J. W. A.; Chang, L. S.; Babji, A. S.; Lim, S. J. Recovery of Value-Added Glycopeptides from Edible Bird’s Nest (EBN) Co-Products: Enzymatic Hydrolysis, Physicochemical Characteristics and Bioactivity. J. Sci. Food Agric. 2020, 100, 4714–4722. DOI: 10.1002/jsfa.10530.