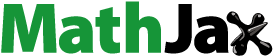
ABSTRACT
The objective of this study was to prepare high-elasticity and high-strength ovalbumin (OVA) gels by comparing the gelling behaviors under the four treatments (alkali-induced, AI; heat-induced, HI; alkali-heat-induced, AHI; and alkali-heat-induced in sequence, AHIS). We measured the secondary structure and investigated the changes in physicochemical properties, intermolecular forces, and water holding capacity (WHC) of the four gels during aging. The AHIS treatment improved the hardness and springiness of the gel; after 1 h of gel formation, the maximum value reached 754.22 ± 46.92 g and 0.96 ± 0.02, respectively. The WHC of the gels also presented a high level, with a maximum value of 88.40 ± 1.20% at 1 h. Although the HI gel had a high hardness value (957.32 ± 51.96 g), it exhibited the lowest elasticity value (0.68 ± 0.03) in 5 h. Meanwhile, the AI gel had low hardness (661.48 ± 32.43 g) and springiness (0.77 ± 0.03) in 5 h. A compact and regular microstructure was formed when the OVA solution was treated with AHIS, meanwhile, the free sulfhydryl (SH) and disulfide bonds (SS) and WHC of the AHIS gel did not change significantly (p > .05), which demonstrated that the gel had formed a stable gel system. Raman spectroscopy showed that AHIS treatment was beneficial to enhance the degree of cross-linking between proteins, the secondary structure changed considerably, the α-helix structure reduced, and the β-structure (β-sheet and β-turn) and random coil increased. Thus, this study provides a new approach to developing high-protein alkaline gel food.
INTRODUCTION
Preserved eggs are traditional egg products in China, having many distinct characteristics, including unique flavor, dark green yolk, and dark brown or transparent egg white (EW).[Citation1,Citation2] It is usually preserved in an alkaline solution or by marinating in alkaline mud with some additions (such as alkaline solution, salt, black tea, and metal ions) for 4–6 weeks at room temperature.[Citation3–5] The sodium hydroxide component is commonly used in the production of preserved eggs to result in the gelation of EW proteins and physicochemical changes.[Citation4] Nonetheless, the continuous or excess penetration of alkali can cause the congealed EW gel to liquefy, and on the other hand, as food, excessive alkali can burn the human gastrointestinal tract.[Citation6,Citation7] Some studies have found that the addition of metal compounds can form insoluble sulfides to block the pores of the eggshell, which preventing excessive alkali from causing damage[Citation8;] however, the metal compounds will inevitably lead consumers to worry about food safety issues. Hence, nowadays, non-heavy metal methods have been widely adopted to marinate preserved eggs to adjust the penetration of alkali.[Citation9–11] It is still worthwhile to continue development and in-depth research in heavy metal-free curing methods, which are important for the safety and processability of gel food.
The gel can be obtained when proteins undergo physical or chemical processes, such as pressurization, heating, and acid, alkali, or enzymatic treatments.[Citation12–16] However, the single induction treatment has some shortcomings. For example, alkali treatment causes the hardness and water holding capacity of OVA gel to gradually decrease after reaching a certain level,[Citation17,Citation18] and this trend is also reflected in the behavior of EW proteins induced by alkali.[Citation19] It is known that a typical heat-treated gel is a nontransparent white gel, like boiled egg white, which has poor elasticity and does not have the same transparency as the preserved eggs.[Citation20] Currently, most studies have focused on the mechanism of a single induction treatment on EW or whole eggs, and only a few researchers have combined two or more induction methods to investigate the gelation properties of proteins. Li et al. figured that a new process using the alkali/heat-induced gel method could be an important supplement to the traditional osmotic curing method.[Citation21] Therefore, studying the effect of alkali-heat treatment on gel properties has far-reaching significance.
OVA is the main protein of EW, accounting for 54–66%, and composed of 385 amino acids, among which half are hydrophobic and a third is charged.[Citation20,Citation22–24] As OVA is a major protein in EW and plays a significant role in the functionality of EW, especially its gelling property,[Citation25] many researchers have used it to investigate the effects of changes in the environmental variables on EW gels.[Citation18–20,Citation26] Previous research on the gel of preserved eggs and model of gel formation under alkali conditions showed that OVA plays a dominant role in gel formation under strong alkali conditions.[Citation22] Therefore, we used OVA as a model system to investigate the effect of alkali-heat treatment on the quality and formation of gels. Four treatments were used to induce OVA formation to explore the changes in physicochemical properties, textural characteristics, and water holding capacity of OVA gels at different aging periods, and the secondary structure, intermolecular forces, and thermal characteristics of the four gels after aging were measured. On the one hand, it can provide theoretical guidance for developing egg products. On the other hand, it can also contribute to new ideas for further enriching and improving high-protein gel foods.
MATERIALS AND METHODS
Materials
OVA powder was purchased from Beijing Huamai Biotechnology Co., Ltd. (Beijing, China). Sodium hydroxide and β-mercaptoethanol were purchased from Shanghai Macklin Biochemical Co., Ltd. (Shanghai, China). All other chemicals used in this study were of analytical grade and obtained from Chengdu Kelong Chemical Reagent Co., Ltd. (Sichuan, China).
Preparation of OVA gels
A 20 g of OVA solution (9%, w/w) was mixed with 2 mol/L alkali solution in a 50 mL beaker to get a final concentration of 0.2, 0.3, 0.4, 0.5, and 0.6% NaOH (w/w) and then heated at 80°C for 10 min after 3 h. A 20 g of OVA solution (9%, w/w) was mixed with 0.4% NaOH (w/w) and allowed to stand for 1, 2, 3, 4, and 5 h respectively, and then heated at 80°C for 10 min. A 20 g of OVA solution (9%, w/w) was mixed with 0.4% NaOH (w/w) and allowed to stand for 3 h and then heated at 50, 60, 70, 80, and 90°C, respectively, for 10 min. A 20 g of OVA solution (9%, w/w) was mixed with a final concentration of 0.4% NaOH (w/w) and allowed to stand for 3 h, followed by heating at 80°C for 5, 10, 15, and 20 min, respectively. After heating, the samples were immediately cooled in ice water and kept at 4°C overnight. Subsequently, the most suitable alkali concentration, alkali treatment time, heating temperature, and heating time in the above method were chosen to prepare the subsequent gel.
The alkali-induced (AI) OVA gel was prepared as follows: 20 g of OVA solution (9%, w/w) was mixed with 0.5% NaOH (w/w) in a 50 mL beaker at a constant temperature (25 ± 1°C) for examination. Heat-induced (HI) OVA gel was prepared as follows: 20 g of OVA solution (9%, w/w) was heated in a water bath at 80°C for 10 min, cooled in ice water, and equilibrated to room temperature (25 ± 1°C). Alkali-heat-induced (AHI) OVA gel was prepared as follows: 20 g of OVA solution (9%, w/w) was mixed with 0.5% NaOH solution in a 50 mL beaker, heated in a water bath at 80°C for 10 min, cooled in ice water, and then equilibrated to room temperature (25 ± 1°C). Alkali-heat-induced in sequence (AHIS) OVA gel was prepared as follows: approximately 20 g of OVA solution (9%, w/w) was mixed with 0.5% NaOH solution in a 50 mL beaker at constant temperature (25 ± 1°C), allowed to stand for 4 h, heated in a water bath at 80°C for 10 min, cooled in ice water, and then equilibrated to room temperature (25 ± 1°C). The above gels were used for further analysis.
Surface hydrophobicity of OVA gels
The OVA gel surface hydrophobicity was determined according to the method of Deng with some modifications.[Citation27] The sample (3 g) was added to 60 mL of distilled water. After homogenization, 2 mL of the supernatant was drawn, followed by addition of 200 μL of 1 mg/mL bromophenol blue (BPB) (in distilled water) and mixed well, stirred at room temperature for 10 min, and then centrifuged at 2000 × g for 15 min. The supernatant was diluted 10 times, 2 mL of phosphate buffer (20 mmol/L, addition of 200 μL of BPB, pH 6.0) was used as a control, and the absorbance (A) of the solution was measured at 595 nm. The hydrophobicity of the protein surface was expressed as the amount of bound BPB according to the following formula (2):
Free sulfhydryl (SH) and disulfide bonds (SS) contents of OVA gels
The OVA gel (5 g) was homogenized in 60 mL Tris-glycine buffer for 2 minutes before being allowed to stand for a while. For SH, 2.9 mL of 0.5% SDS in Tris-Gly was mixed with 0.1 mL of diluted OVA gel and 0.02 mL of Ellman’s reagent was added to develop color. A UV-visible spectrophotometer (UV-1800, Shanghai, China) was used to measure absorbance (A) at 412 nm. For SS, 0.2 mL diluted sample, 1 mL 10 M urea, and 0.02 mL 2-mercaptoethanol were combined and let to stand for 1 hour. After precipitating the protein with 10 mL of trichloroacetic acid (TCA), incubating it at 40°C for 15 minutes, and centrifuging it at 5000 × g for 10 minutes, the precipitate was washed twice with 5 mL of 12% TCA, dissolved in 3 mL of 0.5% SDS, and 1 mL was diluted to 10 mL with the same solvent. Ellman’s reagent (0.05 mL) was used to create the color. A UV-visible spectrophotometer was used to detect absorbance (A) at 412 nm.[Citation28] Equation (3) and (4) were used to compute the contents of SH group and SS bonds:
where A412 is the absorbance at 412 nm, C is the sample concentration in mg solids/mL, D is the dilution factor, 30.2 for SH and 150 for total SH (SH + reduced SS) in egg white respectively, and 73.53 is derived from 106/(1.36 × 104), 1.36 × 104 is the molar absorptivity and 106 is for conversions from the molar basis to the µM/mL basis and from mg solids to g solids. N2 = number of SH groups after reduction, N1 = number of sulfhydryl groups before reduction.
Chemical interactions of OVA gels
The selective protein solubility of the OVA gel was determined using Deng with minor changes.[Citation29] 0.6 M sodium chloride (S1), 0.6 M sodium chloride + 1.5 M urea (S2), 0.6 M sodium chloride + 8 M urea (S3), and 0.6 M sodium chloride + 8 M urea + 0.5 M β-mercaptoethanol (S4) were used to solubilize the OVA gel. Approximately 2 g of the gel was homogenized (High-speed dispersion homogenizer, FJ200-SH, Shanghai Huxi Industrial Co., Ltd., Shanghai, China) with 10 mL of the above solution at 4000 rpm for 3 min at room temperature (25 ± 1°C), followed by refrigeration at 4°C for 1 h and then centrifuged (Kait, TG18G, Jiangsu, China) at 8000 rpm for 10 min. The supernatant was aspirated and its protein concentration was determined by the Bradford method. The absorbance was measured at 595 nm using UV spectrophotometer (Shimadzu UV-VIS Spectrophotometer, UV-1900i, Kyoto, Japan)
Water holding capacity (WHC) of OVA gels
The WHC of the OVA gel was caculated using Wu’s approach with a few tweaks.[Citation30] The gel sample was sliced into 30 × 10 × 5 mm thick slices and the mass of the sample was defined as m1. The OVA gel was wrapped in a long strip of filter paper and crushed with a force of 1 kg for 2 minutes, after which the filter paper strip and the gel were carefully separated, and the mass of the squeezed sample was defined as m2. The following equation (5) was used to calculate the WHC:
Instrumental Texture Profile Analysis (TPA)
The texture of the OVA gel sample was evaluated with a probe (P/0.5) using a TA-XT2i texture analyzer (Stable Micro Systems, Surrey, England). The OVA gel sample was cut into 1 cm3 block and placed on the texture analyzer platform manually. Pretest, test and after test speeds were set to 1 mm/s, a trigger force was set to 5 g, and a compression ratio was set to 50%.[Citation6] The hardness and springiness values were obtained using the Micro Stable Software (Stable Micro Systems, Surrey, England).
Raman spectroscopic measurement
The Raman spectra of AI, HI, AHI, and AHIS OVA gels were measured on the surface of the glass using a laser confocal Raman spectrometer (inVia, Renishaw, England). The slides wrapped in foil were placed under a microscope with a 50 × objective to collect the Raman scattering from the samples. The instrument was equipped with a 180 nm laser (Invictus, Kaiser Optical Systems Inc., Michigan, USA). The laser was focused on each sample and the laser power on the sample was 10 mW. The spectra were acquired using an average spectrum of 40 scans, each with an exposure time of 10s, and were stored as Raman shifts in the range of 100–3800 cm−1. The results were normalized, and the baseline was corrected using Origin. 9.0.[Citation16]
Scanning electron microscopy (SEM) imaging of OVA gels
The gel sample was cut into small particles of about 5 × 5 × 5 mm, placed in a weighing bottle and dried in a constant temperature blast drying oven (GZX-9146MBE, Shanghai Boxun Medical Biological Instrument Corp, Shanghai, China) at 60°C to make its moisture close to zero. After spraying with gold using a sputtering ion instrument, it was scanned and analyzed by scanning electron microscope (Tungsten hairpin filament lamp scanning electron microscope, TESCAN VEGA 3 SBU, TESCAN China, Ltd., Shanghai).
Statistical analyses
The statistical significant tests were performed using analysis of variance (ANOVA) at 95% confidence level. Significant differences among mean values were determined by Tukey’s test.
RESULTS
Changes in hardness and WHC of OVA gels at different conditions
WHC is one of the methods used to determine the internal structure of protein gels.[Citation31] A lower WHC value is related to a coarser microstructure, while aggregate size and density are closely linked to microstructure and gel stiffness and hence to water holding ability of the gel.[Citation32] As shown in , the hardness and WHC of the gels exhibited an increasing trend and then decreased with increasing NaOH concentration, and both reached the maximum at 0.5% alkali concentration, showing values of 715.31 ± 36.44 g and 92.36 ± 0.46%, respectively. This is due to the fact that high concentrations of alkali can damage the internal structure of protein molecules, resulting in excessive internal gaps and a decrease in WHC and hardness. Previous studies have found that alkali treatment could enhance or weaken the strength of the gel, depending on the duration of the alkali treatment. As shown in , the hardness and WHC of the gels showed a gradually increasing trend, which then decreased with an increase in the alkali treatment time. This trend was consistent with the alkali-induced EW model of Chen et al.[Citation22] After 4 h of alkali treatment, the hardness and WHC of gels reached maximum values of 788.52 ± 69.79 g and 96.57 ± 1.53%, respectively. At low temperatures, the OVA denaturation had a lower degree of solidification and a looser structure. When the temperature exceeded 80°C, the gel strength and WHC decreased significantly (p < .05). In addition, the 3D gel network became unstable due to high temperature, resulting in a decrease in WHC and hardness when the temperature exceeded 80°C. With increasing heating temperatures, the degree of involvement of new disulfide bonds correlates with the degree of protein unfolding/aggregation, enhancing the obtained gel strength.[Citation33] As shown in , with an increase in heating temperature, the hardness and WHC of the gel displayed an increasing and then decreasing trend, and reached the maximum at a temperature of 80°C, showing values of 921.64 ± 97.97 g and 92.14 ± 0.42%, respectively. Protein denaturation is a slow process; when it reaches the required denaturation time, the protein molecules are fully developed, and the gel exhibits high hardness. With continuation in the extension of the heating time, high temperatures destroy the three-dimensional network that has been formed and damaged the gel system, causing a decrease in its ability to combine with more water and thus lower the qualitative properties of the gel system. After about 20 min of heating, the gel system completely collapses, resulting in liquefaction of the gel. As shown in , with an increase in heating time, the hardness and WHC of the gel showed a slowly increasing and then decreasing trend, reaching the maximum at 10 min of heating with values of 758.32 ± 43.84 g and 88.47 ± 0.32%, respectively. Therefore, we chose to prepare the gels for the subsequent experiments at an alkali concentration of 0.5%, an alkali treatment time of 4 h, a heating temperature of 80°C, and a heating time of 10 min.
Changes in surface hydrophobicity of OVA gels
OVA consists of 385 amino acids residues, of which about half were hydrophobic and hidden in protein molecules.[Citation34] The level of the unfolding of proteins can be determined by surface hydrophobicity. The dissociation of protein subunits or the expansion of peptide chains exposes hydrophobic groups in protein molecules, which leads to the destruction of the secondary and tertiary structures of the proteins. As shown in , with an increase in the aging time, the surface hydrophobicity of AI and AHI gels showed a significant increase (p < .05), indicating that the unfolding of the gel is not yet finished and the hydrophobic groups are still exposed under alkali environment. During aggregate formation, the continuous interactions between and within protein molecules affect the covalent and non-covalent bonds used to stabilize the tertiary structure and intermolecular aggregation.[Citation33] With an increase in the aging time, the surface hydrophobicity of OVA gels treated with AHIS decreased gradually. This could be because heating the OVA solution was heated after 4 h of alkali treatment might have completely denatured the proteins in the system and fully degraded and recombined the OVA, which resulted in less exposure of hydrophobic amino acids to the outside of protein aggregates. On the one hand, the alkali treatment denatured the protein, and exposed the hydrophobic groups in the protein,[Citation17] which could be seen from the trend of the AI gel. On the other hand, heating promoted the denaturation of the protein, further exposing the hydrophobic groups that are reburied into the protein molecule by aggregation as the heating proceeds.[Citation35] The evidence suggests that the AHIS treatment can enhance the aggregation of the protein, allowing the hydrophobic groups to be further buried in the protein molecules, contributing to the strength of the OVA gel.
Changes in free SH group and SS bond content of OVA gels
A previous study reported that each OVA molecule contains four free SH groups buried in the protein core.[Citation36] showed the changes in free SH contents of OVA gels during different treatments. The free SH content during the four treatments showed a significant downward trend (p < .05) in varying degrees initially, which is consistent with the results of Guo et al.,[Citation37] and the SH content during the four treatments reached roughly the same level after 5 h of aging. In the initial stage, the SH contents of the AI gels were greater than that of the HI gel. This is because, under alkaline conditions, the SH oxidation and SH/SS transformation were rapidly completed, resulting in an accelerated decrease in SH content.[Citation15] After aging for 1 h, the AHIS gel had the lowest SH content, reaching a value of 29.41 ± 0.02 μmol/g protein, and the high temperature made the protein to gradually aggregate, causing hydrophobic shrinkage and thus, the contents of SH groups reduced. This implied that the AHIS treatment made the gel denature more completely and formed a more stable gel than other treatments.
As shown in , the SS bond content of the AI gel was lower than the other three groups that have undergone heat treatment, indicating that heat treatment can increase the disulfide bond content in the gel, which helps to enhance the cross-linking between proteins to support the gel structure.[Citation38] In extreme environments, the majority of exposed SH groups can form SS bonds through SH oxidation and SH-SS exchange.[Citation14] Initially, this change in the gel under the combined alkali-heat treatment was greater than that of the alkali or heat treatment. AHIS gel had a stable and abundant SS bond content, which indicated that the cross-links between proteins are relatively tight. With increase in time, the content of SS bonds in AHIS gel showed a gradual increase and then a slow decline, and this change was not significant (p > .05). The reason for this phenomenon might be that the simultaneous action of alkali and heat accelerated the conversion of SH. Furthermore, the prolonged exposure to strong alkaline solutions severely damaged and degraded the OVA protein, resulting in the SS bond to not only be destroyed in the new network but also the original interior of the molecule.[Citation22,Citation39] The aggregation of protein during the gelation process also affects the gelling properties and changes in β-turn and β-coils. The β-turn and β-coil structures are the basis of gel formation, and the increase in the content of both is beneficial to the formation of good gel structure and increases the hardness of the gel.[Citation40]
Intermolecular forces of OVA gels
The gel formation is inevitably driven and maintained by certain interaction forces between molecules. As shown in , different solvents can destroy the various interaction forces between protein molecules.[Citation18] For example, 0.6 mol/L NaCl can break ionic bonds, 1.5 mol/L urea can break hydrogen bonds, 8 mol/L urea can break hydrogen bonds and hydrophobic interactions, and 0.5 mol/L β-mercaptoethanol can break disulfide bond.[Citation41] By superimposing and combining different solvents, solutions capable of measuring different molecular forces can be obtained: S1 (ionic bond), S2 (hydrogen bond), S3(hydrophobic interaction), and S4 (disulfide bond). High temperature can expose hidden SH and hydrophobic groups inside protein molecules, thus enhancing the interaction between molecules and providing a driving force for gel formation,[Citation33] which reasonably explains the high content of hydrophobic interactions in HI gels. Hydrophobic interaction, ionic bonds and disulfide bonds played a significant role in the HI gel system, which accounted for 43.45 ± 0.15%, 30.96 ± 0.16% and 22.77 ± 1.22%, respectively. The ratio of intermolecular forces between AI and AHI gels is very close, and it is presumed that the mechanism of AI and AHI on the formation of OVA gel is similar, and the effect of alkali on gels is greater than that of heat. The ionic bonds content of all three alkali-treated gels exceeded 60%, which was similar to the gelation results from the alkali-induced EW model,[Citation22] while the highest ionic bond content of 72.93 ± 0.54% was achieved in the AHIS gels. Numerous ionic bond arise from water-ion bonds between the ionized groups of protein and water molecules, as well as the electrostatic repulsion forces arising from the net negative surface charge between protein molecules.[Citation4] As the alkali processing continued, the stabilized gel became capable of absorbing water and the retained water made it easy to form hydrogen bonds in OVA gel.[Citation39] Therefore, the content of hydrogen bond in all the gels treated with alkali was high, while the HI gel without alkali treatment had the lowest content.
Changes in WHC of OVA gels
Changes in WHC of OVA gels induced by different treatments were determined during aging. WHC is an important factor for objectively evaluating the gel matrix to immobilize water.[Citation42] It can represent the binding states between water and protein molecules in the sample and the strength of the gel network structure to a certain extent.[Citation17] It can be seen from that the WHC of the HI OVA gel was significantly lower than the other three groups. The hardness of the HI OVA gel was also significantly lower than the other three groups (p < .05), which also confirmed the conclusion that the WHC of a gel could be determined by both gel microstructure and gel stiffness.[Citation43,Citation44] AI OVA gel showed a significant increase in WHC. It could be presumed that with continuous penetration of strong alkalis, the OVA protein underwent physical and chemical changes and gelation, continuously forming a three-dimensional gel network, and this structure locked more moisture. The WHC of both AHI and AHIS OVA gels showed a significant decline (p < .05). This could be because, under the combined action of alkali and heat, the protein was completely denatured in a relatively short time. With an increase in the aging time, under the continuous action of alkali, the gel structure gradually disintegrated and was fixed in the gel system, causing the release of free water and the gel WHC to decrease. The WHC of the AHIS gel was significantly higher than that of AHI, indicating that the AHIS OVA gel had better gel strength, which could be confirmed by the hardness and springiness indicators in . Huang and Ai et al. demonstrated that the gels obtained by alkali-heat treatment have an advantage over single alkali-treated gels in terms of hardness.[Citation20,Citation45]
Changes in textural properties of OVA gels
Hardness is one of the most important quality characteristics of gel products.[Citation21] As shown in , the hardness of the HI OVA gel showed a decreasing trend initially (p < .05), and then maintained a stable trend; this could be because, with the effects of high temperature, fine network structure might be destroyed and formed holes.[Citation35] The hardness value of the HI gel was higher than the other three groups; however, the thermal gel was white, and not the expected brown elastic gel. Zhao et al. demonstrated that the OVA gel induced by strong alkali increased and then decreased in gel strength which is consistent with the model of alkali-induced EW gel,[Citation18] and the hardness measured was the highest after 30 min. Due to the low concentration of NaOH used in the experiment and the heat treatment enhancing the strength of the gel, the OVA gel induced by the combination of alkali and heat was formed earlier and reached an equilibrium state. Therefore, the hardness of the AHI and AHIS gels remained relatively stable during the aging process (p > .05). As the AHIS gel first underwent 4 h alkali treatment and then 10 min heat treatment, the hardness value reached 754.22 ± 46.92 g after aging for 1 h, which was higher than the gel hardness showing a value of 658.00 ± 32.43 g after alkali treatment for 4 h. This indicates that heat treatment is of great significance for improving the strength of the gel. In contrast, the AHIS gel also showed higher hardness, with similar elasticity and texture as the preserved egg white.
Springiness determines the destruction of the gel structure during initial compression.[Citation22] High values of springiness indicate the ability of the gel to maintain an intact network structure.[Citation46] As shown in , the springiness of the AHI gel showed a significant decline in the first 2 h (p < .05), after which this parameter remained almost unchanged. In addition, the other three groups of springiness indicators showed a significant decline in varying degrees (p < .05). In particular, the springiness of the HI OVA gel showed the largest decline, with the springiness value dropping from 0.90 ± 0.06 to 0.68 ± 0.03, which may be caused by high temperature, destroying the gel network structure. Within 2–5 h, the springiness of the AHIS gel was higher than the other three groups, indicating that compared with other treatment methods, the AHIS treatment could be better at maintaining an intact network structure. From the comprehensive view of , the AHIS gel exhibited excellent hardness and springiness.
Raman spectroscopy of OVA gels
The effect of different treatments on the Raman bands (1200–1800 cm−1) of OVA gels is shown in . The amide (peptide) bond of proteins has several different vibrational modes, of which the amide I and III bands are the most useful for studying the secondary structure. The amide III bands mainly include C-N extension and N-H stretching vibration in the small peptide group, usually located near 1200–1350 cm−1. The wavenumber depends on the geometry of the protein backbone.[Citation33] The specific frequency represents different protein secondary structures, particularly in the amide I region (1600–1700 cm−1), which are due to the contributions of C = O stretching vibration of the amide group, as well as N-H bending and C-N stretching vibration in the plane. Theoretically, the main conformational information of the amide I band is the same as that of the amide III band. However, the amide I band is sensitive to changes in hydrogen bonding, and hence, it can reflect changes in the protein secondary structure well. The information provided by the 1650–1660 cm−1, 1670–1680 cm−1, 1660–1670 cm−1 and 1680–1699 cm−1 reflects the changes in α-helix, β-sheet, random coils and β-turn contents, respectively.[Citation47] As shown in , the quantitative analysis of HI OVA gel showed 15.58% α-helix, 53.93% β-sheet, 16.81% β-turn and 13.68% random coil contents. This is consistent with previous Raman studies on heat-treated OVA gels (16% α-helix, 51% β-sheet, 20% β-turn and 13% random coil).[Citation48] Compared to the secondary structure content of native OVA (41% α-helix, 34% β-sheet, 12% β-turn and 13% random coil),[Citation2,Citation48] the four treatments can induce changes in protein secondary structure, leading to a decrease in α-helix and an increase in β-sheet and β-turn. The α-helix structure is mainly maintained by hydrogen bonds; however, due to alkali and heat treatment, the cross-linking between proteins is enhanced, causing the hydrogen bonds inside the α-helix to break, stretch and unfold, forming the β-sheet and β-turns structure. This finding is consistent with the results obtained by Zhao et al.[Citation18] According to a previous study, the ordered gel structure was mainly contributed by β-sheets, accompanied by constant conversion of α-helices.[Citation17] The two β-structure are the basis of gel formation, and the increase in the content of both is conducive to the formation of a good gel structure, increasing the gel strength.[Citation35,Citation49,Citation50] It has been well proved that there is a significant positive correlation between β-sheet content and hardness.[Citation51] In particular, the high content (53.93%) of β-sheet in HI gels supported the observation that HI gel had high hardness. The intensity of the characteristic peak is proportional to the number of chemical bonds or groups. The peaks of AI, HI, AHI, and AHIS gels are located at 1661, 1670, 1663, and 1668 cm−1, respectively. The characteristic absorption peaks of the amide I band moved toward the high wavenumber region, indicating that the protein molecules showed more interactions among themselves and formed a denser network structure and that the HI gels had a denser structure and higher hardness than the other gels.
Table 1. The secondary structure contents of OVA gels under alkali-induced (AI), heat-induced (HI), alkali-heat-induced (AHI), alkali-heat-induced in sequence (AHIS) treatments
Effect of the four treatments on the microstructure of OVA gels
The differences in the microstructure of OVA gels under different treatments show the compactness and rigidity of the network.[Citation18] Compared with the rapid aggregation of denatured protein molecules under heat-induced conditions, the aggregation rate of protein under strong alkali was slower.[Citation2,Citation3] As seen in , the HI gel protein molecules were arranged more orderly and had a compact granular structure gel structure, which exhibited several tiny pores, fewer voids overall, and a denser structure. During heating, the proteins underwent unfolding with subsequent aggregation gradually. As a result, a more compact network was formed.[Citation52] In addition, the AI OVA gel was highly cross-linked to form a network structure with many irregular and large pores, large voids and a loose structure. This conclusion corroborated with the study of Zhao et al., wherein the gel network of preserved egg white had a uniform, loose, and fine filamentous structure with many regular voids, whereas the hard-cooked egg gel showed a highly dense and stacked compact granular structure without voids.[Citation53] The gel microstructure of AHIS was more compact and denser. Upon heating, the protein molecules were further aggregated by vibration, prompting the formation of a homogeneous network structure. At the same time, heating promoted the vibration of water molecules in the gel, breaking the formed hydrogen bonds and changing the distribution of hydrophobic groups on the surface. Additionally, it also promoted the conversion of SH groups to SS bonds and facilitated further aggregation between EW proteins, leading to a more compact microstructure and greater hardness of AHIS.[Citation45] The protein molecules of the AHI gels were arranged in a more disordered manner, and the gel structure was rougher. This may be due to the combination of alkali and heat that caused irregular and violent vibrations of the protein molecules inside the gel, resulting in a rough and porous structure of the gel.
CONCLUSION
This study explored the effects of AI, HI, AHI, and AHIS treatment on the physical and chemical properties, texture and intermolecular forces of OVA protein gel. AHIS gel showed higher hardness and springiness than AI and AHI gels during aging. In addition, the AHIS gel showed dense and smooth microstructure benefiting from the secondary structure of β-structure, which contributed to the integrity and stability of the gel. Meanwhile, the WHC of AHIS gel was high, and the intermolecular force was mainly ionic bonds. AHIS treatment made the gel denature more completely and formed a more stable gel than other treatments. AHIS gel retained the semitranslucent and elastic characteristics of alkali-induced gels, while having better WHC and hardness than alkali gels, which is important for making up for the defect that alkali-induced gel is prone to liquefaction under continuous alkaline conditions. The results have particular significance in providing information regarding the formation of high-elasticity and high-strength semitransparent ovalbumin gel induced by alkali-heat treatment.
Acknowledgments
The author would like to thank everyone who volunteered to assist with the study. The authors declare no conflicts of interest.
Disclosure statement
No potential conflict of interest was reported by the author(s).
Additional information
Funding
References
- Luo, W. X.; Xue, H.; Xiong, C. H.; Li, J. K.; Tu, Y. G.; Zhao, Y. Effects of Temperature on Quality of Preserved Eggs during Storage [J]. Poultr. Sci. 2020, 99(6), 3144–3157. DOI: 10.1016/j.psj.2020.01.020.
- Zhao, Y.; Cao, D. H.; Shao, Y. Y.; Xiong, C. H.; Li, J. K.; Tu, Y. G. Changes in Physico-chemical Properties, Microstructures, Molecular Forces and Gastric Digestive Properties of Preserved Egg White during Pickling with the Regulation of Different Metal Compounds [J]. Food Hydrocolloids. 2020, 98. DOI: 10.1016/j.foodhyd.2019.105281.
- Zhang, X. W.; Jiang, A. M.; Chen, M. T.; Ockerman, H. W.; Chen, J. J. Effect of Different Alkali Treatments on the Chemical Composition, Physical Properties, and Microstructure of Pidan White [J]. J. Food Sci. Technol. 2013, 52(4), 2264–2271. DOI: 10.1007/s13197-013-1201-x.
- Zhao, Y.; Chen, Z. Y.; Li, J. K.; Xu, M. S.; Shao, Y. Y.; Tu, Y. G. Changes of Microstructure Characteristics and Intermolecular Interactions of Preserved Egg White Gel during Pickling [J]. Food Chem. 2016, 203, 323–330. DOI: 10.1016/j.foodchem.2016.02.044.
- Cai, J.; Sweeney, A. M. The Proof Is in the Pidan: Generalizing Proteins as Patchy Particles [J]. ACS Cent. Sci. 2018, 4(7), 840–853. DOI: 10.1021/acscentsci.8b00187.
- Ganasen, P.; Benjakul, S. Physical Properties and Microstructure of Pidan Yolk as Affected by Different Divalent and Monovalent Cations [J]. LWT - Food Sci. Technol. 2010, 43(1), 77–85. DOI: 10.1016/j.lwt.2009.06.007.
- Ganasen, P.; Benjakul, S. Chemical Composition, Physical Properties and Microstructure of Pidan White as Affected by Different Divalent and Monovalent Cations [J]. J. Food Biochem. 2011, 35(5), 1528–1537. DOI: 10.1111/j.1745-4514.2010.00475.x.
- Zhao, Y.; Xu, M. S.; Tu, Y. G. Research Progress in Mechanisms of Preserved Egg Processing [J]. Food Sci. 2010, 31(17), 472–475.
- Feng, T. T.; Peng, Q.; Wang, Y.; Ye, Y. Effect of Alkali Concentration in Brine on Quality of Non-heavy-metal Quickly Salted Preserved Eggs by Cascade Alkali Adjusting Method [J]. Food Ferment. Ind. 2020, 46(10), 191–196. DOI: 10.13995/j.cnki.11-1802/ts.022026.
- Zhang, D. C.; Wang, Y.; Lei, L.; Ye, Y. Effect of Curing Agent and Recombination on Quality of Salted Preserved Eggs without Heavy Metal [J]. Sci. Technol. Food Ind. 2021, 42(6), 43–49. DOI: 10.13386/j.1002-0306.2020050325.
- Zhao, Y.; Tu, Y. G.; Li, J. K.; Xu, M. S.; Yang, Y. X.; Nie, X. L.; Yao, Y.; Du, H. Y. Effects of Alkaline Concentration, Temperature, and Additives on the Strength of Alkaline-induced Egg White Gel [J]. Poultr. Sci. 2014, 93(10), 2628–2635. DOI: 10.3382/ps.2013-03596.
- Xiong, W. F.; Ren, C.; Xu, X. Y.; Li, J.; Wang, L. F.; Li, B. Thermally Induced Gelation Behavior and Fractal Analysis of Ovalbumin-carboxymethylcellulose Electrostatic Complexes [J]. Food Hydrocolloids. 2019, 91, 214–223. DOI: 10.1016/j.foodhyd.2019.01.027.
- Lassé, M.; Deb-Choudhury, S.; Haines, S.; Larsen, N.; Gerrard, J. A.; Dyer, J. M. The Impact of pH, Salt Concentration and Heat on Digestibility and Amino Acid Modification in Egg White Protein [J]. J. Food Compost. Anal. 2015, 38, 42–48. DOI: 10.1016/j.jfca.2014.08.007.
- Van Der Plancken, I.; Van Loey, A.; Hendrickx, M. E. Effect of Heat-treatment on the Physico-chemical Properties of Egg White Proteins: A Kinetic Study [J]. J. Food Eng. 2006, 75(3), 316–326. DOI: 10.1016/j.jfoodeng.2005.04.019.
- Van Der Plancken, I.; Van Loey, A.; Hendrickx, M. E. G. Changes in Sulfhydryl Content of Egg White Proteins Due to Heat and Pressure Treatment [J]. J. Agricutural and Food Chemistry. 2005, 53, 5726–5733. DOI: 10.1021/jf050289.
- Chang, C. H.; Niu, F. G.; Su, Y. J.; Qiu, Y.; Gu, L. P.; Yang, Y. J. Characteristics and Emulsifying Properties of Acid and Acid-heat Induced Egg White Protein [J]. Food Hydrocolloids. 2016, 54, 342–350. DOI: 10.1016/j.foodhyd.2015.09.026.
- Gao, X. J.; Yao, Y.; Wu, N.; Xu, M. S.; Zhao, Y.; Tu, Y. G. The Underlying Mechanism of Alkali-induced Ovalbumin Gel Transforms to Sol: Physicochemical Properties, Structure and Quantitative Protein Degradation Analysis [J]. Food Hydrocolloids. 2021, 120. DOI: 10.1016/j.foodhyd.2021.106954.
- Zhao, Y.; Chen, Z. Y.; Li, J. K.; Xu, M. S.; Shao, Y. Y.; Tu, Y. G. Formation Mechanism of Ovalbumin Gel Induced by Alkali [J]. Food Hydrocolloids. 2016, 61, 390–398. DOI: 10.1016/j.foodhyd.2016.04.041.
- Gao, X. J.; Yao, Y.; Wu, N.; Xu, M. S.; Zhao, Y.; Tu, Y. G. The Sol-gel-sol Transformation Behavior of Egg White Proteins Induced by Alkali [J]. Int. J. Biol. Macromol. 2020, 155, 588–597. DOI: 10.1016/j.ijbiomac.2020.03.209.
- Huang, X. L.; Li, J. H.; Chang, C. H.; Gu, L. P.; Su, Y. J.; Yang, Y. J. Effects of NaOH/NaCl Pickling on Heat-induced Gelation Behaviour of Egg White [J]. Food Chem. 2019, 297. DOI: 10.1016/j.foodchem.2019.06.006.
- Li, J. H.; Zhang, Y. F.; Fan, Q.; Teng, C. H.; Xie, W. Y.; Shi, Y.; Su, Y. J.; Yang, Y. J. Combination Effects of NaOH and NaCl on the Rheology and Gel Characteristics of Hen Egg White Proteins [J]. Food Chem. 2018, 250, 1–6. doi: 10.1016/j.foodchem.2018.01.031.
- Chen, Z. Y.; Li, J. K.; Tu, Y. G.; Zhao, Y.; Luo, X. Y.; Wang, J. J.; Wang, M. L. Changes in Gel Characteristics of Egg White under Strong Alkali Treatment [J]. Food Hydrocolloids. 2015, 45, 1–8. DOI: 10.1016/j.foodhyd.2014.10.026.
- Zhu, Y.; Vanga, S. K.; Wang, J.; Raghavan, V. Impact of Food Processing on the Structural and Allergenic Properties of Egg White [J]. Trends Food Sci. Technol. 2018, 78, 188–196. DOI: 10.1016/j.tifs.2018.06.005.
- Ji, L.; Liu, H. P.; Cao, C. L.; Liu, P. W.; Wang, H.; Wang, H. N. Chemical and Structural Changes in Preserved White Egg during Pickled by Vacuum Technology [J]. Food Sci. Technol. Int. 2012, 19(2), 123–131. DOI: 10.1177/1082013212442186.
- Croguennec, T.; Renault, A.; Beaufils, S.; Dubois, -J.-J.; Pezennec, S. Interfacial Properties of Heat-treated Ovalbumin [J]. J. Colloid Interface Sci. 2007, 315(2), 627–636. 10.1016/j.jcis.2007.07.041.
- Stǎnciuc, N.; Banu, I.; Turturicǎ, M.; Aprodu, I. pH and Heat Induced Structural Changes of Chicken Ovalbumin in Relation with Antigenic Properties [J]. Int. J. Biol. Macromol. 2016, 93(Pt A), 572–581. DOI: 10.1016/j.ijbiomac.2016.09.025.
- Deng, W. X.; Wang, Y.; Zhou, X. Y.; Liu, Z. S.; Ye, Y. Effect of Heat Induction on Physicochemical Properties of Egg White during Egg Pickling [J]. Food Sci. Technol. 2021, 46(11), 74–80. DOI: 10.13684/j.cnki.spkj.2021.11.012.
- Luo, M. J.; Luo, C. X., and Wu, G. X. Determination of Sulfhydryl Group and Disulfide Chain of Protein in Food by Ellman’s Reagent Colorimetric Method [J]. J. Zhengzhou Grain College 1986, 1, 92–95. doi:10.16433/j.cnki.issn1673-2383.1986.01.013.
- Deng, C.; Shao, Y. Y.; Xu, M. S.; Yao, Y.; Wu, N.; Hu, H. L.; Zhao, Y.; Tu, Y. G. Effects of Metal Ions on the Physico-chemical, Microstructural and Digestion Characteristics of Alkali-induced Egg White Gel [J]. Food Hydrocolloids. 2020, 107. DOI: 10.1016/j.foodhyd.2020.105956.
- Wu, H.; Wang, Y.; Jiang, Q.; Jiang, X.; Feng, Q.; Shi, W. Changes in Physicochemical Properties and Myofibrillar Protein Properties in Grass Carp Salted by Brining and Injection [J]. Int. J. Food Sci. Technol. 2021, 56(11), 5674–5687. DOI: 10.1111/ijfs.15108.
- Bao, Z. J.; Wu, J. P.; Cheng, Y.; Chi, Y. J. Effects of Lipid Peroxide on the Structure and Gel Properties of Ovalbumin [J]. Process Biochem. 2017, 57, 124–130. DOI: 10.1016/j.procbio.2017.03.009.
- Nieuwland, M.; Bouwman, W. G.; Pouvreau, L.; Martin, A. H.; De Jongh, H. H. J. Relating Water Holding of Ovalbumin Gels to Aggregate Structure [J]. Food Hydrocolloids. 2016, 52, 87–94. DOI: 10.1016/j.foodhyd.2015.06.018.
- Wu, M. G.; Cao, Y.; Lei, S. M.; Liu, Y.; Wang, J. H.; Hu, J.; Li, Z. K.; Liu, R.; Ge, Q. F.; Yu, H. Protein Structure and Sulfhydryl Group Changes Affected by Protein Gel Properties: Process of Thermal-induced Gel Formation of Myofibrillar Protein [J]. Int. J. Food Prop. 2019, 22(1), 1834–1847. DOI: 10.1080/10942912.2019.1656231.
- Zou, W. J.; Mourad, F. K.; Zhang, X. Y.; Ahn, D. U.; Cai, Z. X.; Jin, Y. G. Phase Separation Behavior and Characterization of Ovalbumin and Propylene Glycol Alginate Complex Coacervates [J]. Food Hydrocolloids. 2020, 108. DOI: 10.1016/j.foodhyd.2020.105978.
- Xue, H.; Tu, Y. G.; Xu, M.; Liao, M. F.; Luo, W. X.; Guo, W. B.; Zhang, G. W.; Zhao, Y. Changes in Physicochemical Properties, Gel Structure and in Vitro Digestion of Marinated Egg White Gel during Braising [J]. Food Chem. 2020, 330. DOI: 10.1016/j.foodchem.2020.127321.
- Mine, Y. Recent Advances in the Understanding of Egg White Protein Functionality [J]. Trends Food Sci. Technol. 1995, 6(7), 225–232. DOI: 10.1016/S0924-2244(00)89083-4.
- Guo, W. B.; Zhao, Y.; Yao, Y.; Wu, N.; Xu, M. S.; Du, H. Y.; Tu, Y. G. Relationship between Protein Structure Changes and in Vitro Digestion of Preserved Egg White during Pickling [J]. Int. J. Biol. Macromol. 2019, 138, 116–124. DOI: 10.1016/j.ijbiomac.2019.07.057.
- Chen, K.; Chen, X.; Liang, L.; Xu, X. L. Gallic Acid-aided Cross-linking of Myofibrillar Protein Fabricated Soluble Aggregates for Enhanced Thermal Stability and a Tunable Colloidal State [J]. J. Agric. Food Chem. 2020, 68(41), 11535–11544. DOI: 10.1021/acs.jafc.0c02059.
- Gharbi, N.; Labbafi, M. Effect of Processing on Aggregation Mechanism of Egg White Proteins [J]. Food Chem. 2018, 252(252), 126–133. DOI: 10.1016/j.foodchem.2018.01.088.
- Kang, Z. L.; Wang, P.; Xu, X. L.; Zhu, C. Z.; Li, K.; Zhou, G. H. Effect of Beating Processing, as a Means of Reducing Salt Content in Frankfurters: A Physico-chemical and Raman Spectroscopic Study [J]. Meat Sci. 2014, 98(2), 171–177. DOI: 10.1016/j.meatsci.2014.05.025.
- Pérez-Mateos, M.; Lourenco, H.; Montero, P. Borderías, A. J. Rheological and Biochemical Characteristics of High-Pressure- and Heat-Induced Gels from Blue Whiting (Micromesistius Poutassou) Muscle Proteins. J. Agricutural and Food Chemistry. 1997, 451, 44–49. DOI:10.1021/jf960185m.
- Khemakhem, M.; Attia, H.; Ayadi, M. A. The Effect of pH, Sucrose, Salt and Hydrocolloid Gums on the Gelling Properties and Water Holding Capacity of Egg White Gel [J]. Food Hydrocolloids. 2019, 87, 11–19. DOI: 10.1016/j.foodhyd.2018.07.041.
- Urbonaite, V.; De Jongh, H. H. J.; Van Der, L. E.; Pouvreau, L. Permeability of Gels Is Set by the Impulse Applied on the Gel [J]. Food Hydrocolloids. 2015, 50, 7–15. DOI: 10.1016/j.foodhyd.2015.03.024.
- Urbonaite, V.; De Jongh, H. H. J.; Van Der, L. E.; Pouvreau, L. Water Holding of Soy Protein Gels Is Set by Coarseness, Modulated by Calcium Binding, Rather than Gel Stiffness [J]. Food Hydrocolloids. 2015, 46, 103–111. DOI: 10.1016/j.foodhyd.2014.12.010.
- Ai, M. M.; Zhou, Q.; Xiao, N.; Guo, S. G.; Cao, Y. Y.; Fan, H.; Ling, Z. T.; Zhou, L. D.; Li, S. C.; Long, J. L., et al. Enhancement of Gel Characteristics of NaOH-induced Duck Egg White Gel by Adding Ca(OH)2 With/without Heating [J]. Food Hydrocolloids. 2020, 103. DOI: 10.1016/j.foodhyd.2020.105654.
- Fernández-López, J.; Martínez, A.; Fernández-Ginés, J. M.; Sayas-Barberá, E.; Sendra, E.; Pérez-Alvarez, J. A. Gelling and Color Properties of Ostrich (Struthio Camelus) Egg White [J]. J. Food Qual. 2006, 29(2), 171–183. DOI: 10.1111/j.1745-4557.2006.00065.x.
- Herrero, A. M. Raman Spectroscopy for Monitoring Protein Structure in Muscle Food Systems [J]. Food Sci. Nutr. 2008, 48(6), 512–523. DOI: 10.1080/10408390701537385.
- Ngarize, S.; Herman, H.; Adams, A.; Howell, N. Comparison of Changes in the Secondary Structure of Unheated, Heated, and High-pressure-treated Beta-lactoglobulin and Ovalbumin Proteins Using Fourier Transform Raman Spectroscopy and Self-deconvolution [J]. J. Agricultural and Food Chemistry. 2004, 5221, 6470–6477. DOI:10.1021/jf030649y.
- Xu, L. L.; Zhao, Y.; Xu, M. S.; Yao, Y.; Wu, N.; Du, H. Y.; Tu, Y. G. Changes in Physico-chemical Properties, Microstructure, Protein Structures and Intermolecular Force of Egg Yolk, Plasma and Granule Gels during Salting [J]. Food Chem. 2019, 275, 600–609. DOI: 10.1016/j.foodchem.2018.09.078.
- Li, K.; Zhao, Y. Y.; Kang, Z. L.; Wang, H. H.; Ma, H. J.; Xu, X. L. Effect of NaCl on Processing Characteristics and Protein Secondary Structures in Pork Meat Batters [J]. Food Sci. 2017, 38(15). DOI: 10.7506/spkx1002-6630-201715013.
- Yang, Y.; Zhao, Y.; Xu, M.; Yao, Y.; Wu, N.; Du, H.; Tu, Y. Effects of Strong Alkali Treatment on the Physicochemical Properties, Microstructure, Protein Structures, and Intermolecular Forces in Egg Yolks, Plasma, and Granules [J]. Food Chem. 2020, 311, 125998. DOI: 10.1016/j.foodchem.2019.125998.
- Kaewmanee, T.; Benjakul, S.; Visessanguan, W. Effects of Salting Processes and Time on the Chemical Composition, Textural Properties, and Microstructure of Cooked Duck Egg [J]. J. Food Sci. 2011, 76(2), S139–S147. DOI: 10.1111/j.1750-3841.2010.01975.x.
- Zhao, Y.; Tu, Y. G.; Xu, M. S.; Li, J. K.; Du, H. Y. Physicochemical and Nutritional Characteristics of Preserved Duck Egg White [J]. Poultr. Sci. 2014, 93(12), 3130–3137. DOI: 10.3382/ps.2013-03823.