ABSTRACT
Growth inhibition of Streptococcus mutans (SM), Streptococcus salivarius (SS1), and Streptococcus sanguinis (SS2) were determined at different Sparassis latifolia polysaccharides (SLPs) concentrations (2%, 4%, 8%, 16%) mixed with artificial saliva (AS). Metabolomics was determined by the action of 4% SLPs and AS on each bacterium to obtain the bacteriostatic activity of SLPs on oral processing. The results showed that the 16% mixture could inhibit the growth of the oral bacteria S. mutans, S. salivarius, and S. sanguinis. The inhibitory effect on oral bacteria decreased with decreasing polysaccharide concentration. The metabolic pathways and metabolites analyses screened for active inhibitory substances against oral bacteria. These results implied that SLPs are potential new material for developing oral functional products.
Introduction
Food oral processing, the first stage of food decomposition in the human body, is important for nutrient absorption to gain energy and sensory pleasure.[Citation1] In the twentieth century, Hutchings et al.[Citation2] proposed a 3D model of food processing in the mouth (), with individual differences and complexity in the fragmentation of food within the oral cavity. Saliva secretion induced by food stimulation plays a very important role.[Citation3] Saliva, composed mainly of 99% water and 1% organic matter, is a continuous phase buffer medium consisting of a gel network of water, electrolytes, highly glycosylated mucins with poor water-soluble proteins, water-insoluble lipids, bacterial cells, and epithelial cells.[Citation4] Saliva promotes swallowing and has a specific cleaning ability. Therefore, saliva is considered when conducting in vitro simulated oral processing studies. Nevertheless, artificial saliva is usually used in such studies due to the complexity of natural saliva composition.[Citation5] Okra polysaccharides and Thai plant mucilage were mixed with artificial saliva for in vitro simulated oral treatment, and these extracts were found to work well with artificial saliva.[Citation6,Citation7]
Figure 1. The 3D model of food processing in the mouth: (1) tender juicy steak; (2) tough dry meat; (3) dry sponge cake; (4) oyster; (5) liquids. Before a food may be swallowed, its ‘degree of structure’ must have been reduced below the level of plane ABCD, and its ‘degree of lubrication’ must have crossed plane EFGH.
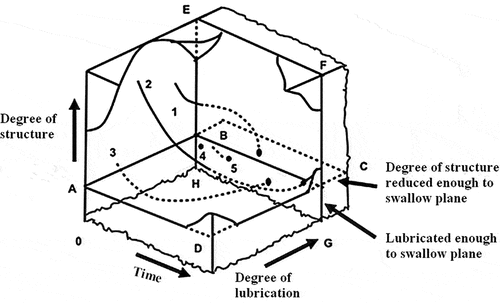
An excellent oral micro ecological environment is the primary condition for food oral processing. Saliva has a certain ability to clean the oral environment. However, there are still many bacteria that affect the micro-ecological health of the oral cavity and cause problems while eating. Studies show Streptococcus mutans is the primary bacterium causing dental caries through adhesion and dental plaque formation.[Citation8] Further expansion of plaque caused by food residues produces acidity that may lead to inflammation and mouth ulcers.[Citation9] Other bacteria, including Streptococcus sanguinis and Streptococcus salivarius, could grow in the environment provided by S. mutans, promoting tooth decay.[Citation10] Moreover, they also generate some smelly secondary products during food residues decomposition, producing halitosis.[Citation11] Therefore, oral products and medicines are necessary to improve the oral microecological environment. Nowadays, scientists prefer plant-derived natural active ingredients to develop products, such as plant volatile oils and alcohols, which can inhibit oral bacteria’s growth and acid production and reduce the possibility of dental caries.[Citation8,Citation12] Compared with synthetic chemicals, natural ingredients do not irritate the oral mucosa or have adverse effects on people.[Citation13]
Polysaccharides formed by aldose and ketose dehydration are linear or branched chains linked by glycoside bonds. Polysaccharides are very important biomacromolecules, ubiquitous in plants, animals, and microbial tissues, with many biological activities, including antibiosis and anti-inflammatory properties.[Citation14] Therefore, they have great development potential in food nutrition and medicine. With the advancement of polysaccharide research and industrial development, polysaccharides have been widely used in food, medicine, materials, and other fields. In food oral processing, okra polysaccharides in a concentration range of 0.25% to 1% work well with artificial saliva to promote swallowing.[Citation6] Polysaccharides from Paeonia sinjiangensis K.Y. Pan have different inhibitory effects on the growth and acid production of S. mutans and S. sanguinis.[Citation15] Lentinus edodes polysaccharide heal phenol-induced oral ulceration faster using chemicals and significantly raised serum antioxidant enzymes activities in rats with oral ulceration.[Citation16] Also, acemannan, a polysaccharide extracted from Aloe vera, was more effective in reducing ulcer size and pain than chemicals. In clinical trials, patients were mainly satisfied after 0.1% triamcinolone acetonide and acemannan treatment.[Citation17]
Sparassis latifolia is an edible mushroom with a dual function in medicine, whose main characteristic is a β-glucan content of more than 40%.[Citation18] S. latifolia polysaccharides (SLPs) have antimicrobial and anti-inflammatory activities in virtue of the β-glucan chains. SLPs have some inhibitory effects on bacteria and a few fungi. A specific concentration of SLPs can inhibit common foodborne pathogenic bacteria, such as Staphylococcus aureus, Escherichia coli, and Listeria.[Citation19] Water extract of S. latifolia polysaccharides could restrain allergic inflammatory reactions, such as food allergy, asthma, and atopic dermatitis, by decreasing pro-inflammatory cytokines.[Citation20] Woo-Suk[Citation21] found that S. latifolia extracts play an inhibitory role in inflammatory reactions via regulating nitric oxide (NO) production. However, the antibacterial effect of SLPs after oral processing is rarely reported. Therefore, in this research, SLPs were mixed with artificial saliva to study their antifungal bioactivity in oral processing in vitro. The possible mechanisms of SLPs inhibiting oral bacteria are described based on the non-targeted metabolomics analysis of secondary products. We hope this information about SLPs will be useful in developing new oral products sources.
Materials and methods
Materials
Dried S. latifolia was provided by the Edible Fungi Research Center of Shanxi Agricultural University (Shanxi, China). Artificial saliva was purchased from Shanghai Yuanye Bio-Technology Co., Ltd (China). Streptococcus mutans ATCC 25715, Streptococcus sanguinis ATCC 49295, and Streptococcus salivarius ATCC13419 were purchased from Shanghai Bioresource Collection Center (China). All other chemicals used were analytical grade.
Methods
Isolation and purification of polysaccharides
Dried S. latifolia was milled for 10 minutes in an ultra-mizer (JS14S, Jiangyin Xiangda Machinery Manufacturing Co., Ltd, China) and sifted through a 200-mesh sieve. Powdered S. latifolia was diluted in distilled water according to the liquid ratio 1:16 (g/ml). The pH was adjusted to 4.2 ± 0.1 with 0.375% papain, 0.375% cellulase, and 0.25% pectinase; then, the extract was incubated in water at 54°C for 3.5 h and inactivated at 100°C for 10 min. The extract was centrifuged at 4500 r/min for 20 min, and the supernatant was concentrated using a rotary evaporator (Büchi Labortechnik AG, Switzerland). Three volumes of anhydrous ethanol were added to the concentrate and precipitated at 4°C for 8 h. The ethanol solution was recovered by centrifugation at 4500 r/min for 5 min, and the precipitate was collected. Precipitates were washed with acetone and clarified with an ether solution. The polysaccharides were denuded of proteins using the sevag reagent. The supernatant was purified with a macroporous resin and freeze-dried in a vacuum (Vacuum freeze dryer PL 3000, Thermo, USA) to obtain the SLPs.[Citation22,Citation23, Citation24]Through structural identification, the relative molecular mass of SLPs were found to be 1.04 × 104 ~ 6.9 × 106 u, the main chain of the obtained SLPs was β-(1→3)-D-glucan, and the branch was β-(1→6)-D-glucan, composed of galactose, glucose, xylose, mannose, and fructose with a molar ratio of 10.55: 24.76: 2.51: 5.64:1.3.
Preparation of bacterial suspension
According to the instructions, the experimental strains S. mutans, S. sanguinis, and S. salivarius, herein SM, SS1, and SS2, were activated and inoculated in bovine brain immersion fluid liquid medium. Growth curves were recorded with a UV spectrophotometer (UV-1200, Shanghai Metrum Instrument Co., Ltd, China) at OD630, and the corresponding number of colonies was determined by the dilution-coated plate method. The corresponding bacterial concentration was 105 ~ 106 CFU/mL at OD630 of 0.46 ± 0.1.
Inhibitory rate and pH values assay: Prior to performing antimicrobial experiments, purchased artificial saliva (AS) was determined to have no inhibitory effect on the experimental strains. SLPs solutions of 2%, 4%, 8%, and 16% concentrations were autoclaved, refrigerated at 4°C, and mixed with AS at 4:1 (v/v). For the control group, liquid medium was mixed with AS at 4:1 (v/v) and with each bacterial suspension at 1:9 (v/v). Different concentrations of SLPs and AS were mixed with each bacterial suspension at 1:9 (v/v) for the experimental group, and all groups’ initial pH was adjusted to 7.20 ± 0.01. The groups were cultured in an anaerobic incubator (YQX-II, Shanghai Yuejin Medical Device Co., Ltd, China) at 37°C. The OD630 and pH values were determined at 1, 3, 5, 10, 15, 20, 25, 30, 35, 40, 45, 50, 55, and 60 min. The antibacterial rate was calculated using the formula: Antibacterial rate (%) = [OD1-(OD2-OD3)]/OD2, where OD1 is control group absorbance, OD2 is experimental group absorbance, OD3 is the absorbance of polysaccharide and artificial saliva (4:1 v/v) mixed with liquid nutrient medium (1:9 v/v).[Citation25]
Non-targeted metabolomics assay
The polysaccharides concentration for the metabolomics test was determined based on the experimental results of the antibacterial rate and pH values. The experiment was divided into control groups (SM+AS, SS1+ AS, and SS2+ AS), SLPs group (SLPs+AS), and bacteria with SLPs groups (SLPs+AS+SM, SLPs+AS+SS1, SLPs+AS+SS2). For the control groups, liquid medium was mixed with AS (4:1 v/v) and mixed with each bacterial suspension at 1:9 (v/v), named SM (S. mutans), SS1 (S. sanguinis), and SS2 (S. salivarius). SLPs groups: 4% SLPs were mixed with AS (4:1 v/v), named SLPs. Bacteria with SLPs group: 4% SLPs were mixed with AS (4:1 v/v) and with the experimental strain (9:1 v/v), named SMP, SS1P, and SS2P. The three groups were cultured for 6 h at 37°C, then the supernatants of the three groups were centrifuged at 4°C 11000 g for 20 min using 0.22 m super-filter membrane filtration, quenched with liquid nitrogen and stored at −80°C.
The liquid mass coupling system for metabolomics analysis consists of a Waters superefficient liquid phase (I-Class, Waters Technology (Shanghai) Co., Ltd, China) and Waters high-resolution mass spectrometer (G2-XS, Waters Technology (Shanghai) Co., Ltd, China) with an HSS T3 chromatography column (1.8um2.1 * 100 mm). Raw data were collected for primary and secondary mass spectrometry data using the MassLynx V4.2 with MSe mode. Data processing, including peak extraction and peak alignment, was performed by Progenesis QI. Metabolite identification was performed based on the Progenesis QI online METLIN database, theoretical fragment identification, and mass number deviations within 100ppm.
Statistical analysis
All experiments were repeated thrice, and results are reported as the means ± standard deviation (SD). Metabolomics raw data were normalized by the total peak area. The relative quantitative values of the metabolite were calculated as each metabolite per sample divided by the total peak area of that sample. Other data were analyzed using SPSS statistics 25, and statistically different samples were identified by the Duncan method, independent sample by T-test, and mapping using Origin 2021.
Results
SLPs inhibited the growth of oral bacteria
The inhibition rate of oral bacteria by different SLPs concentrations is shown in . Within 60 min of simulated oral processing, SLPs had an inhibitory effect on S. mutans, S. salivarius, and S. sanguinis, and their inhibitory effect was concentration-dependent. The 16% SLPs had the strongest inhibition, with inhibition rates for S. mutans, S. salivarius, and S. sanguinis of 87.86 ± 3.25%, 93.78 ± 2.34%, and 89.09 ± 0.33%, respectively.
SLPs inhibited oral bacterial acid production
Inhibition of S. mutans acid production by different SLPs concentrations is shown in . The 16% SLPs significantly inhibited the S. mutans acid production capacity (p < 0.01), and the acid production inhibition decreased with the decreased SLPs concentration. The 2% SLPs did not inhibit acid production, and the 2% SLPs group produced more acid than the control group over time. Changes in S. salivarius and S. sanguinis acidity were not apparent over the one-hour determination time, as shown in . SLPs still restrained the acid production of the two bacteria.
Table 1. Inhibition of acid production of S. mutans by different concentrations of SLPs
Table 2. Inhibition of acid production of S. salivarius by different concentrations of SLPs
Table 3. Inhibition of acid production of S. sanguinis by different concentrations of SLPs
Total metabolic pathways and metabolites
The total metabolic pathways and metabolites of S. mutans, S. salivarius, and S. sanguinis are shown in . Six hundred and twenty-six species and 366 metabolic pathways, such as amino acid metabolism, fatty acid metabolism, sugar metabolism, vitamin and ubiquitin metabolism, were identified. The secondary map of the public database was used for the metabolites’ secondary map matching, and the second map was classified based on the Human Metabolome Database (HMDB) classification information. The statistical results are shown in . The HMDB classification classified metabolites into organic heterocyclic compounds, organic oxygen compounds, organic acids and their derivatives, nucleotides and analogs, lipids and lipid-like molecules. The metabolites were mainly concentrated in 302 lipids and lipid compounds, 169 organic heterocyclic compounds, and 121 organic acids and their derivatives. The secondary map metabolites were submitted to the Kyoto Encyclopedia of Genes and Genomes (KEGG) for metabolic pathway analysis, and the first 20 KEGG metabolic pathways results are shown in . The first 20KEGG metabolite enrichment pathways were divided into nine global and overview map pathways, six amino acid metabolism pathways, three fatty acid metabolism pathways, and one vitamin and ubiquitin metabolism pathway. According to the nine global and overview map pathways, 167 metabolites were enriched in metabolically related pathways, involving the biosynthesis of secondary metabolites, microbial metabolism, carbon source metabolism, 2-oxygen carboxylic acid metabolism, fatty acid metabolism, degradation of aromatic compounds, biosynthesis of amino acids, and co-factor biosynthesis. Other metabolic pathways, vitamin and ubiquitin metabolic pathway, and unsaturated fatty acids were mainly concentrated in the S. mutans, S. salivarius, and S. sanguinis experimental groups with SLPs.
SLPs reduced the total number of nucleotides
Bacterial growth requires nutrients for anabolism, and the number of nucleotides represents the DNA and RNA synthesized by the bacteria, which indirectly reflects bacterial growth. The statistics of the 22 nucleotides and their analogs are shown in . The number of nucleotides in the SMP, SS1P, and SS2P groups was significantly lower than their corresponding SM, SS1, and SS2 controls, showing that SLPs significantly inhibit the growth and reproduction of S. mutans, S. salivarius, and S. sanguinis.
SLPs reduced acid metabolites
Free hydrogen ions-producing acids were screened from organic acids and their derivatives, fatty acids and their derivatives, and amino acids, as shown in . A total of 43 acidic metabolites were produced in all groups, and the acidic composition was significantly different between the experimental and controls groups. Organic acids in the experimental group, such as ketoglutaric acid, succinic acid, norleucine acid, and indoleacetic acid, were produced in large quantities. However, the corresponding control groups produced more saturated and unsaturated fatty acids. The number of experimental acid metabolites was significantly less than the control group (p < 0.05).
The differentially produced acidic metabolites of S. mutans are shown in . In the SMP group compared to the SM group, citric acid, succinic acid, 3-indoleacrylic acid, etodolac, diaminopimelic acid, and tetrahydrodipicolinate were reduced, whereas oleic acid, erucic acid, phenylpyruvic acid increased significantly (p < .05). Compared with the SLPs group, oleic acid, erucic acid, and 3-phenylpyruvic acid were significantly increased in the SMP group (p < .05). It is shown that polysaccharides could inhibit organic acids production by S. mutans.
Table 4. The differential acid metabolites of S. mutans.
The differentially produced acidic metabolites of S. salivarius are shown in . Compared with the SS1 group, the SS1P group showed reduced citric acid, succinic acid, 3-indoleacrylic acid, etodolac, aminoadipic acid, diaminopimelic acid; contrary, tetrahydrodipicolinate, while oleic acid, docosatrienoic acid, erucic acid were increased significantly (p < .05). Compared with the SLPs group, 3-indoleacrylic acid, oleic acid, docosatrienoic acid, and erucic acid were significantly increased in the SS1P group (p < .05). Therefore, polysaccharides could inhibit organic acids produced by S. salivarius.
Table 5. The differential acid metabolites of S. salivarius.
S. sanguinis differentially produced acidic metabolites are shown in . In the SS2P group, citric acid, succinic acid, 3-Indoleacrylic acid, etodolac, diaminopimelic acid, and tetrahydrodipicolinate were reduced compared with the SS1 group, while erucic acid and docosatrienoic acid were significantly increased (p < .05). Compared with the SLPs group, 3-indoleacrylic acid and erucic acid were significantly increased in the SS2P group (p < .05). Therefore, polysaccharides inhibit organic acids produced by S. sanguinis.
Table 6. The differential acid metabolites of S. sanguinis.
SLPs reduced antibiotics
Analysis of the differential metabolites of organic heterocyclic compounds shows that differentially produced metabolites between S. mutans, S. salivarius, and S. sanguinis experimental and control groups were the secondary product antibiotics virginiamycin S1, tacrolimus, nystatin, and deoxycoformycin. The results are shown in . Although the synthesis mechanisms of these antibiotics are unclear, they did not inhibit S. mutans, S. salivarius, and S. sanguinis.
SLPs reduced odor composition
The main differential odor metabolic components between the experimental and control groups are shown in . In the control group, decomposed nutrients of S. mutans, S. salivarius, and S. sanguinis produce foul odor components, such as indole-related metabolites, putrescine, and 2-methylphenol. In the experimental group, after adding SLPs, the main odorants were megastigmatrienone, 1-octen-3-ol, 3,7-dimethyl-6-octen-1-ol, and menthol, which are the unique fragrances of S. latifolia. Moreover, the heptyl acetate and 1,5-decanolide produced under the action of oral bacteria also emitted a pleasant smell.
Metabolic map of SLPs
According to the experimental group’s metabolic pathways and metabolites analysis, the possible decomposition processes of SLPs under the respective catabolism of S. mutans, S. salivarius, and S. sanguinis are summarized in . Oral bacteria produce oligosaccharides such as stachyose, raffinose, maltose, maltoheptaose, and mannose. The glucose produced by the breakdown of oligosaccharides enters the glucose metabolic pathway to produce saturated and unsaturated fatty acid components. Fatty acids could form fatty acid ester components.
The inhibitory mechanism of SLPs on oral bacteria
Based on the differences between metabolic substances and metabolic pathways, the possible SLPs antibacterial mechanism in simulated oral processing is shown in . SLPs could inhibit glucose, arginine, and lysine metabolism in S. mutans, S. salivarius, and S. sanguinis. Lysine biosynthesis was primarily inhibited. Product inhibition, mainly of fatty acids, is the primary inhibitory effect of the SLPs metabolites on the bacteria.
Discussion
The present study mainly evaluated the oral antibiosis effect of Sparassis latifolia polysaccharides. Numerous studies have proved that SLPs have antibacterial and anti-inflammatory effects due to their large β-glucan content.[Citation19–21] Our study considered the oral processing behavior, the saliva secretion stimulated by food, and the complexity of the oral environment in the antibacterial effect of polysaccharides. To initially explore the oral antimicrobial effects of SLPs, we simulated oral processing in vitro by different polysaccharide proportions with artificial saliva before conducting experiments referred to in other studies.[Citation6,Citation25] The oral antibacterial effect of SLPs was explored based on the experimental results.
Given the transient duration of oral processing behavior, the time to measure bacterial inhibition was shortened to 1 h. As a validation, the bacteria concentration was adjusted to the growth log-phase range. The results of the antibacterial rate proved that SLPs had inhibitory effects on oral bacteria S. mutans, S. salivarius, and S. sanguinis and enhanced with increasing SLPs concentration. However, when the concentration was below 4%, there was no significant inhibitory effect on the oral bacteria. Furthermore, the conditions provided the growth material for the oral bacteria over time. S. mutans, S. salivarius, and S. sanguinis are acid-producing bacteria, particularly S. mutans.[Citation8–10] Compared with S. salivarius and S. sanguinis, S. mutans was the main acid-producing bacteria, as demonstrated by pH changes within an hour. To further explore the inhibition mechanism of polysaccharides on oral bacteria, untargeted metabolomics of 4% SLPs and artificial saliva mixture was determined based on the antibacterial rate results.
The metabolic pathways of oral bacteria included sugar metabolism, fatty acid metabolism, and amino acid metabolism. At the same time, the metabolites were classified in organic heterocyclic compounds, organic oxygen compounds, organic acids and their derivatives, nucleotides and analogs, and lipids and lipid molecules. The statistical analysis of metabolites associated with oral diseases was studied based on the metabolomics results. The growth and reproduction of the bacteria require genetic information, namely DNA and RNA replication, and the number of nucleotides and their derivatives is directly related to the growth of the bacteria.[Citation26] From the comparison of nucleotide number, SLPs had an inhibitory effect on oral bacteria S. mutans, S. salivarius, and S. sanguinis.
Additionally, oral bacterium S. mutans is an acid-resistant species that uses nutrients to produce acidic metabolites, leading to many oral diseases such as dental caries, oral ulcers, and periodontitis.[Citation9,Citation27] So, it was essential to focus on acidic metabolites that produced free hydrogen ions. The total amount of acid metabolites enriched in the glucose metabolism pathway of S. mutans, S. salivarius, and S. sanguinis was reduced after adding SLPs, and this result was consistent with the measured pH changes. The main chain of polysaccharides was β-(1→3)-D-glucan, and the branched-chain was β-(1→6)-D-glucan,[Citation28] which the oral bacteria could not decompose or decompose slowly, so the sugar metabolism pathway and organic acid content decreased. Conversely, the saturated and unsaturated fatty acids were increased under the action of oral bacteria. Moreover, fatty acids were less harmful than organic acids of oral stimulation. One reason for this is that the polysaccharides contained partial glycolipid molecules, which decompose from the polysaccharide-branched chain during bacterial decomposition. Previous studies found that bacteria could use the type II fatty acid synthesis system to synthesize saturated and unsaturated fatty acids and different kinds of fatty acids in different bacteria.[Citation29,Citation30]
Other differential metabolites were the secondary product antibiotics Virginiamycin S1, Tacrolimus, Nystatin, and Deoxycoformycin. Antibiotics are a class of secondary metabolites with antipathogens or other activities by microorganisms, higher plants, and animals, which interfere with the development functions of other living cells.[Citation31] The reduction of antibiotics secondary metabolites may threaten the growth of the bacteria themselves.[Citation32]
In oral diseases, halitosis is also a complex problem to overcome, and oral microorganisms such as S. mutans, S. salivarius, S. sanguinis, and some other anaerobic bacteria are closely related to halitosis and even lead to cancer.[Citation11] Obviously, the inhibitory effect of polysaccharides on oral bacteria could produce fewer odor substances and reduce the risk of fetid breath. However, with time, the oral bacteria would decompose some SLPs, mainly in the chain of α-glycoside bonds, resulting in odor. This study found that the odor-producing metabolic components differed significantly from the control group after adding polysaccharides. Moreover, adding SLPs allowed the production of pleasant-smelling substances instead of foul-smelling substances.
Combined with the metabolomics results, it can be speculated that the inhibition of oral bacteria S. mutans, S. salivarius, and S. sanguinis by SLPs might result from three mechanisms. Firstly, SLPs inhibited glucose, tryptophan, and lysine metabolism. In the acidic metabolites, the main substances in the glucose metabolism pathway, citric acid and succinate, decreased after adding SLPs. KEGG metabolite-enriched pathways and different metabolites showed that the arginine and tryptophan metabolism was reduced. The foul-smelling aminopropylcadaverine, N-acetylputrescine, and putrescine are arginine intermediate metabolites.[Citation33] Etodolac and 3-Indoleacrylic acid are tryptophan metabolites.[Citation34] These components were all significantly reduced upon the addition of SLPs. Secondly, lysine biosynthesis inhibition. A study shows that lysine acetylation of proteins play a key role in regulating bacterial metabolism, transcription, translation, and stress response to environmental changes.[Citation35] In S. mutans, lysine acetylation also plays a role in fine-tuning regulation of protein activities associated with biofilm formation.[Citation36] A decrease in lysine synthesis could affect lysine acetylation. The diaminopimelic acid and tetrahydrodipicolinate, intermediaries in lysine biosynthesis, decreased after the addition of SLPs. Thus, SLPs could affect bacterial growth by inhibiting lysine synthesis. Thirdly, substances produced by bacterial decomposition of SLPs, such as fatty acid and 3-Phenylpyruvic acid can inhibit S. mutans, S. salivarius, and S. sanguinis growth. Fatty acids are anionic surfactants with antimicrobial properties at low pH.[Citation37] Moreover, selectively act against Gram-positive bacteria by targeting bacterial cell walls and membranes.[Citation38] The hydrophobic parts of the fatty acids can partition the lipids of the bacterial cell membrane, thereby disturbing the structures of oral bacteria.[Citation39] In addition, acting on glycolytic enzymes strengthens the glycolytic pathway and reduces acid production activity.[Citation40] Thus, the major metabolites, oleic acid, docosatrienoic acid, erucic acid, and other fatty acid metabolites, inhibit the growth. Moreover, the caries prevention properties of the acid metabolites phenyl-pyruvic acid have been reported in the literature. 3-Phenylpyruvic acid is a strong bactericidal substance,[Citation41] inhibiting Gram-positive and Gram-negative bacteria and fungi.[Citation42] S. mutants, S. salivarius, and S. sanguinis are Gram-positive bacteria.
Our study preliminarily demonstrated that S. latifolia polysaccharide had an inhibitory effect on the growth of oral bacteria S. mutans, S. salivarius, and S. sanguinis, and the protective effect on the mouth was mainly reflected in reducing the generation of organic acids and unpleasant odors. These results showed that SLPs might be used as new oral materials in oral diseases treatment. Nevertheless, there were some shortcomings. Our study selected anticaries and antibacterial substances utilizing untargeted metabolomics techniques. There were also some errors in metabolomics, so other systems biology research methods are needed to obtain more reliable and definite research results in terms of content and function. Furthermore, the inhibition mechanism of SLPs against oral bacteria S. mutans, S. salivarius, and S. sanguinis is not clear. The production mechanism and the specific antibacterial mechanism of active fatty acid products need further study, and the antibiotics also deserve our attention.
Author contributions
JG completed the literature searches, review and drafted the manuscript. Other authors (SY, JC, YC, FC, CF) reviewed, edited, and approved the manuscript for its intellectual content
Disclosure statement
No potential conflict of interest was reported by the author(s).
Additional information
Funding
References
- Devezeaux De Lavergne, M.; Young, A. K.; Engmann, J.; Hartmann, C. Food Oral Processing-An Industry Perspective. Front Nutr. 8, 202124, 10.3389/fnut.2021.634410.
- Hutchings, J. B.; Lillford, P. The Perception of Food Texture‐the Philosophy of the Breakdown Path. J. Texture Stud. 1988, 19, 103–115. DOI: 10.1111/j.1745-4603.1988.tb00928.x.
- Bozorgi, C.; Holleufer, C.; Wendin, K. Saliva Secretion and swallowing—The Impact of Different Types of Food and Drink on Subsequent Intake. Nutrients. 2020, 12, 256. DOI: 10.3390/nu12010256.
- Sarkar, A.; Ye, A.; Singh, H. Oral Processing of Emulsion Systems from a Colloidal Perspective. Food Funct. 2017, 8, 511–521. DOI: 10.1039/c6fo01171c.
- Panda, S.; Chen, J.; Benjamin, O. Development of Model Mouth for Food Oral Processing Studies: Present Challenges and Scopes. Innov. Food Sci. Emerg. Technol. 2020, 102524. DOI: 10.1016/j.ifset.2020.102524.
- Yuan, B.; Ritzoulis, C.; Chen, J. Extensional and Shear Rheology of Okra Polysaccharides in the Presence of Artificial Saliva. NPJ Sci. Food. 2018, 2, 1–6. DOI: 10.1038/s41538-018-0029-1.
- Manosroi, A.; Pattamapun, K.; Khositsuntiwong, N.; Kietthanakorn, B.-O.; Issarangporn, W.; Chankhampan, C.; Manosroi, W., Manosroi, J. Physicochemical Properties and Biological Activities of Thai Plant Mucilages for Artificial Saliva Preparation. Pharm. Biol. 2015, 53(11), 1653–1660. DOI: 10.3109/13880209.2014.1001402.
- Daneshkazemi, A.; Zandi, H.; Davari, A.; Vakili, M; Emtiazi, M; Lotfi, R.; Masoumi, S.M.R . Antimicrobial Activity of the Essential Oil Obtained from the Seed and Oleo-gum-resin of Ferula Assa-foetida against Oral Pathogens. Front Dent16. 2019, 113. 10.18502/fid.v16i2.1362.
- Porter, S. R.; Leao, J. C. Oral Ulcers and Its Relevance to Systemic Disorders. Aliment. Pharmacol. Ther. 2005, 21, 295–306. DOI: 10.1111/j.1365-2036.2005.02333.x.
- Pitts, N. B.; Zero, D. T.; Marsh, P. D.; Ekstrand, K.; Weintraub, J. A.; Ramos-Gomez, F.; Tagami, J.; Twetman, S.; Tsakos, G.; Ismail, A., et al. Dental Caries. Nat. Rev. Dis. Primers. 2017, 3, 1–16. DOI: 10.1038/nrdp.2017.30.
- Tanda, N.; Hoshikawa, Y.; Ishida, N.; Sato, T.; Takahashi, N.; Hosokawa, R., and Koseki, T. Oral Malodorous Gases and Oral Microbiota: From Halitosis to Carcinogenesis. J. Oral Biosci 2015, 57, 175–178. DOI: 10.1016/j.job.2015.05.004.
- Bin, C.; Al-Dhabi, N. A.; Esmail, G. A.; Arokiyaraj, S., and Arasu, M. V. Potential Effect of Allium Sativum Bulb for the Treatment of Biofilm Forming Clinical Pathogens Recovered from Periodontal and Dental Caries. Saudi J. Biol. Sci. 2020, 27, 1428–1434. DOI: 10.1016/j.sjbs.2020.03.025.
- Palombo, E. A. Traditional Medicinal Plant Extracts and Natural Products with Activity against Oral Bacteria: Potential Application in the Prevention and Treatment of Oral Diseases. Evid. Based Complement. Alternat. Med. 2011, 2011, 1–15. DOI: 10.1093/ecam/nep067.
- Shen, L.H.; Wang, J.S.; Li, Y.; Zhang, D.H.; Zhu, T.C. Research and Application of Plant Polysaccharide. Zhongguo Nong Xue Tong Bao. 2011, 27, 349–352.
- Li, H.;, (2013) Study on effect of polysaccharides from Paenonia sinjiangensis K.Y.Pan on oral cariogenic bacterial virulences in vitro[master’s thesis]. [China]: Xinjiang Medical University
- Yu, Z. H.; Li, H. Y.; Qian, Y., and Yan, L. Effect of Lentinus Edodes Polysaccharide on Oxidative Stress, Immunity Activity and Oral Ulceration of Rats Stimulated by Phenol. Carbohydr. Polym. 2009, 75, 115–118. DOI: 10.1016/j.carbpol.2008.07.002.
- Bhalang, K.; Thunyakitpisal, P.; Rungsirisatean, N. Acemannan, a Polysaccharide Extracted from Aloe Vera, Is Effective in the Treatment of Oral Aphthous Ulceration. J. Altern. Complement. Med. 2013, 19, 429–434. DOI: 10.1089/acm.2012.0164.
- Hu, S.; Wang, D.; Zhang, J.; Du, M.; Cheng, Y.; Liu, Y.; Zhang, N.; Wang, D., and Wu, Y. Mitochondria Related Pathway Is Essential for Polysaccharides Purified from Sparassis Crispa Mediated Neuro-protection against Glutamate-induced Toxicity in Differentiated PC12 Cells. Int. J. Mol. Sci. 2016, 17, 133. DOI: 10.3390/ijms17020133.
- Hao, Z.Q.; Wang, R.R.; Feng, C.P.; Chang, M.C.; Meng, J.L.; Liu, J.Y.; Cheng, H.Y. Research of Sparassis Crispa Polysaccharide and Its Function. Shi Yong Jun. 2017, 36, 48–51.
- Kim, H. H.; Lee, S., and Singh, T. S. Choi, J.K.; Shin, T.Y.; Kim, S.H. Sparassis Crispa Suppresses Mast Cell-mediated Allergic Inflammation: Role of Calcium, Mitogen-activated Protein Kinase and Nuclear factor-κB. Int. J. Mol. Sci. 2012, 30, 344–350. DOI: 10.3892/ijmm.2012.1000.
- Choi, W. S.; Shin, P. G.; Bok, Y. Y.; Jun, N. H., and Kim, G.-D. Anti-inflammatory Effects of Sparassis Crispa Extracts. J. of Mushroom. 2013, 11, 46–51. doi:10.14480/JM.2013.11.1.046.
- Hao, Z.; Chen, Z.; Chang, M.; Men, J.L.; Liu, J.Y., and Feng, C.P. Rheological Properties and Gel Characteristics of Polysaccharides from Fruit-bodies of Sparassis Crispa. Int. J. Food Prop. 2018, 21, 2283–2295. DOI: 10.1080/10942912.2018.1510838.
- Shi, L. 2016 . Bioactivities, Isolation and Purification Methods of Polysaccharides from Natural Products: A Review. Int. J. Biol. Macromol. 92, 37–48. 10.1016/j.ijbiomac.2016.06.100.
- Men, J.L.; Feng, C.P.; Chang, M.C.; Cheng, H.Y. Antimicrobial Characteristics of Polysaccharide from Ganoderma Lentinan. J. of Shanxi Agricultural Univ. (Natural Science Edition). 2012, 32, 261–264.
- Funami, T.; Nakauma, M. Instrumental Characteristics from Extensional Rheology and Tribology of Polysaccharide Solutions. J. Texture Stud. 2021, 52, 567–577. DOI: 10.1111/jtxs.12638.
- Hasan, I.; Gerdol, M.; Fujii, Y.; Rajia, S.; Koide, Y.; Yamamoto, D.; Kawsar, S., and Ozeki, Y. cDNA and Gene Structure of MytiLec-1, a Bacteriostatic R-type Lectin from the Mediterranean Mussel (Mytilus Galloprovincialis. Mar. Drugs. 2016, 14(5), 92. DOI: 10.3390/md14050092.
- Sanpinit, S.; Moosigapong, K.; Jarukitsakul, S.; Issuriya, A.; Joycharat, N.; Maneenoon, K.; Jaisamut, P.; Chusri, S.; Voravuthikunchai, S.P.; Jetwanna, K.W.; Limsuwan, L.S. 2022 , et al. Selected Thai Traditional Polyherbal Medicines Suppress the Cariogenic Properties of Streptococcus Mutans by Disrupting Its Acid Formation and Quorum Sensing Abilities. S. Afr. J. Bot. 144, 355–63. 10.1016/j.sajb.2021.09.014.
- Tada, R.; Harada, T.; Nagi-Miura, N.; Adachi, Y.; Nakajima, M.; Yadomae, T., and Ohno, N. NMR Characterization of the Structure of a β-(1→3)-d-glucan Isolate from Cultured Fruit Bodies of Sparassis Crispa. Carbohydrate Research.2007, 342(17), 2611–2618. DOI: 10.1016/j.carres.2007.08.016.
- Yu, Y.; Ma, J.; Wang, H. Advances in Fatty Acid Biosynthetic Diversity in Bacteria. J. of Microbiol.(China). 2016, 36, 76–83.
- Liu, Y.; Zhong, Y.; Jiang, L. Research and Prospects of the Microorganisms Producing Polyunsaturated Fatty Acids. Genomics Appl Biol. 2018, 37, 4380–4390.
- Dantas, G.; Sommer, M. O.; Oluwasegun, R. D., and Church, G. M. Bacteria Subsisting on Antibiotics. Science 2008, 320, 100–103. DOI: 10.1126/science.1155157.
- Hamad, B. The Antibiotics Market. Nat. Rev. Drug Discov. 2010, 9, 675. DOI: 10.1038/nrd3267.
- Lu, C. D. Pathways and Regulation of Bacterial Arginine Metabolism and Perspectives for Obtaining Arginine Overproducing Strains. Appl. Microbiol. Biotechnol. 2006, 70, 261–272. DOI: 10.1007/s00253-005-0308-z.
- Sun, X.Z.; Zhao, D.Y.; Zhou, Y.C.; Wang, Q.Q.; Qin, G., and Yao, S.K. Alteration of Fecal Tryptophan Metabolism Correlates with Shifted Microbiota and May Be Involved in Pathogenesis of Colorectal Cancer. World J. Gastroenterol. 2020, 26, 7173. DOI: 10.3748/wjg.v26.i45.7173.
- Rodionov, D. A.; Vitreschak, A. G.; Mironov, A. A., and Gelfand, M. S. Regulation of Lysine Biosynthesis and Transport Genes in Bacteria: Yet Another RNA Riboswitch. Nucleic Acids Res. 2003, 31, 6748–6757. DOI: 10.1093/nar/gkg900.
- Lei, L.; Zeng, J.; Wang, L.; Gong, T.; Zheng, X.; Qiu, W.; Zhang, R.; Yun, L.; Yang, Y.; Li, Y., et al. Quantitative Acetylome Analysis Reveals Involvement of Glucosyltransferase Acetylation in Streptococcus Mutans Biofilm Formation. Environ. Microbiol. Rep. 2021, 13, 86–97. DOI: 10.1111/1758-2229.12907.
- Hayes, M. L.; Berkovitz, B. K. The Reduction of Fissure Caries in Wistar Rats by a Soluble Salt of Nonanoic Acid. Arch. Oral. Biol. 1979, 24, 663–666. DOI: 10.1016/0003-9969(79)90115-8.
- Kabara, J. J.; Swieczkowski, D. M.; Conley, A. J., and Truant, J. P. Fatty Acids and Derivatives as Antimicrobial Agents. Antimicrobial Agents and Chemotherapy.1972, 2(1), 23–28. DOI: 10.1128/aac.2.1.23.
- Nalina, T.; Rahim, Z. H. A. The Crude Aqueous Extract of Piper Betle L. And Its Antibacterial Effect Towards Streptococcus Mutans. Am J Biotechnol Biochem. 2007, 3, 10–15. DOI: 10.3844/ajbbsp.2007.10.15.
- Naidu, A. S.;, Ed. Natural Food Antimicrobial Systems; America: CRC press, 2000.
- Somers, E.; Ptacek, D.; Gysegom, P.; Srinivasan, M., and Vanderleyden, J. Azospirillum Brasilense Produces the Auxin-Like Phenylacetic Acid by Using the Key Enzyme for Indole-3-Acetic Acid Biosynthesis. Appl. Environ. Microbiol. 2005, 71, 1803–1810. DOI: 10.1128/AEM.71.4.1803-1810.2005.
- Huang, H.;, (2018) Inhibitory effect of Bacillus subtilis on cariogenic bacteria by metabolomics approaches[master’s thesis]. [China]: Lanzhou University.