ABSTRACT
Essential fatty acids play an important role in promoting brain development, decreasing the incidence of cardiovascular diseases, and reducing inflammation. However, they are inherently unstable and susceptible to oxidative deterioration due to two or more double bonds in their structure. Delivery systems have been developed to provide effective encapsulation and protection for sensitive fatty acids, thus fulfilling their health benefits. Emulsion-based microencapsulation is one of the most promising techniques for the delivery of omega 3 & 6 fatty acids. The emulsion composition and structure, as well as the storage conditions are regarded as key factors to influence the shelf life and stability of emulsions. To maximize the resistance of these fatty acids in emulsions against oxidation, emulsion structure design was particularly highlighted, and different methods for tailoring emulsion structure were developed. The current work was focused on the careful design of emulsion structure to improve the oxidative stability and shelf life of fatty acids (FAs). Different types of carrier systems, formulations, including conventional emulsions, multilayer emulsions, gelled emulsions, pickering emulsions, and structures designs are discussed, and their protective effect for FAs are with regard to food fortification is also conferred, particularly the role of interfacial structure in emulsions is emphasized. The effects of emulsifiers and its modification methods on the interfacial structure are presented to improve further the stability of FAs during storage.
Introduction
Omega 3 and 6 fatty acids contain multiple double bonds in their carbon chains[Citation1];that are most commonly present in plants and animals, such as in oils and lipids like linseed oil, algal oil, fish oil, groundnut oil, olive oil, flax oil respectively,[Citation2] which have been proved to play an important role in promoting the brain development and cognitive balances,[Citation3] reducing the incidence of cardiovascular diseases,[Citation4] and controlling inflammation,[Citation5,Citation6] suppressing tumor carcinogenesis.[Citation7,Citation8] However, they are sensitive to oxidative degradation during processing due to a high degree of unsaturation, which potentially reduce the nutritional quality and destroy the sensory characteristics of the foods.[Citation9] For example, because of a high content of PUFA (polyunsaturated fatty acids) and MUFA (monounsaturated fatty acids), sunflower oil, groundnut oil, fish oil produce rancid odors due to the oxidative deterioration[Citation10,Citation11] Fish oil decomposed to volatile aldehydes, ketones, and alcohols due to the oxidation, in which hexanal, 2,4-heptadienal, 4-heptenal, (Z)-4-heptanal, and (E,E)-2,4-heptadienal at low odor thresholds affected the sensory quality of the products.[Citation12,Citation13] Therefore, different strategies have been developed to minimize omega 3/6 fatty acid oxidation, mainly including the addition of antioxidants either synthetic or natural or semi-synthetic[Citation14] microencapsulation[Citation15–17] and incorporation into emulsion formulation,[Citation9] till the most recent technique of microencapsulation with a modified layering structure.[Citation18]
Butylated hydroxytoluene, butylated hydroxy anisole, tert-butylhydroquinone, and α-tocopherol, are the most commonly used antioxidants in the food industry to prevent the oxidation of omega 3 fats.[Citation19] However, these antioxidants are gradually falling out of the favor of the consumers due to their synthetic nature and side effects on health on a long run.[Citation20] When encapsulation can effectively protect fatty acids (FAs) from being oxidized, usage of natural starches like gum arabic, modified starches, modified chitosan, and pectin have been frequently applied as wall materials to encapsulate these fatty acids as shown in SEM of multi-layered microencapsulation structure of antioxidants of plant-based polyphenols (AOX) and wall material (WM).[Citation15,Citation16,Citation18] Besides, complex wall materials exhibit a great reduction in PUFAs, MUFAs oxidation process. When pea protein isolate – modified starch complexes are used to encapsulate docosahexaenoic acid (DHA)-rich canola oil, the peroxide value (PV) of the microcapsules decreased by over 60% compared to bulk oil.[Citation17] Thus, incorporation of highly sensitive fatty carboxylic acid into oil-in-water (O/W) emulsions is additionally a wide used approach to limit the oxidation of PUFAs and MUFAs with advantage of the benefit of preparation and low value[Citation20–23] also claiming the slow disintegration with respect to time, temperature, and acids.[Citation18]
Figure 1. SEM of multi-layered microencapsulation structure of antioxidants (AOX) and wall material (WM).
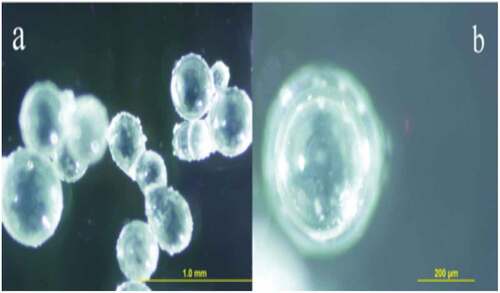
The carrier structure like the unsaturation degree of oil is merited by the consistency of the wall material, yet because the thickness and also the charge of oil/water interface, considerably influence the oxidation rate of PUFAs and MUFAs in emulsions.Based on the modified emulsion structure of the matrix and wall material,[Citation18] relates to method of preparing multi-layered microencapsulation of fatty acids by incorporating multi-layered anti-oxidant to increase the shelf life of edible oil () comprising the steps of: a) mixing antioxidant core materials and wall materials composition in the corresponding ratio of (1:1.5, 2.5:1.25, l:lv/v), respectively; ) concentrating the obtained mixture up to 9% solid content with a thickness in between 10 and 40 urn each layer; c) dispersing the obtained mixture in water under a heated magnetic agitation at 50–95°C for 3–7 minutes; d) incorporating multi-layered anti-oxidant with edible lipids by spray drying by maintaining temperature in between 100°C and 160°C or by covalent bonding as depicted in Multilayering Coating ratios – antioxidants (AOX) of natural polyphenols extracted from herbs, spices, and wall material (WM) was performed as stated.[Citation18]
Figure 3. Schematic illustration of different types of emulsions: conventional emulsions (a), multilayer emulsions (b), gelled emulsions (c), pickering emulsions (d), and multi-layered microencapsulation structure (e).

Regarding oil phase, the susceptibility of PUFAs and MUFAs to oxidation depends on the bis-allylic C-H bonds in their carbon chain, and hence the rate of lipid oxidation is affected by the lipid unsaturation.[Citation1,Citation24] Oxidative stability of PUFAs and MUFAs in emulsions is also influenced by the viscosity,[Citation25] pH,[Citation26] and ionic strength[Citation26–28]) of the mixture phase. The effect of both aqueous phase and oil phase on PUFAs and MUFAs protection is linked to the structure of oil/water interface where the oxidation of PUFAs and MUFAs is initiated. During lipid oxidation process, amphiphilic lipid hydrogen peroxide aggregates at the interface and reacts with the pro-oxidants in the aqueous phase.[Citation21,Citation29] The free radicals generated by the reaction penetrate through the interface layer initiate the free radical-chain reaction with PUFAs and MUFAs in the oil droplets, thus accelerating the oxidation. Therefore, the thickness, charge, and composition of the interface area unit thought of to be the foremost necessary factors touching the oxidation rate of these fatty acids.[Citation30,Citation31] A large number of studies have demonstrated the successful limitation of PUFAs and MUFAs oxidation by the strategy of emulsion structure and carrier design.[Citation1,Citation32,Citation33]
This review aimed to summarize the recent practice of emulsion – carrier structure design to improve the oxidative stability of FAs through microencapsulation technique. The following two aspects are covered: (a) relationship between emulsion type and lipid oxidative stability, and (b) interfacial structure design for improvement of the FAs oxidative stability. In particular, the feasibility and superiority of the emulsion-carrier structure design is discussed.
Carrier structure for micrpencapsulation type for oxidative stability
The type of carrier systems significantly influences the oxidative stability of PUFAs and MUFAs[Citation34–36] In this section, various types of emulsions structures that are commonly used for PUFAs and MUFAs stabilization are discussed, including conventional emulsions, multilayer emulsions, gelled emulsions, pickering emulsions, and multi-layered emulsion type ().
Conventional carrier systems
Conventional O/W carriers structures contain an oil phase dispersed in an aqueous phase, which are usually stabilized with one layer of emulsifiers[Citation1 . Compared with the bulk oil, conventional emulsions due to the protective effect of interfacial layer formed by some emulsifiers could effectively inhibit the lipid oxidation[Citation23] . Emulsifiers such as proteins, polysaccharides, and phospholipids are widely used to stabilize emulsions and exhibit good protection of fatty acids[Citation37] .
Principally because of the power of proteins to create a physical barrier, which may separate lipids from alternative reactive species.[Citation38] the main oxidation pathway in emulsions is that the metal-catalyzed decomposition of supermolecule hydroperoxides.[Citation21] Proteins within the liquid part are often used because the chelating agents and bind pro-oxidative transition metals, and hinder their access to the supermolecule substrate and inhibit oxidation.[Citation39] Additionally, some proteins also can inhibit supermolecule oxidation by scavenging free radicals, and therefore the antioxidation capability varies with proteins because of completely different aminoalkanoic acid compositions, sequences, and abstraction arrangements. As an example, whey macromolecule,[Citation40] soy macromolecule,[Citation41] egg macromolecule,[Citation42] and casein[Citation43] square measure are able to improve the aerophilic stability of omega-three fatty acids once acting as emulsifiers. The emulsion stabilized with whey macromolecule showed a lower PUFAs and MUFAs oxidation rate as compared with the casein-stabilized emulsion at pH scale seven 7.0.[Citation44] The attainable clarification was that the phosphate teams carried by the casein molecules showed the next affinity with pro-oxidants (e.g., iron) than the process teams on alternative proteins, that semiconductor diode to AN aggregation of pro-oxidants at the interface and therefore accelerated the oxidation.[Citation45]
Polysaccharides containing both nonpolar and polar groups can adsorb to the surfaces of oil droplets and are able to increase the oxidative stability of PUFAs and MUFAs.[Citation46] For example, adding cellulose, stabilized emulsions effectively restrained the oxidation of animal oil.[Citation47] It is because the metal-chelating ability of the pectin decreases the concentration of pro-oxidants in the continuous phase.[Citation18,Citation48] Similar results were reported for Tween 80 – stabilized emulsions containing metallic element alginate within the continuous part.[Citation49] As a rule of thumb, if anionic polysaccharides square measure free in a binary compound part, the interaction between emulsifiers and pro-oxidants could bring pro-oxidants far from the interface of the emulsion and therefore will increase the aerophilous stability of PUFAs and MUFAs in emulsions[Citation47,Citation49] ion polysaccharides protect macromolecule against reaction in an exceedingly completely different mechanism, as an example, chitosan participates within the formation of a charged surface layer that repels ion transition metal ions far from the interface.[Citation50,Citation51]
Phospholipids extracted from both animal and plant sources show excellent emulsifying capability and attract much attention due to their antioxidant activity.[Citation52] Because phospholipids are amphiphilic molecules, so they can adsorb to oil–water interfaces and stabilize lipid droplets, and blend well.[Citation53] Addition of soybean lecithin into oil emulsions was reported to improve the stability of emulsions by decelerating the iron-catalyzed lipid oxidation.[Citation54] Similar results were ascertained in oilseed lecithin-stabilized animal oil emulsions.[Citation52] Besides, compared to proteins, phospholipids are evidenced to stop PUFAs against oxidation effectively. The animal oil emulsions stable with less phospholipid had a lower concentration of Thiobarbituric acid reactants (TBARS) (2.1 µmol/L) than that stable with whey supermolecule isolate (WPI) (3.5 μmol/L) upon 48-days storage.[Citation55] In general, phospholipids area unit thought of to be capable of chelating transition metals by phosphate teams[Citation56] and act as surface barrier against peroxyl radicals,[Citation57] it’s conjointly believed that amino phospholipids may participate within the Maillard reaction with lipid oxidation product, resulting in the resulted product with increased antioxidation ability.[Citation58] Generally, conventional emulsions are ease of preparation due to their simple structure. Their resistance against oxidation largely depends on emulsifiers used. However, these emulsions are usually sensitive to environments, such as pH, ion strength, and temperature during storage because of the single layer of emulsifier. These factors potentially decrease the physical stability of emulsions, and thus reduce the chemical stability of fatty acids in emulsions. Therefore, Omega 3 fatty acids in conventional emulsions still undergo oxidation.[Citation59] To further improve the oxidative stability of PUFAs and MUFAs in emulsions, additional strategies have to be developed with the objective of obtaining products with a long-term physical and chemical stability; when applied in food ingredients, enzymes, cells, or other materials.
Classical layered emulsion system
Here, multilayer emulsions are characterized with droplets coated by quite one layer of emulsifiers.[Citation60] They’re ready by the static deposition technique, during which associate degree oppositely charged electrolyte layer directly adsorbs to the surface of a preexisting layer of emulsifiers.[Citation60] A second wetting agent adsorbable to the surface of monolayer emulsions results in the fabrication of bilayer emulsions. Similarly, tri-layer emulsions are ready by the sorption of third wetting agent to the surface of bilayer emulsions.
FAs in bilayer emulsions show higher reaction stability than those in typical emulsions with single surface layer.[Citation60] Like, chia oil bilayer emulsions stable with phospholipid and chitosan exhibited lower PV (<1 meq hydroperoxides/kg oil) than the mono-layer emulsions (3 to 6 meq hydroperoxides/kg oil) and chia bulk oil (<2 meq hydroperoxides/kg oil).[Citation59] The linseed oil in sodium caseinate/pectin-stabilized bilayer emulsions were more stable against oxidation compared to the oil in sodium caseinate-stabilized monolayer emulsions.[Citation61] The enhanced aerophilic stability in bilayer emulsions could also be as a result of the usage of multiple emulsifiers has the synergistic impact in reinforcing the antioxidative capabilities. Besides, it’s additionally attributed to the actual fact that multilayer emulsions have a thick interface layer that may defend PUFAs against the liquid pro-oxidant species because of its “barrier” impact.[Citation62] As an example, a thicker surface layer of the bilayer emulsions acts as a physical barrier separating PUFAs from pro-oxidants in liquid part and therefore contributes to the limitation of PUFAs oxidation,[Citation1] except for dispersion systems, the physical barrier of thick interface layer is contributing to scale back the oxidation rate of microcapsules once the emulsions square measure dried into powders. Bilayer microcapsules were fabricated by spray-drying from the bilayer emulsions stabilized by pectin and whey protein hydrolyzate, whose hydroperoxide content reduced by over 60% than for the monolayer microcapsules after 12 weeks.[Citation63] The bilayer microcapsules spray dried from lecithin/chitosan-stabilized emulsions provided a more robust protecting impact against animal oil oxidation compared to the monolayer microcapsules throughout storage at totally different temperatures.[Citation64] It had been steered that thick wall of the bilayer emulsions might stop external pro- oxidants from reaching the PUFAs in microcapsules core.
It is noteworthy that lipid oxidation can also be inhibited by the formation of a positively charged interface that can repel cationic metal ions.[Citation21] For example, droplets coated by proteins are extremely positive underneath the isoelectric purpose of proteins, therefore, charged emulsifying proteins because of the static repulsion will keep metals aloof from the interface and defend the FAs in emulsions against oxidation.[Citation38]
Emulsions with three layers of emulsifiers are usually developed with a shot to any improve the aerophilous stability of FAs.[Citation65] The ready tri-layer animal oil emulsions stable with Tween twenty, chitosan, and pectin. The results demonstrated that the oxidation stability of animal oil in emulsions redoubled with the redoubled range of surface layers. Nevertheless, not all tri-layer emulsions obey similar laws. Compared with the bilayer emulsion stabilized with chitosan, the tri-layer emulsion stabilized with chitosan/alginate accelerated the PUFAs oxidation rate during storage, but showed better oxidative stability than the monolayer emulsion with chitosan/alginate.[Citation66] The actual fact that the charged emulsion droplets coated by chitosan/alginate electrostatically repelled pro-oxidative transition metals. Also, the surface assimilation order of wetting agent into interface effects the chemical reaction stability of PUFAs. It had been shown that emulsions with inner WPI and outer fish gelatin (FG) layers exhibited higher chemical reaction stability (0.34 ppm of hexanal) than that with layers of opposite surface assimilation order (0.5 ppm of hexanal) upon 21-days storage. To a stronger surface activity of WPI, resulting in the next interface surface assimilation than FG. In addition, positive charges of outer FG layer electrostatically repelled the transition metals. Hence, both charge and interfacial thickness need to be taken into account when multilayer emulsions are designed to prevent PUFAs against oxidation.
Gelled emulsions
Gelled emulsions carries with it blended oil droplets entrapped at intervals in a gel matrix. This can be acheived either by adding thickeners into the primary emulsions directly or promoting the cross-linking of biopolymers (such as proteins and polysaccharides) within the emulsions.[Citation25,Citation67] Current researches specialize in the employment of gelled emulsions as food-grade delivery systems for Fas.[Citation68]
Compared with non-gelled linseed oil nano-emulsions, the PV of gelled nano-emulsions with carbomer polymer as a thickener was significantly reduced by 27% after 12 days of storage at 45°C.[Citation25] Similar results were reported for gelled vegetable oil emulsions stabilized by alginate that showed lower level of hydroperoxides than that in the non-gelled emulsions.[Citation35] The protection of PUFAs in gelled emulsions is owed to the very fact that the accumulated viscousness of binary compound part retards the migration and transfer of the reactive species throughout oxidation.[Citation69] Additionally, gelled emulsions prepared by cross-linking of biopolymers encapsulate FAs and therefore enhance the protection for lipids. By electrostatic complexation of low methoxy cellulose and caseinate at hydrogen ion concentration 4–5, colloidal gel particles containing animal oil were fancied, that reduced PV by nearly ninetieth as compared with standard emulsions.[Citation70] Also, multilayer gelled emulsions are ready to foresee the PUFAs against reaction. The bilayer emulsion with inner-gelatin and outer-alginate layers was atomized into calcium chloride solution to produce a bilayer gelled emulsion.[Citation71] A lower concentration of primary and secondary oxidation products was observed in the bilayer gelled emulsion than in the monolayer emulsion or bulk oil. It was suggested that the gelation of the interface layer formed a barrier for both hampering the contact between PUFAs and MUFAs from the pro-oxidants and retarding the oxygen diffusion. In addition, it’s been established that gelled emulsions maximize the result of antioxidants. Casein is widely used as associate degree inhibitor since it is able to scavenge free radicals and chelate transition metals.[Citation72] Compared with the nano-emulsion stabilized with caseinate, the PV and TBARS of flaxseed oil encapsulated in hydrogel beads containing caseinate reduced by over 75%, which may be attributed to the improved local concentration of caseinate in hydrogel beads.[Citation43]
Pickering emulsions
Pickering emulsions refer to the emulsions stabilized by solid particles instead of surfactants.[Citation73] These solid particles irreversibly absorb to the surface of droplets, reduce the surface tension, and form emulsions with better physical stability.[Citation73] Pickering emulsions also present an excellent protection of PUFAs from chemical degradation as compared with conventional emulsions.[Citation74]
Pickering emulsions stabilized with inorganic silica particles showed better oxidation stability than that stabilized by Tween 20, and reduced the concentration of lipid hydroperoxide by up to 40%.[Citation74] Similar results were discovered for vegetable oil emulsions stable with food-grade particles, crystalline polysaccharide, and changed starch.[Citation75] Also, Pickering emulsions stable by macromolecule droplets will limit the PUFAs oxidation. For instance, the safflower oil emulsion stabilized with milk protein-coated lipid droplets showed much improved oxidation stability than that in the composition-matched conventional protein-stabilized emulsion.[Citation76] In Pickering emulsions, the thick interfacial layer formed by particles acts as a physical barrier. It lessens the interaction between pro-oxidants in aqueous phase and the hydroperoxides at the droplet interface and cuts the rate of radical permeation.[Citation77] Moreover, the presence of particles decreases the contact between aqueous phase and oil phase, thereby effectively reducing the surface area where oxidation reaction occurs.[Citation74]
Compared to particles with the single ingredient, complex particles have shown an improved capability to stabilize Pickering emulsions. Gliadin–chitosan complex exhibited greater wettability and interface adsorption capacity than gliadin.[Citation78] It effectively improved the rigidity or mechanical strength of the Pickering emulsion, resulting in an enhanced oxidative stability of corn oil. Zein–pectin complex particles with better interfacial packing behaviors was applied to prepare Pickering emulsions, which showed a stronger network architecture that further limited the oxidation of PUFAs.[Citation79] The high viscoelasticity of emulsions limits the diffusion and transition of pro-oxidants toward the interface, endowing greater resistance against oxidation.[Citation80] Besides, the antioxidative capability of the complex particles also needs to be taken into account. Compared with silicon oxide, silica-ε-polylysine microparticles quenched additional peroxyl radicals, resulting in a lower permeation rate of radicals within the emulsion.[Citation81] Similar results were determined within the Pickering emulsions stable with starch-polylysine nanoparticles.[Citation82] Advanced particles containing polyphenols have shown glorious protection to PUFAs against oxidation aside from poly(amino acids). The malondialdehyde content of Pickering emulsions stable with gliadin-grape seed proanthocyanins advanced particles was reduced by two hundredth than that in gliadin-stabilized emulsions, exhibiting associate improved aerophilic stability of PUFAs.[Citation79] The polyphenols area unit prone to be delivered and set at the interface, therefore, inhibit PUFAs oxidation through the formation of advanced particles.
However, not the whole surface area of stabilizers could be covered under Pickering-emulsions while it deemed difficult due to the aerophilic degradation of PUFAs; thus, additional food-grade particles instead of inorganic particles ought to be developed for widening their applications within the food business. As the aforesaid descriptions, the oxidation stability of PUFAs differs in numerous forms of emulsion systems. Overall, PUFAs show the enhanced oxidative stability in multilayer emulsions than monolayer emulsions due to the improved antioxidative capabilities by combining different emulsifiers and the increased thickness of interfacial layers. Compared with non-gelled emulsions, the gelled emulsions show a better protection of PUFAs due to the increased viscosity of aqueous phase and the maximized effect of antioxidants. For PUFAs in emulsions containing relatively large oil droplets (>2 μm), pickering emulsions stabilized with colloidal particles may display a better protective effect for PUFAs because irreversibly adsorbed particles not only lead to very stable systems to droplet coalescence, but also benefit the anchoring of antioxidants at the interface.
Interfacial structure design to improve oxidative stability
Different carrier designs with special interfacial structures can significantly inhibit or minimize the oxidation rate of FAs by altering the location and reactivity of pro- oxidative transition metals, lipid hydroperoxide, and free radicals[Citation1] when in contact with physical or chemical triggering factors. Besides, interfacial structure design also plays an important role in preventing the oxidation of FAs. This is in the main done by modifying surface layers that comprises valence and/or noncovalent complexes through chemical, enzymatic, and physical approaches ().
Table 1. Emulsifier modification in different ways to improve the oxidative stability of omega 3 fatty acids.
Covalent complexes
Covalent complexes are prepared by conjugating one biopolymer to another and have shown great techno-functional and biological properties.[Citation83] Through controlling reactants and reaction conditions, food-grade covalent complexes with good antioxidant activity can be produced. Maillard reaction, chemical grafting reaction, and enzymatic reaction are commonly used to form valence complexes.
Maillard reaction: Maillard reaction is a reaction between reducing sugars and amino acids, which is widely used to prepare conjugates. Maillard reaction products (MRPs) showed great capability in metal-chelating, reducing power, and radical scavenging.[Citation84,Citation85] Owing to such properties, they’re useful as they stop unsought oxidation of PUFAs in emulsions whereas acting as emulsifiers and also the adsorbable MRPs at the interface limit radical-chain reaction in initial oxidation stage.[Citation86] By with chemicals linking ribose/fructose and lysine/glycine, the ready MRPs showed the same inhibitor activity to it of butylated radical anisole (one of the artificial antioxidants) and effectively pent-up the oxidation of vegetable oil in emulsions..[Citation87] Hydrolyzed β-lactoglobulin- glucose conjugates exhibited great protection of PUFAs in menhaden O/W emulsions, reducing 80% and 90% of lipid hydroperoxides and propanal compared to the control group after 10 days, respectively.[Citation88]
Besides, the protective effect of MRPs against lipid oxidation also includes their improved antioxidative property, which is influenced by the molecular weight. The PV of fish oil in the caseinate-dextran (100KD) conjugates-stabilized emulsions was significantly lower than that in the emulsions stabilized by caseinate and caseinate-dextran (6KD) conjugates.[Citation89] It was recommended that MRPs with a high mass possessed higher capability in metal-chelating because of their hydroxyl radical or pyrrole teams.
In addition, a protecting impact of MRPs against macromolecule reaction results from the thick and dense surface layer,[Citation90] as an example, in emulsions stable by pea macromolecule isolate-gum Arabic conjugates, the hexanal content was lower, however, macromolecule hydroperoxides showed no vital distinction compared to the management cluster.[Citation91] It had been recommended that surface layer effectively prevented the interaction between hydroperoxides and transition metal in liquid part, and avoided the more increasing content of hexanal. Similar results were additionally discovered in emulsions stable by soy macromolecule isolate-soy soluble carbohydrate conjugates that showed Associate in Nursing enhanced drop size of emulsions because of thicker adsorbable wetter layers.[Citation92] Moreover, conjugation enhances the sorption potency of conjugates to the interface and will increase the cohesion of surface layer.[Citation93] The advantages of MRPs are exhibited even the conjugate-stable emulsions were dried into powders. It was suggested that the MRPs offered higher encapsulation efficiency for PUFAs, reducing the surface oil, and lead to better protection of PUFAs from oxidation in the micro-capsules.[Citation94,Citation95]
Free radical grafting reaction: Free radical grafting reaction is widely used to develop protein–polyphenol or polysaccharide–polyphenol conjugates.[Citation42,Citation96] Through redox reactions, hydroxyl radicals are formed and react with specific residues on the protein or polysaccharide chain to produce free radicals. The reaction between free radicals and polyphenols leads to the formation of conjugates.[Citation83] The free radical grafting reaction improves the amount of phenolic hydroxyl groups of conjugates and enhances their free radical scavenging abilities and reducing abilities.[Citation97] Besides, a reduced interfacial tension of conjugates results in stronger interfacial adsorption so that the polyphenols have better antioxidant activities.[Citation60]
The protein–polyphenol conjugates exhibited better antioxidative activity compared with the protein alone. The covalent attachment of catechin to egg white protein showed better antioxidant capacity in both in vitro models and linoleic acid O/W emulsion.[Citation42] Stronger oxygen radical scavenging capacity and reducing power was observed for epigallocatechin gallate-WPI conjugates, which were used to stabilize menhaden oil emulsion and exhibited a lower PV and TBARS during the storage.[Citation98] By using egg albumin-catechin conjugates as emulsifiers, the concentration of primary and secondary oxidation products in fish O/W emulsions was significantly reduced by 30% to 65% and 40% to 80% compared with the control group, respectively.[Citation99] Polysaccharide–polyphenol conjugates also function in averting emulsions against oxidation. The inhibition percentage of lipidic peroxidation in the emulsion stabilized by gallic acid–chitosan and catechin–chitosan conjugates rose more than threefold compared with that stabilized by unmodified chitosan.[Citation96]
Enzymatic cross-linking: Enzymatic cross-linking promotes the formation of intramolecular and intermolecular bonds between biopolymers.[Citation100] It is an effective approach to produce conjugates that could inhibit lipid oxidation and improve the stability of PUFAs in emulsions during storage.[Citation101,Citation102]
Transglutaminases crosslink proteins through three different paths that include acyl transfer reaction, deamidation reaction, and Gln-Lys residues cross-linking reaction.[Citation100] But the cross-linking before or once emulsification affects the reaction rate of PUFAs in emulsions. Once metallic element caseinate-stabilized animal oil emulsions were treated by transglutaminases, no improved, or attenuate aerobic stability was ascertained compared with the untreated emulsions.[Citation101] However, the flaxseed oil emulsion stabilized by cross-linked sodium caseinate exhibited better oxidative stability with reduced formation of hydroperoxides and volatiles.[Citation102] Similarly, the hexanal content of rapeseed oil emulsions decreased by more than 40% when cross-linked bean protein isolate was used as an emulsifier.[Citation103] Furthermore, the emulsion stabilized by cross-linked Na caseinate exhibited higher protection of animal oil despite a discount discovered for the inhibitor property of treated Na caseinate.[Citation104] These studies ensure that cross-linking by transglutaminases before emulsion formation ends up in higher resistance of emulsions against chemical reaction. This can be attributed to the cross-linking between free proteins or adsorbate proteins resulting in completely different interface structure and barrier property.[Citation101] For cross-linked proteins, they have a tendency to create denser surface layer that gives extra resistance to the diffusion of O from the continue part to the oil part.
Laccase can oxidize various substrates that have a p-diphenol structure, such as polyphenols, methoxy-substituted phenols, anilines, and diamines.[Citation105,Citation106] It has been widely used for emulsifier modification and the resulted conjugates exhibit enhanced antioxidative ability due to increased amount of phenolic hydroxyl. An improved antioxidation capability was observed for laccase-catalyzed polymerized catechin.[Citation107] Similarly, gelatin–catechin conjugates synthesized showed smart scavenging activity against anion radicals.[Citation108] The content of oxidation merchandise within the emulsion stable with rice protein–ferulic acid conjugates was reduced by over five hundredth from that stable with rice protein – ferulic acid physical mixture.[Citation109] It should be as a result of the cross-linking promoted the targeted polyphenol to accumulate at the droplets interface and therefore protected the lipoid from oxidation.
Genipin-induced cross-linking strategy: Genipin could be a nature cross-linking agent that acts with primary alkane series teams (such as essential amino acid and arginine) of proteins to make building block and building block valency bonds.[Citation110] Genipin-mediated wetting agent modification helps to enhance the chemical science stability of emulsions. When surface cross-linking by genipin, increased aerophilous stability of animal oil was determined for the emulsion stabilized with WPI or WPI-epigallocatechin gallate.[Citation98] Cross-linking of surface macromolecule could promote macromolecule aggregation, resulting in a dense and thick-surface coating that hinder the lipoid reaction by retarding the permeation of pro-oxidants and free radicals.[Citation98]
Multi-layered microencapsulation: In recent years, several scientific studies have investigated the use of multi-layer emulsions for stabilizing oil-in-water emulsions. Multi-layer emulsions are composed of small oil droplets dispersed in an aqueous medium, and each oil droplet is surrounded by a multi-layered membrane generally composed of an emulsifier and a charged biopolymer. Pea proteins and pectin are electrically charged biopolymers, where oil-in-water emulsions were created using three flavor compounds: ethyl acetate (EA), ethyl butyrate (EB), and ethyl hexanoate, one with and one without pectin. Two samples of the oil-in-water emulsions, one with and one without pectin, were then fried in a spray-drier. The results showed that when pectin was used as an additional oil droplet coating, flavor retention increased significantly, and that the beta-sheet structure was protected by the presence of pectin.[Citation111]
In regard to the surface dispersion and charge ratio, the mutual arrangement of polyelectrolytes of multilayer polyelectrolyte microcapsules (with layers – [PAH/PSS]3PAH) by determination of the dissociation level of polyallylamine (PAH) from the surface of a polyelectrolyte microcapsules (PMC) of various types was studied[Citation112]: PMC with a dissolved CaCO3 core after preparation, PMC with an undissolved CaCO3core and PMC with an encapsulated protein. The study concluded that the polyelectrolyte layers are mixed in the entire shell of the capsules with a dissolved CaCO3core. In the case of the PMC with an undissolved CaCO3 core, such mixing of polyelectrolyte layers does not occur. That fact allows us to conclude that the mixing of polyelectrolytes layers mixing at the stage of dissolution of CaCO3core. The PMC with encapsulated protein has partial mixing of polyelectrolytes layers. That phenomenon may be due to the fact that seven-layered protein-containing microcapsules already have a dense and well-formed shell. Also, it has been studied that the surface charge of the PMC does not depend on the charge of the polymer forming the outer layer of the capsule.
Where, current carrier system designs face the trade-off among several major considerations: mechanical stability, complete encapsulation and selective permeability, compatible designs, and suitable extra-cellular microenvironment for optimal cellular functions. Enhancement of one consideration may sacrifice the other. Thus, multi-layered microencapsulation was found to increase the retention of volatile flavors, sensitive compounds during spray drying, thus improving the chemical stability and provides controlled-sustainable release.[Citation18] In the case of capsules filled with an inter-polyelectrolyte complex, an approximately equal amount of the released polymer of each labeled layer was observed. Relates to technique of multi-layered microencapsulation (MM) of edible lipids by incorporating multi-layered anti-oxidant with edible lipids to extend the period of edible oil comprising the steps of: a) combination inhibitor (AOX) core materials and wall materials (WM) composition within the quantitative relation of (1:1.5 (AOX 1:WM I), 2.5:1.25 (AOX 2: WM 2), l:l(AOX + WM 3) v/v); b) concentrating the obtained mixture with an optimized thickness for every layer; c) dispersing the obtained mixture in water underneath a heated magnetic agitation at 50–95°C for 3–7 minutes; c) incorporating multi-layered anti-oxidant with edible lipids by spray drying by maintaining temperature in between 100°C and 160°C.[Citation18]
Interfacial polymerization of microcapsules: Polymeric researchers desire sturdily to reduce dependency on fossil or petroleum feed stock by utilizing renewable source-based polymeric resins in coating. Like, the attempt made to develop neem oil (a renewable source) two-pack self-healing anticorrosive polyurethane (PU) coatings cured at room temperature. The oil-based polyesteramide was prepared in two steps; viz., in the first step, oil fatty amide was synthesized, and in the second, it was directly converted to polyesteramide by condensing amide with phthalic anhydride. Polyesteramide further was utilized for preparation of self-healing PU coatings with incorporation of polyamidoamine-based polyurea microcapsules containing natural self-healing agent. The obtained coatings were tested for thermal, physicochemical, and corrosion resistance tests.[Citation113]
Yet another research sums up the novel chemistry that has been developed to synthesize polyurea microcapsules containing solvent and linseed oil as the active healing agent by interfacial polymerization of commercial methylene diphenyl diisocyanate (MDI) and dendritic 0.0 G PAMAM capable of cross-linking to form a shell material; the thermal stability of the microcapsules showed that prepared microcapsules had excellent stability up to 380 °C. The idea and approach presented in this work have the potential to fabricate microcapsules that could provide better anticorrosive and mechanical properties to coating composites.[Citation113]
Nanostructures: While, the preparation of microcapsules composed of natural materials have received great attention, as they represent promising systems for the fabrication of micro-containers for controlled loading and release of active compounds, and for other applications. Where using polysaccharides as the main materials is receiving great interest, as they constitute the main components of the plant cell wall, which represent an ideal platform to mimic for creating biocompatible systems with varied responsive properties. Several researchers have described methods for the preparation of microcapsules with various sizes and properties using cell wall polysaccharide nanomaterials. Researchers have focused mostly on the works where polysaccharide nanomaterials were used as the main structural components. Where, Omega-3 polyunsaturated fatty acids (ω-3 PUFAs) exert structuring effects on the cellular membrane organization, regulate the gene expression, and modulate various signaling cascades and metabolic processes; thus, the liquid crystalline structures formed upon mixing of eicosapentaenoic acid (EPA, 20:5) with the lyotropic nonlamellar lipid monoolein and the formation of multicompartment assemblies were stabilized by PEGylation of the lipid/water interfaces using a phospholipid with a poly(ethylene glycol) chain. It showed that PUFA influences the phase type and the sizes of the aqueous compartments of the liquid crystalline carriers. The resulting multicompartment systems and stealth nano-sponges may serve as mesoporous reservoirs for co-encapsulation of ω-3 PUFA (e.g., EPA) with water-insoluble drugs and hydrophilic macromolecules toward development of combination treatment strategies.[Citation114]
Coming to the green compositions and processes for fabrication of dual- and multiloaded nanocarriers with an antioxidant functionality, the co-encapsulation of curcumin (studied as a key multipurpose phytochemical antioxidant) with ω-3 polyunsaturated fatty acids (PUFAs) (studied as active ingredients of natural fish oil) may increase the oxidative stability of self-assembled formulations aiming at the development of “food drugs.” The temperature effect examined at 22 and 5°C with regard to preparation conditions and mesophase stability on storage. New knowledge about the controlled multicomponent supramolecular assembly and the achieved stabilization of low-temperature cubic should facilitate the development of cost-effective, stable, and safe delivery systems of weakly soluble natural antioxidant compounds co-encapsulated with ω-3 PUFA oils.[Citation75]
Other methods
High-pressure processing is another operative way to modify interfacial layer and improve the resistance of PUFAs against oxidation.[Citation115,Citation116] After 10 days storage at 50 ◦C, lipid hydroperoxides in untreated gliadin particles-stabilized emulsions were around three times as much as that in 120 MPa micro-fluidization treated emulsions.[Citation97] Similar results were determined for emulsions stabilized with metal caseinate so processed by high blend.[Citation104] High unfolds the di-sulfide bond within the proteins and ends up in the formation of building block di-sulfide bonds.[Citation117] This irreversible structure amendment happens between not solely adsorbable proteins, however, conjointly free proteins, and conduces to make a lot of rigid interface layer so it should effectively shield PUFAs from reaction.[Citation97,Citation115,Citation116] Some attempts to use of covalent bonds to increase structure stability have been also presented, but the resulting microcapsules were mostly composed by synthetic materials, and the development of more green pathways is required to prepare systems that could be exploited in delivery of active compounds.
Noncovalent complexes
The interactions between biopolymers offer opportunities for surface structure modifications. Physical complexation of biopolymers happens through electrostatic interactions, hydrophobic interactions, and hydro-info bonds. These noncovalent complexes is fashioned when or before emulsion preparation, and do facilitate in inhibiting PUFAs reaction in emulsions.[Citation118] The previous is invented in the main through static deposition, that is wide utilized in preparation of multilayer emulsions.[Citation60] The latter is especially mentioned during this section.
Intramolecular soluble complexes of WPI and Gum Arabic (GA) at pH 0.5 were applied to stabilize conjugated polyunsaturated fatty acid emulsion that exhibited a considerably lower element consumption than that stabilized with WPI or GA alone, decreasing from one.85 μmol/(mL⋅min⋅μm), 0.35 μmol/(mL⋅min⋅μm) to zero.05 μmol/(mL⋅min⋅μm).[Citation119] The insoluble complexes, like complicated coacervates conjointly offer associate degree element barrier. For example, the complicated coacervates of soy macromolecule with chitosan created microcapsule for oil reduced the concentration of hexanal to a few times lesser than the management.[Citation120] Similar results were determined for the microcapsule created with oilseed macromolecule isolate-oilseed gum coacervates and WPI-GA coacervates.[Citation121,Citation122] In these cases, each compact surface layer and antioxidative biopolymers provided a robust protection result against PUFAs reaction. Also, polyphenols is applied to come up with noncovalent complexes. In vitro antioxidative activity of tannin-cuttlefish skin gelatin complicated at pH seven hierarchal between phenol acid and gelatin.[Citation123] However, the concentration of TBARS in animal oil emulsions stabilized with the complexes was reduced compared thereupon stabilized with tannin or gelatin throughout the storage. The complexes fashioned between gelatin and phenol acid-grape seed proanthocyanins were effective in preventing PUFAs from reaction.[Citation118] The polyphenols are prone to degradation and laborious to take up at the interface so it exhibits restricted protection of FAs in emulsions. The complexes facilitate to move polyphenols to the interface, leading to higher resistant of PUFAs against reaction. This provides a robust strategy to regulate FAs reaction in emulsions proving advantageous. Likewise, the wheat gliadin–dextran MRPs were applied to fabricate complexes of wheat gliadin–dextran with resveratrol and decelerated lipoid reaction in emulsion.[Citation124] Also, the resveratrol dropped at the interface through sorption of MRPs showed higher protection of PUFAs in emulsion than that through sorption of gliadin. Therefore, the noncovalent reaction between valency complexes and polyphenol is probably going to be sensible that suggests an increase in antioxidative activity because of their binding interaction.
We think that associating the structure formation with the thermodynamic measurement of the wall material layered with the active compound for the interaction between components could be of great importance, as it will give better information on the nature and the strength of interaction within the coating components, which can be used to prepare more stable microcapsules. The surface chemistry could also be used to tailor specific interactions between the coating material and the encapsulated species, which could be of great interest to achieve a better control of the load-release properties, compared to the few systems presented, which used a diffusion mechanism to encapsulate species (<change class=“ins iAUser” data=“377” data=“1” data=“Author” data=“1659012165967” id=“80” aid=“786153” user_name=“Author” title=“Inserted by Author - 07/28/2022 6:12 pm” sync=“syc”></change>).
Figure 5. Drug diffusion profile of multi-layered microencapsulation structure of antioxidants (AOX) and wall material (WM) with respect to time.
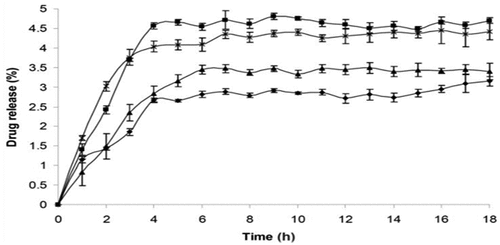
Authors Maria Jenita et al., 2020 evaluated the functional properties of co-microencapsulation of anthocyanins (polyphenol) extracted from spices and herbs, to evaluate their properties after storage for 90 days and exposed to heat. With almost any food-grade material considered as GRAS, using it as encapsulating material or as active compound, such as dextrans, gum arabic, persian gum, galactooligosaccharides, glucose, sucrose, lactose, maltodextrin, starch, vitamins, chitin, egg white, and mannitol. The challenge of co-microencapsulation is to find the synergy between active compounds and encapsulating materials for further optimization and to make the generation of such microcapsules more efficient to encapsulate the fatty acids, thus reducing operating costs. Where the results of storage shelf life subjected to physical and chemical instabilities were as follows: antioxidant activity showed no changes, remaining at 55.47 mMol Trolox/g, total anthocyanin content did not decrease, remaining at 20.76 mg C3G/g, the functional micronutrient content decreased from 10.30 × 10 × 110 CFU/g to 5.51 × 110 CFU/g.[Citation18] The authors report that the 2% sample showed a decrease in anthocyanin content and antioxidant activity, based on the rancimat oxidative stability records.
In co-microencapsulation, in order to find the ideal matrix to protect the active compounds such in the case of fatty acids, as well as to find and/or develop a synergy between the active compounds, it is necessary for the researcher to explore and evaluate the encapsulating materials and active compounds available to him, taking into account the accessibility, characteristics and cost of each one of them, selected based on the application.
Conclusions and future prospects
Emulsion-based delivery systems are promising to boost the aerophilous stability for PUFAs and MUFAs. Emulsion structure as well as the consistence of liquid section similarly because the thickness and therefore the charge of oil/water interface has been centered to enhance the aerophilous stability of PUFAs and MUFAs. Completely different emulsion varieties with numerous structures are associated with the aerophilous stability. Compared with the traditional emulsions, a thick surface layer in multi-layer emulsions acts as a chemical science barrier, whereas high consistence of continuous introduce gelled emulsions hinders the transfer development. On the opposite hand, emulsion structure style is additionally achieved by modifying surface layers by making ready valency and/or noncovalent complexes as emulsifiers. The usage of complexes ends up in the formation of dense surface layers that will conjointly exhibit smart antioxidative capability. Therefore, among completely different approaches to organize conjugate complexes, protein cross-linking is a lot of economical for modifying surface layers as a result of it ends up in the conjugates that not solely will type denser surface layers, however even have the exaggerated quantity of phenoplast chemical group, that provides higher protection for lipoid reaction. Additionally, stabilized emulsions with macromolecule fibrils are a possible approach to boost the reaction resistance of PUFAs and MUFAs. The multi-layered emulsion-based systems are well tried to enhance the aerophilous stability of PUFAs and MUFAs unlike in polymeric microcapsule and nano based. The generation of co-microencapsulation is cost-effective and distribution are multifactorial. Thus, a co-microencapsulation is deemed a promising multi-approach technique for enhancement of functional properties.
Disclosure statement
No potential conflict of interest was reported by the author(s).
References
- Berton-Carabin, C. C.; Ropers, M.-H.; Genot, C. Lipid Oxidation in oil-in-water Emulsions: Involvement of the Interfacial Layer. Compr. Rev. Food Sci. Food Saf 2015, 13(5), 945–977. DOI: 10.1111/1541-4337.12097.
- Lane, K. E.; Derbyshire, E. J. Omega-3 Fatty acids—A Review of Existing and Innovative Delivery Methods. Crit. Rev. Food Sci. Nutr 2018, 58(1), 62–69. DOI: 10.1080/10408398.2014.994699.
- Derbyshire, E. Brain Health across the Lifespan: A Systematic Review on the Role of Omega-3 Fatty Acid Supplements. Nutrients. 2018, 10(8), 1094. DOI: 10.3390/nu10081094.
- Elagizi, A; Lavie, C. J.; Marshall, K.; DiNicolantonio, J. J.; O’Keefe, J. H.; Milani, R. V. Omega-3 Polyunsaturated Fatty Acids and Cardiovascular Health: A Comprehensive Review. Prog. Cardiovasc. Diseases. 2018, 61(1), 76–85. DOI: 10.1016/j.pcad.2018.03.006.
- Laye, S.; Nadjar, A.; Joffre, C.; Bazinet, R. P. Anti- Inflammatory Effects of Omega-3 Fatty Acids in the Brain: Physiological Mechanisms and Relevance to Pharmacology. Pharmacol. Rev 2018, 70(1), 12–38. DOI: 10.1124/pr.117.014092.
- Rogero, M.; Calder, P. Obesity, Inflammation, toll-like Receptor 4 and Fatty Acids. Nutrients. 2018, 10(4), 432. DOI: 10.3390/nu10040432.
- D’Eliseo, D.; Velotti, F. Omega-3 Fatty Acids and Cancer Cell Cytotoxicity: Implications for multi-targeted Cancer Therapy. J. Clin. Med 2016, 5(2), 15. DOI: 10.3390/jcm5020015.
- Manson, J. E.; Cook, N. R.; Lee, I.-M.; Christen, W.; Bassuk, S. S.; Mora, S.; Copeland, T.; Albert, C. M.; Gordon, D.; Copeland, T. Marine N-3 Fatty Acids and Prevention of Cardiovascular Disease and Cancer. N. Engl. J. Med 2019, 380(1), 23–32. DOI: 10.1056/NEJMoa1811403.
- Miyashita, K.; Uemura, M.; Hosokawa, M. Effective Prevention of Oxidative Deterioration of Fish Oil: Focus on Flavor Deterioration. Ann. Rev. Food Sci. Technol 2018, 9(1), 209–226. DOI: 10.1146/annurev-food-030117-012320.
- Chen, X.; Zhang, Y.; Zu, Y.; Yang, L.; Lu, Q.; Wang, W. Antioxidant Effects of Rosemary Extracts on Sunflower Oil Com- Pared with Synthetic Antioxidants. Int. J. Food Sci. Technol 2014, 49(2), 385–391. DOI: 10.1111/ijfs12311.
- Wang, D.; Fan, W.; Guan, Y.; Huang, H.; Yi, T.; Ji, J. Oxidative Stability of Sunflower Oil Flavored by Essential Oil from Corian- Drum Sativum L. during Accelerated Storage. LWT Food Sci. Technol 2018a, 98, 268–275. DOI: 10.1016/j.lwt.2018.08.055.
- Czerny, M.; Schieberle, P.; Schieberle, P.; Schieberle, P.; Schieberle, P.; Schieberle, P.; Schieberle, P.; Hernandez, N. M.; Schieberle, P. Re-investigation on Odour Thresholds of Key Food Aroma Compounds and Development of an Aroma Language Based on Odour Qualities of Defined Aqueous Odorant Solutions. Eur. Food Res. Technol 2008, 228(2), 265–273. DOI: 10.1007/s00217-008-0931-x.
- Mahmoud, M. A. A.; Thorsten, T.; Loos, H. M.; Maria, W.; Andrea, B. Odorants in Fish Feeds: A Potential Source of Malodors in Aquaculture. Front. Chem 2018, 6, 241. DOI: 10.3389/fchem.2018.00241.
- Wang, X.; Zhu, C.; Peng, T.; Zhang, W.; Zhang, J.; Hu, L.; Wu, C.; Pan, X.; Wu, C. Enhanced Stability of an Emulsion Enriched in Unsaturated Fatty Acids by Dual Natural Antioxidants Fortified in Both the Aqueous and Oil Phases. Food Hydrocolloids. 2018b, 82, 322–328. DOI: 10.1016/j.foodhyd.2018.02.012.
- Encina, C.; Vergara, C.; Giménez, B.; Oyarzún-Ampuero, F.; Robert, P. Conventional spray-drying and Future Trends for the Microencapsulation of Fish Oil. Trends Food Sci. Technol 2016, 56, 46–60. DOI: 10.1016/j.tifs.2016.07.014.
- Vishnu, K. V.; Chatterjee, N. S.; Ajeeshkumar, K. K.; Lekshmi, R. G. K.; Tejpal, C. S.; Mathew, S.; Ravishankar, C. N. Microencapsulation of Sardine Oil: Application of Vanillic Acid Grafted Chitosan as a bio-functional Wall Material. Carbohydr. Polym 2017, 174, 540–548. DOI: 10.1016/j.carbpol.2017.06.076.
- Yildiz, G.; Ding, J.; Gaur, S.; Andrade, J.; Engeseth, N. E.; Feng, H. Microencapsulation of Docosahexaenoic Acid (DHA) with Four Wall Materials Including Pea protein-modified Starch Complex. Int. J. Biol. Macromol 2018, 114, 935–941. DOI: 10.1016/j.ijbiomac.2018.03.175.
- Maria Jenita, P.; Sukumar, M.; Lalithapriya, U.; Renuka, V. Formulation of Stable Edible Oil by Incorporating Microencapsulated Natural Polyphenols. Indian Patent J ( 201941043744 A. 2020, 45.
- Tong, L. M.; Sasaki, S.; McClements, D. J.; Decker, E. A. Antioxidant Activity of Whey in a Salmon Oil Emulsion. J. Food Sci 2010, 65(8), 1325–1329. DOI: 10.1111/j.1365-2621.2000.tb10606.x.
- McClements, D. J.; Bai, L.; Chung, C. Recent Advances in the Utilization of Natural Emulsifiers to Form and Stabilize Emulsions. Ann. Rev. Food Sci. Technol 2017, 8(1), 205–236. DOI: 10.1146/annurev-food-030216-030154.
- McClements, D. J.; Decker, E. A. Lipid Oxidation in oil-in- Water Emulsions: Impact of Molecular Environment on Chemical Reactions in Heterogeneous Food Systems. J. Food Sci 2000, 65(8), 1270–1282. DOI: 10.1111/j.1365-2621.2000.tb10596.x.
- McClements, D. J.; Decker, E. A. Interfacial Antioxidants: A Review of Natural and Synthetic Emulsifiers and Coemulsifiers that Can Inhibit Lipid Oxidation. J. Agric. Food Chem 2017, 66(1), 20–35. DOI: 10.1021/acs.jafc.7b05066.
- Miyashita, K.; Nara, E.; Ota, T. Oxidative Stability of Polyunsaturated Fatty Acids in an Aqueous Solution. Biosci., Biotechnol., Biochem 1993, 57(10), 1638–1640. DOI: 10.1271/bbb.57.1638.
- Mezouari, S.; Eichner, K. Evaluation of the Stability of Blends of Sunflower and Rice Bran Oil. Eur. J. Lipid Sci. Technol 2007, 109(5), 531–535. DOI: 10.1002/ejlt.200600217.
- Szumałaa, P.; Wysocka, I. Effect of Gelation and Storage Conditions on the Oxidative Stability of Microemulsion and Nanoemulsion Delivery Systems. Eur. J. Pharm. Sci. 2018, 214, 17–25. DOI: 10.1016/j.ejps.2018.08.021.
- Charoen, R.; Jangchud, A.; Jangchud, K.; Harnsilawat, T.; Decker, E. A.; McClements, D. J. Influence of Interfacial Com- Position on Oxidative Stability of oil-in-water Emulsions Stabilized by Biopolymer Emulsifiers. Food Chem 2012, 131(4), 1340–1346. DOI: 10.1016/j.foodchem.2011.09.128.
- Cui, L.; Cho, H. T.; McClements, D. J.; Decker, E. A.; Park, Y. Effects of Salts on Oxidative Stability of Lipids in Tween-20 Stabilized oil-in-water Emulsions. Food Chem 2016, 197(Part B), 1130–1135. DOI: 10.1016/j.foodchem.2015.11.099.
- Cui, L.; Jing, F.; Sun, Y.; Zhu, Z.; Yi, J. The Prooxidant Activity of Salts on the Lipid Oxidation of lecithin-stabilized oil-in-water Emulsions. Food Chem 2018, 252, 28–32. DOI: 10.1016/j.foodchem.2018.01.094.
- Chen, B.; Panya, A.; McClements, D. J.; Decker, E. A. New Insights into the Role of Iron in the Promotion of Lipid Oxidation in Bulk Oils Containing Reverse Micelles. J. Agric. Food Chem. 2012, 60, 3524–3532. DOI:10.1021/jf300138h.
- Jacobsen, C. Enrichment of Foods with Omega-3 Fatty Acids: A Multidisciplinary Challenge. Ann. N.Y. Acad. Sci 2010, 1190(1), 141–150. DOI: 10.1111/j.1749-6632.2009.05263.x.
- Waraho, T.; McClements, D. J.; Decker, E. A. Impact of Free Fatty Acid Concentration and Structure on Lipid Oxidation in oil-in- Water Emulsions. Food Chem 2011a, 129(3), 854–859. DOI: 10.1016/j.foodchem.2011.05.034.
- Waraho, T.; McClements, D. J.; Decker, E. A. Mechanisms of Lipid Oxidation in Food Dispersions. Trends Food Sci. Technol 2011b, 22(1), 3–13. DOI: 10.1016/j.tifs.2010.11.003.
- Zhu, Z.; Zhao, C.; Yi, J.; Liu, N.; Cao, Y.; Decker, E. A.; McClements, D. J. Impact of Interfacial Composition on Lipid and Protein co-oxidation in oil-in-water Emulsions Containing Mixed Emulsifiers. J. Agric. Food Chem 2018, 66(17), 4425–4468. DOI: 10.1021/acs.jafc.8b00590.
- Guzey, D.; McClements, D. J. Formation, Stability and Properties of Multilayer Emulsions for Application in the Food Industry. Adv. Colloid Interface Sci 2006, 128–130, 227–248. DOI: 10.1016/j.cis.2006.11.021.
- Sato, A.; Moraes, K.; Cunha, R. Development of Gelled Emulsions with Improved Oxidative and pH Stability. Food Hydrocolloids. 2014, 34, 184–192. DOI: 10.1016/j.foodhyd.2012.10.016.
- Tavernier, I.; Wijaya, W.; Meeren, P. V. D.; Dewettinck, K.; Patel, A. R. Food-grade Particles for Emulsion Stabilization. Trends Food Sci. Technol 2016, 50, 159–174. DOI: 10.1016/j.tifs.2016.01.023.
- Bush, L.; Stevenson, L.; Lane, K. E. The Oxidative Stability of Omega-3 oil-in-water Nanoemulsion Systems Suitable for Functional Food Enrichment: A Systematic Review of the Literature. Crit. Rev. Food Sci. Nutr 2017, 59(6), 1154–1168. DOI: 10.1080/10408398.2017.1394268.
- Elias, R. J.; Kellerby, S. S.; Decker, E. A. Antioxidant Activity of Proteins and Peptides. Crit. Rev. Food Sci. Nutr 2008, 48(5), 430–441. DOI: 10.1080/10408390701425615.
- Faraji, H.; Mcclements, D. J.; Decker, E. A. Role of Continuous Phase Protein on the Oxidative Stability of Fish oil-in-water Emulsions. J. Agric Food Chemi 2004, 52(14), 4558–4564. DOI: 10.1021/jf035346i.
- Hwang, J. Y.; Ha, H. K.; Lee, M. R.; Kim, J. W.; Kim, H. J.; Lee, W. J. Physicochemical Property and Oxidative Stability of Whey Protein Concentrate Multiple nano-emulsion Containing Fish Oil. J. Food Sci 2017, 82(2), 437–444. DOI: 10.1111/1750-3841.13591.
- Shao, Y.; Tang, C. H. Characteristics and Oxidative Stability of Soy protein-stabilized oil-in-water Emulsions: Influence of Ionic Strength and Heat Pretreatment. Food Hydrocolloids. 2014, 37, 149–158. DOI: 10.1016/j.foodhyd.2013.10.030.
- Gu, L.; Ning, P.; Chang, C.; McClements, D. J.; Su, Y.; Yang, Y. Fabrication of surface-active Antioxidant Food Biopolymers: Conjugation of Catechin Polymers to Egg White Proteins. Food Biophys. 2017, 12, 198–210. DOI:10.1007/s11483-017-9476-5.
- Chen, F.; Liang, L.; Zhang, Z.; Deng, Z.; Decker, E. A.; McClements, D. J. Inhibition of Lipid Oxidation in nano-emulsions and Filled Microgels Fortified with Omega-3 Fatty Acids Using Casein as a Natural Antioxidant. Food Hydrocolloids. 2017, 63, 240–248. DOI: 10.1016/j.foodhyd.2016.09.001.
- Qiu, C.; Zhao, M.; Decker, E. A.; McClements, D. J. Influence of Protein Type on Oxidation and Digestibility of Fish Oil- in-water Emulsions: Gliadin, Caseinate, and Whey Protein. Food Chem 2015, 175, 249–257. DOI: 10.1016/j.foodchem.2014.11.112.
- Sugiarto, M.; Ye, A.; Singh, H. Characterisation of Bind- Ing of Iron to Sodium Caseinate and Whey Protein Isolate. Food Chem 2009, 114(3), 1007–1013. DOI: 10.1016/j.foodchem.2008.10.062.
- McClements, D. J.; Gumus, C. E. Natural Emulsifiers— Biosurfactants, Phospholipids, Biopolymers, and Colloidal Particles: Molecular and Physicochemical Basis of Functional Performance. Adv. Colloid Interface Sci 2016, 234, 3–26. DOI: 10.1016/j.cis.2016.03.002.
- Chen, B.; McClements, D. J.; Decker, E. A. Role of Continuous Phase Anionic Polysaccharides on the Oxidative Stability of Menhaden oil-in-water Emulsions. J. Agric. Food Chem 2010, 58(6), 3779–3784. DOI: 10.1021/jf9037166.
- Celus, M.; Kyomugasho, C.; Loey, A. M. V.; Grauwet, T.; Hendrickx, M. E. Influence of Pectin Structural Properties on Interactions with Divalent Cations and Its Associated Functionalities. Compr. Rev. Food Sci. 2018, 17, 1576–1594. DOI:10.1111/1541-4337.12394.
- Salvia-Trujillo, L.; Decker, E. A.; McClements, D. J. Influence of an Anionic Polysaccharide on the Physical and Oxidative Stability of Omega-3 Nanoemulsions: Antioxidant Effects of Algi- Nate. Food Hydrocolloids. 2016, 52, 690–698. DOI: 10.1016/j.foodhyd.
- Klinkesorn, U.; Sophanodora, P.; Chinachoti, P.; McClements, D. J.; Decker, E. A. Increasing the Oxidative Stability of Liquid and Dried Tuna oil-in-water Emulsions with Electrostatic layer-by-layer Deposition Technology. J. Agric. Food Chem 2005, 53(11), 4561–4566. DOI: 10.1021/jf0479158.
- Panya, A.; Laguerre, M.; Lecomte, J.; Villeneuve, P.; Weiss, J.; McClements, D. J.; Decker, E. A. Effects of Chitosan and Rosmarinate Esters on the Physical and Oxidative Stability of Liposomes. J. Agric. Food Chem 2010, 58(9), 5679–5684. DOI: 10.1021/jf100133b.
- Li, J.; Pedersen, J. N.; Anankanbil, S.; Guo, Z. Enhanced Fish oil-in-water Emulsions Enabled by Rapeseed Lecithins Obtained under Different Processing Conditions. Food Chem 2018, 264, 233–240. DOI: 10.1016/j.foodchem.2018.05.053.
- Pichot, R.; Watson, R. L.; Norton, I. T. Phospholipids at the Interface: Current Trends and Challenges. Int. J. Mol. Sci. 2013, 14, 11767–11794. DOI: 10.3390/ijms140611767.
- Choe, J.; Oh, B.; Choe, E. Effect of Soybean Lecithin on iron-catalyzed or chlorophyll-photosensitized Oxidation of Canola Oil Emulsion. J. Food Sci 2014, 79(11), C2203–C2208. DOI: 10.1111/1750-3841.12683.
- Fomuso, L. B.; Corredig, M.; Akoh, C. C. Effect of Emulsifier on Oxidation Properties of Fish oil-based Structured Lipid Emulsions. J. Agric. Food Chem 2002, 50(10), 2957–2961. DOI: 10.1021/jf011229g.
- Yoshida, K.; Terao, J.; Suzuki, T.; Takama, K. Inhibitory Effect of Phosphatidylserine on iron-dependent Lipid Peroxidation. Biochem. Biophys. Res. Commun. 1991, 179, 1077–1081. DOI:10.1016/0006-291X(91)91929-7.
- Pan, Y.; Tikekar, R. V.; Nitin, N. Effect of Antioxidant Properties of Lecithin Emulsifier on Oxidative Stability of Encapsulated Bioactive Compounds. Int. J. Pharmaceutics. 2013, 450(1–2), 129–137. DOI: 10.1016/j.ijpharm.2013.04.038.
- Hidalgo, F. J.; León, M. M.; Zamora, R. Antioxidative Activity of Amino Phospholipids and phospholipid/amino Acid Mixtures in Edible Oils as Determined by the Rancimat Method. J. Agric. Food Chem 2006, 54(15), 5461–5467. DOI: 10.1021/jf060848s.
- Julio, L. M.; Copado, C. N.; Diehl, B. W. K.; Ixtaina, V. Y.; Tomás, M. C. Chia Bilayer Emulsions with Modified Sunflower Lecithins and Chitosan as Delivery Systems of Omega-3 Fatty Acids. LWT. 2017, 89, 581–590. DOI: 10.1016/j.lwt.2017.11.044.
- Sivapratha, S.; Sarkar, P. Multiple Layers and Conjugate Materials for Food Emulsion Stabilization. Crit. Rev. Food Sci. Nutr 2016, 58(6), 877–892. DOI: 10.1080/10408398.2016.1227765.
- Kartal, C.; Unal, M. K.; Otles, S. Flaxseed oil-in-water Emulsions Stabilized by Multilayer Membranes: Oxidative Stability and the Effects of pH. J. Dispersion Sci. Technol 2016, 37(12), 1683–1691. DOI: 10.1080/01932691.2016.1141294.
- Silvestre, M. P. C.; Chaiyasit, W.; Brannan, R. G.; McClements, D. J.; Decker, E. A. Ability of Surfactant Headgroup Size to Alter Lipid and Antioxidant Oxidation in oil-in-water Emulsions. J. Agric. Food Chem 2000, 48(6), 2057–2061. DOI: 10.1021/jf991162l.
- Tamm, F.; Härter, C.; Brodkorb, A.; Drusch, S. Functional and Antioxidant Properties of Whey Protein hydrolysate/pectin Complexes in Emulsions and spray-dried Microcapsules. LWT. 2016, 73, 524–527. DOI: 10.1016/j.lwt.2016.06.053.
- Jiménezmartín, E.; Gharsallaoui, A.; Pérezpalacios, T.; Carrascal, J. R.; Rojas, T. A. Suitability of Using Monolayered and multi-layered Emulsions for Microencapsulation of ω-3 Fatty Acids by Spray Drying: Effect of Storage at Different Temperatures. Food Bioprocess. Technol 2015, 8(1), 100–111. DOI: 10.1007/s11947-014-1382-y.
- Jo, Y. J.; Chun, J. Y.; Kwon, Y. J.; Min, S. G.; Choi, M. J. Formulation Development of multi-layered Fish Oil Emulsion by Using Electrostatic Deposition of Charged Biopolymers. Int. J. Food Eng 2015, 11(1), 31–39. DOI: 10.1515/ijfe-2014-0177.
- Gudipati, V.; Sandra, S.; McClements, D. J.; Decker, E. A. Oxidative Stability and in Vitro Digestibility of Fish oil-in-water Emulsions Containing Multilayered Membranes. J. Agric. Food Chem 2010, 58(13), 8093–8099. DOI: 10.1021/jf101348c.
- Farjami, T.; Madadlou, A. Fabrication Methods of Biopolymeric Microgels and microgel-based Hydrogels. Food Hydrocolloids. 2017, 62, 262–272. DOI: 10.1016/j.foodhyd.2016.08.017.
- Lu, Y.; Mao, L.; Hou, Z.; Miao, S.; Gao, Y. Development of Emulsion Gels for the Delivery of Functional Food Ingredients: From Structure to Functionality. Food Eng. Rev 2019, 11(4), 245–258. DOI: 10.1007/s12393-019-09194-z.
- Sun, C.; Gunasekaran, S.; Richards, M. P. Effect of Xanthan Gum on Physicochemical Properties of Whey Protein Isolate Stabilized oil-in-water Emulsions. Food Hydrocolloids. 2007, 21(4), 555–564. DOI: 10.1016/j.foodhyd.2006.06.003.
- Zhang, Z.; Decker, E. A.; McClements, D. J. Encapsulation, Protection, and Release of Polyunsaturated Lipids Using biopolymer-based Hydrogel Particles. Food Res. Int 2014, 64, 520–526. DOI: 10.1016/j.foodres.2014.07.020.
- Sato, A. C. K.; Polastro, M. Z.; Furtado, G. D. F.; Cunha, R. L. Gelled double-layered Emulsions for Protection of Flaxseed Oil. Food Biophys 2018, 13(3), 316–323. DOI: 10.1007/s11483-018-9537-4.
- Gallaher, J. J.; Hollender, R.; Peterson, D. G.; Roberts, R. F.; Coup- Land, J. N. Effect of Composition and Antioxidants on the Oxidative Stability of Fluid Milk Supplemented with an Algae Oil Emulsion. Int. Dairy J 2005, 15(4), 333–341. DOI: 10.1016/j.idairyj.2004.08.010.
- Pickering, S. U. CXCVI.— Emulsions. J. Chem. Soc., Trans. 1907, 91, 2001–2021. DOI: 10.1039/CT9079102001.
- Kargar, M.; Spyropoulos, F.; Norton, I. T. The Effect of Inter- Facial Microstructure on the Lipid Oxidation Stability of oil-in-water Emulsions. J. Colloid Interface Sci 2011, 357(2), 527–533. DOI: 10.1016/j.jcis.2011.02.019.
- RakotoarisoaMiora Rakotoarisoa, M.; Borislav, A.; Kargar, M.; Fayazmanesh, K.; Alavi, M.; Spyropoulos, F.; Norton, I. Composition-Switchable Liquid Crystalline Nanostructures as Green Formulations of Curcumin and Fish Oil. Am. Chem.l Society. 2021, 9(44), 14821–14835. DOI: 10.1021/acssuschemeng.1c04706.
- Okubanjo, S. S.; Loveday, S. M.; Ye, A.; Wilde, P. J.; Singh, H. Droplet-stabilized oil-in-water Emulsions Protect Unsaturated Lipids from Oxidation. J. Agric. Food Chem 2019, 67(9), 2626–2636. DOI: 10.1021/acs.jafc.8b02871.
- Pan, Y.; Tikekar, R. V.; Wang, M. S.; Avena-Bustillos, R. J.; Nitin, N. Effect of Barrier Properties of Zein Colloidal Particles and oil-in-water Emulsions on Oxidative Stability of Encapsulated Bioactive Compounds. Food Hydrocolloids. 2015, 43, 82–90. DOI: 10.1016/j.foodhyd.2014.05.002.
- Li, M. F.; He, Z. Y.; Li, G. Y.; Zeng, Q. Z.; Su, D. X.; Zhang, J. L.; He, S.; Yuan, Y.; He, S. The Formation and Characterization of Antioxidant Pickering Emulsions: Effect of the Interactions between Gliadin and Chitosan. Food Hydrocolloids. 2019, 90, 482–489. DOI: 10.1016/j.foodhyd.2018.12.052.
- Zhou, F. Z.; Yan, L.; Yin, S. W.; Tang, C. H.; Yang, X. Q. Development of Pickering Emulsions Stabilized by Gliadin/ Proanthocyanidins Hybrid Particles (Gphps) and the Fate of Lipid Oxidation and Digestion. J. Agric. Food Chem 2018, 66(6), 1461–1471. DOI: 10.1021/acs.jafc.7b05261.
- Zeng, T.; Wu, Z. L.; Zhu, J. Y.; Yin, S. W.; Tang, C. H.; Wu, L. Y.; Yang, X. Q. Development of Antioxidant Pickering High Internal Phase Emulsions (Hipes) Stabilized by protein/polysaccharide Hybrid Particles as Potential Alternative for PHOs. Food Chem 2017, 231, 122–130. DOI: 10.1016/j.foodchem.2017.03.116.
- Zhao, Y.; Guan, Y.; Pan, Y.; Nitin, N.; Tikekar, R. V. Improved Oxidative Barrier Properties of Emulsions Stabilized by silica–polymer Microparticles for Enhanced Stability of Encapsulants. Food Res. Int 2015, 74, 269–274. DOI: 10.1016/j.foodres.2015.05.008.
- Scheffler, S. L.; Wang, X.; Huang, L.; San-Martin Gonzalez, F.; Yao, Y. Phytoglycogen Octenyl Succinate, an Amphiphilic Carbo- Hydrate Nanoparticle, and ε-polylysine to Improve Lipid Oxidative Stability of Emulsions. J. Agric. Food Chem 2010, 58(1), 660–667. DOI: 10.1021/jf903170b.
- Liu, F.; Ma, C.; Gao, Y.; McClements, D. J. Food-grade Covalent Complexes and Their Application as Nutraceutical Delivery Systems: A Review. Compr. Rev. Food Sci. Food Saf 2016, 16(1), 76–95. DOI: 10.1111/1541-4337.12229.
- Gu, F. L.; Kim, J. M.; Abbas, S.; Zhang, X. M.; Xia, S. Q.; Chen, Z. X. Structure and Antioxidant Activity of High Molecular Weight Maillard Reaction Products from casein-glucose. Food Chem 2010, 120(2), 505–511. DOI: 10.1016/j.foodchem.2009.10.044.
- Jiang, Z.; Brodkorb, A. Structure and Antioxidant Activity of Maillard Reaction Products from α-lactalbumin and β- Lactoglobulin with Ribose in an Aqueous Model System. Food Chem 2012, 133(3), 960–968. DOI: 10.1016/j.foodchem.2012.02.016.
- Shi, Y.; Liang, R.; Chen, L.; Liu, H.; Goff, H. D.; Ma, J.; Zhong, F. The Antioxidant Mechanism of Maillard Reaction Products in oil-in-water Emulsion System. Food Hydrocolloids. 2019, 87, 582–592. DOI: 10.1016/j.foodhyd.2018.08.039.
- Vhangani, L. N.; Van, W. J. Antioxidant Activity of Maillard Reaction Products (Mrps) in a lipid-rich Model System. Food Chem 2016, 208, 301–308. DOI: 10.1016/j.foodchem.2016.03.100.
- Dong, S.; Panya, A.; Zeng, M.; Chen, B.; McClements, D. J.; Decker, E. A. Characteristics and Antioxidant Activity of Hydrolyzed β-lactoglobulin-glucose Maillard Reaction Products. Food Res. Int 2013, 46(1), 55–61. DOI: 10.1016/j.foodres.2011.11.022.
- Liu, J.; Liu, W.; Salt, L. J.; Ridout, M. J.; Ding, Y.; Wilde, P. J. Fish Oil Emulsions Stabilized with Caseinate Glycated by Dextran: Physicochemical Stability and Gastrointestinal Fate. J. Agric. Food Chem 2018, 67(1), 452–462. DOI: 10.1021/acs.jafc.8b04190.
- Wong, B. T.; Day, L.; Augustin, M. A. Deamidated Wheat protein–dextran Maillard Conjugates: Effect of Size and Location of Polysaccharide Conjugated on Steric Stabilization of Emulsions at Acidic pH. Food Hydrocolloids. 2011, 25(6), 1424–1432. DOI: 10.1016/j.foodhyd.2011.01.017.
- Zha, F.; Dong, S.; Rao, J.; Chen, B. Pea Protein Isolate- Gum Arabic Maillard Conjugates Improves Physical and Oxidative Stability of oil-in-water Emulsions. Food Chem 2019, 285, 130–138. DOI: 10.1016/j.foodchem.2019.01.151.
- Yang, Y.; Cui, S. W.; Gong, J.; Guo, Q.; Wang, Q.; Hua, Y. A Soy protein-polysaccharides Maillard Reaction Product Enhanced the Physical Stability of oil-in-water Emulsions Containing Citral. Food Hydrocolloids. 2015, 48, 155–164. DOI: 10.1016/j.foodhyd.2015.02.004.
- Corzo-Martínez, M.; Carrera-Sánchez, C.; Villamiel, M.; Rodríguez- Patino, J. M.; Moreno, F. J. Assessment of Interfacial and Foaming Properties of Bovine Sodium Caseinate Glycated with Galactose. J. Food Eng 2012, 113(3), 461–470. DOI: 10.1016/j.jfoodeng.2012.06.025.
- Tonon, R. V.; Grosso, C. R. F.; Hubinger, M. D. Influence of Emulsion Composition and Inlet Air Temperature on the Microencapsulation of Flaxseed Oil by Spray Drying. Food Res. Int 2011, 44(1), 282–289. DOI: 10.1016/j.foodres.2010.10.018.
- Zhang, Y.; Tan, C.; Abbas, S.; Eric, K.; Xia, S.; Zhang, X. Modified SPI Improves the Emulsion Properties and Oxidative Stability of Fish Oil Microcapsules. Food Hydrocolloids. 2015, 51, 108–117. DOI: 10.1016/j.foodhyd.2015.05.001.
- Curcio, M.; Puoci, F.; Iemma, F.; Parisi, O. I.; Cirillo, G.; Spizzirri, U. G.; Picci, N. Covalent Insertion of Antioxidant Molecules on Chitosan by a Free Radical Grafting Procedure. J. Agric. Food Chem 2009, 57(13), 5933–5938. DOI: 10.1021/jf900778u.
- Liu, J.; Pu, H.; Liu, S.; Kan, J.; Jin, C. Synthesis, Characterization, Bioactivity and Potential Application of Phenolic Acid Grafted Chitosan: A Review. Carbohydr. Polym 2017, 174, 999–1017. DOI: 10.1016/j.carbpol.2017.07.014.
- Fan, Y.; Liu, Y.; Gao, L.; Zhang, Y.; Yi, J. Oxidative Stability and in Vitro Digestion of Menhaden Oil Emulsions with Whey Protein: Effects of EGCG Conjugation and Interfacial cross-linking. Food Chem 2018, 265, 200–207. DOI: 10.1016/j.foodchem2018.05.098.
- Feng, J.; Cai, H.; Wang, H.; Li, C.; Liu, S. Improved Oxidative Stability of Fish Oil Emulsion by Grafted ovalbumin-catechin Conjugates. Food Chem 2017, 241, 60–69. DOI: 10.1016/j.foodchem.2017.08.055.
- Benjamin, Z.; Lutz, F.; Jochen, W. Stabilization of Food Dispersions by Enzymes. Food Funct 2014, 5(2), 198–213. DOI: 10.1039/C3FO60499C.
- Kellerby, S. S.; Yeun Suk, G.; McClements, D. J.; Decker, E. A. Lipid Oxidation in a Menhaden oil-in-water Emulsion Stabilized by Sodium Caseinate cross-linked with Transglutaminase. J. Agric. Food Chem 2006, 54(26), 10222–10227. DOI: 10.1021/jf062143w.
- Ma, H.; Forssell, P.; Kylli, P.; Lampi, A. M.; Buchert, J.; Boer, H.; Partanen, R. Transglutaminase Catalyzed cross-linking of Sodium Caseinate Improves Oxidative Stability of Flaxseed Oil Emulsion. J. Agric. Food Chem 2012, 60(24), 6223–6229. DOI: 10.1021/jf301166j.
- Liu, C.; Damodaran, S.; Heinonen, M. Effects of Microbial Transglutaminase Treatment on Physiochemical Properties and Emulsifying Functionality of Faba Bean Protein Isolate. LWT. 2019, 99, 396–403. DOI: 10.1016/j.lwt.2018.10.003.
- Phoon, P. Y.; Paul, L. N.; Burgner, J. W., II; Martin-Gonzalez, M. F. S.; Narsimhan, G. Effect of cross-linking of Interfacial Sodium Caseinate by Natural Processing on the Oxidative Stability of oil-in-water (O/W) Emulsions. J. Agric. Food Chem 2014, 62(13), 2822–2929. DOI: 10.1021/jf403285z.
- Kudanga, T.; Nyanhongo, G. S.; Guebitz, G. M.; Burton, S. Potential Applications of laccase-mediated Coupling and Grafting Reactions: A Review. Enzyme Microb. Technol. 2011, 48, 195–208. DOI:10.1016/j.enzmictec.2010.11.007.
- Minussi, R. C.; Pastore, G. M.; Durán, N. Potential Applications of Laccase in the Food Industry. Trends Food Sci. Technol 2002, 13(6–7), 205–216. DOI: 10.1016/S0924-2244(02)00155-3.
- Kurisawa, M.; Chung, J. E.; Uyama, H.; Kobayashi, S. Laccase-catalyzed Synthesis and Antioxidant Property of Poly(catechin). Macromol. Biosci 2003, 3(12), 758–764. DOI: 10.1002/mabi.200300038.
- Chung, J. E.; Kurisawa, M.; Uyama, H.; Kobayashi, S. Enzymatic Synthesis and Antioxidant Property of gelatin-catechin Conjugates. Biotechnol. Lett 2003, 25(23), 1993–1997. DOI: 10.1023/B:BILE.0000004391.27564.8e.
- Jia, X.; Zhao, M.; Jia, C.; Teng, J.; Wei, B.; Huang, L.; Xia, N. Emulsifying Properties and Antioxidant Stability of Enzymatically cross-linked Products between Rice Protein and Ferulic Acid. Food Sci 2017, 38(13), 131–137. DOI: 10.7506/spkx1002-6630-201713022.
- Johnston, S. P.; Nickerson, M. T.; Low, N. H. The Physicochemical Properties of Legume Protein Isolates and Their Ability to Stabilize oil-in-water Emulsions with and without Genipin. J. Food Sci. Technol 2015, 52(7), 4135–4145. DOI: 10.1007/s13197-014-1523-3.
- Gharsallaoui, A.; Roudant, G.; Beney, L.; Chambin, O.; Voilley, A.; Saurel,; Saurel, R. Properties of spray-dried Food Flavours Microencapsulated with Two- Layered Membranes: Role of Interfacial Interactions and Water. Food Chem 2011, 132(4), 1713–1720. DOI: 10.1016/j.foodchem.2011.03.028.
- Musin, E. V.; Kim, A. L.; Dubrovskii, A. V.; Tikhonenko, S. A. New Sight at the Organization of Layers of Multilayer Polyelectrolyte Microcapsules. Sci. Rep 2021, 11(1), 14040. DOI: 10.1038/s41598-021-93565-2.
- Tatiya, P. D.; Hedaoo, R. K.; Mahulikar, P. P.; Gite, V. V. Novel Polyurea Microcapsules Using Dendritic Functional Monomer: Synthesis, Characterization, and Its Use in Self-healing and Anticorrosive Polyurethane Coatings. Am. Chem.l Society. 2013, 52(4), 1562–1570. DOI: 10.1021/ie301813a.
- Angelova Orcid, A.; Drechsler, M.; Garamus, V. M. Liquid Crystalline Nanostructures as PEGylated Reservoirs of Omega-3 Polyunsaturated Fatty Acids: Structural Insights toward Delivery Formulations against Neurodegenerative Disorders. Am. Chem.l Society. 2018, 3(3), 3235–3247. DOI: 10.1021/acsomega.7b01935.
- Lee, S.-H.; Lefèvre, T.; Subirade, M.; Paquin, P. Changes and Roles of Secondary Structures of Whey Protein for the Formation of Protein Membrane at Soy oil/water Interface under High- Pressure Homogenization. J. Agric. Food Chem 2007, 55(26), 10924–10931. DOI: 10.1021/jf0726076.
- Lee, S. H.; Lefèvre, T.; Subirade, M.; Paquin, P. Effects of ultra-high-pressure Homogenization on the Properties and Structure of Interfacial Protein Layer in Whey protein-stabilized Emulsion. Food Chem 2009, 113(1), 191–195. DOI: 10.1016/j.foodchem.2008.07.067.
- Van der Plancken, I.; Loey, A. V.; Hendrickx, M. E. G. Changes in Sulfhydryl Content of Egg White Proteins Due to Heat and Pressure Treatment. J. Agric. Food Chem 2005, 53(14), 5726–5733. DOI: 10.1021/jf050289.
- Huang, Y.; Li, A.; Qiu, C.; Teng, Y.; Wang, Y. Self-assembled Colloidal Complexes of polyphenol-gelatin and Their Stabilizing Effects on Emulsions. Food Funct 2017, 8(9), 3145. DOI: 10.1039/C7FO00705A.
- Yao, X.; Xiang, S.; Nie, K.; Gao, Z.; Zhang, W.; Fang, Y.; Jiang, F.; Phillips, G. O.; Jiang, F. Whey Protein isolate/gum Arabic Intramolecular Soluble Complexes Improving the Physical and Oxidative Stabilities of Con- Jugated Linoleic Acid Emulsions. RSC Adv 2016, 6(18), 14635–14642. DOI: 10.1039/C5RA26040J.
- Yuan, Y.; Kong, Z.-Y.; Sun, Y.-E.; Zeng, Q.-Z.; Yang, X.-Q. Complex Coacervation of Soy Protein with Chitosan: Constructing Antioxidant Microcapsule for Algal Oil Delivery. LWT. 2017, 75, 171–179. DOI: 10.1016/j.lwt.2016.08.045.
- Eratte, D.; Wang, B.; Dowling, K.; Barrow, C. J.; Adhikari, B. P. Complex Coacervation with Whey Protein Isolate and Gum Arabic for the Microencapsulation of Omega-3 Rich Tuna Oil. Food Funct. 2014, 5, 2743–2750. DOI:10.1039/C4FO00296B.
- Kaushik, P.; Dowling, K.; McKnight, S.; Barrow, C. J.; Adhikari, B. Microencapsulation of Flaxseed Oil in Flaxseed Protein and Flaxseed Gum Complex Coacervates. Food Res. Int 2016, 86, 1683–1691. DOI: 10.1016/j.foodres.2016.05.015.
- Aewsiri, T.; Benjakul, S.; Visessanguan, W.; Wierenga, P. A.; Grup- Pen, H. Antioxidative Activity and Emulsifying Properties of Cuttlefish Skin gelatin-tannic Acid Complex as Influenced by Types of Interaction. Innovative Food Sci. Emerging Technol 2010, 11(4), 712–720. DOI: 10.1016/j.ifset.2010.04.001.
- Qiu, C.; Wang, B.; Yong, W.; Teng, Y. Effects of Colloidal Complexes Formation between Resveratrol and Deamidated Gliadin on the Bioaccessibility and Lipid Oxidative Stability. Food Hydrocoll. 2017, 69, 466–472. DOI: 10.1016/j.foodhyd.2017.02.020.